Abstract
The determination of the collection efficiency (CE) of particles during transport, vaporization, and ionization in the aerosol mass spectrometer (AMS), which uses vaporizer to evaporate non-refractory particles with subsequent ionization, is important for accurately quantifying the concentrations of chemical constituents. Particle bounce in the vaporizer can be considered as one of the most important parameters influencing the CE of particles. Substrates with various shapes (flat, cylindrical, reverse-conical, cup, trapezoidal, and reverse-T), materials (stainless steel, copper, tungsten, and molybdenum), pores with average sizes of 0.2, 1, 5, 20, and 100 μm, and mesh with a size of 79 μm, which can be a possible candidate for the vaporizer in the AMS, were constructed. Bounce fractions of sub-micrometer particles (polystyrene latex, oleic acid, and dioctyl phthalate) were determined using the differential mobility analyzer (DMA)-impactor technique under a constant impact velocity. For the porous substrate, the particle bounce fraction significantly decreased with increasing pore size and porosity, but there was an upper limit for the pore size above which the particle bounce fraction no longer decreased significantly (i.e., the rebounded particles successfully escaped from the pores). The mesh substrate also had a lower particle bounce fraction than the flat substrate. Among the tested materials, the copper substrate having the lowest hardness and elasticity had the lowest particle bounce fraction. In addition, the reverse-T shape substrate having more available surfaces for particle entrapment led to the reduction of particle bounce fraction. In terms of phase, the liquid particles had lower particle bounce fractions than the solid particles. Our results suggest that the vaporizer in the AMS should provide traps for multiple collisions of the rebounding particles with an appropriate porosity or mesh and should be made of low-hardness materials to minimize particle bounce.
Copyright 2015 American Association for Aerosol Research
INTRODUCTION
A real-time determination of the chemical constituents of aerosols is essential in determining their source, formation pathway, and aging process, as well as better understanding their effects on climate change and human health. The aerosol mass spectrometry technique has been used as a powerful tool to determine the chemical constituents of sub-micrometer atmospheric aerosols in real time (Suess and Prather Citation1999; Jayne et al. Citation2000; McMurry Citation2000; Noble and Prather Citation2000; Allan et al. Citation2003; Jimenez et al. Citation2003; Middlebrook et al. Citation2003; Dall’Osto et al. Citation2004; Drewnick et al. Citation2004; Topping et al. Citation2004; Takami et al. Citation2005; Takegawa et al. Citation2005; Nash et al. Citation2006; Canagaratna et al. Citation2007; DeCarlo et al. Citation2007; Liu et al. Citation2007; Kamphus et al. Citation2008). One of the popular methods of aerosol mass spectrometry instruments is to combine thermal vaporization and electron impact ionization (EI) with subsequent mass analysis of the produced ions by mass spectrometer. For example, the Aerodyne aerosol mass spectrometry (AMS) (Aerodyne Inc., USA; hereafter, the Aerodyne AMS is written as AMS) is able to provide the size-resolved chemical composition (e.g., organics, sulfate, nitrate, ammonium, and chloride) of non-refractory sub-micrometer particles in several minutes, and the AMS mass spectra can also be used to provide useful information on organic types (Paatero and Tapper Citation1994; Paatero Citation1997; Zhang et al. Citation2005; Lanz et al. Citation2007; Ulbrich et al. Citation2008; Ng et al. Citation2011; Zhang et al. Citation2011).
Although the AMS has been successfully applied as a quantitative tool for the determination of chemical constituents of non-refractory sub-micrometer particles, questions remain concerning the collection efficiency (CE) of particles, which is essential to accurately quantify the mass concentration of chemical constituents. The CE is defined as the ratio of the mass concentration of particles measured with the AMS to the concentration measured before entering into the AMS. The CE is affected by particle transmission into the vacuum, transport of particles onto the vaporizer, particle bounce in the vaporizer, and particle vaporization efficiency (Huffmann et al. Citation2005; Matthew et al. Citation2008). Several laboratory and field tests reported that a CE of less than 100% was mainly caused by particle bounce from the vaporizer, especially for solid particles (Jayne et al. Citation2000; Huffman et al. Citation2005; Quinn et al. Citation2006; Liu et al. Citation2007; Salcedo et al. Citation2007). In addition, particle bounce has been considered as an important issue for impactor techniques that use the inertial force of particles (i.e., high impaction velocity) for sampling or separation (Virtanen et al. Citation2010; Bateman et al. Citation2014).
Physical properties of particles, such as hygroscopicity, size, density, phase, elasticity, and hardness, play an important role in particle bounce behavior (Wall et al. Citation1990; Stein et al. Citation1994; Matthew et al. Citation2008; Virtanen et al. Citation2010; Virtanen et al. Citation2011; Bateman et al. Citation2014). Hygroscopic particles, such as salts, are known to have much less bounce than non-hygroscopic particles (Stein et al. Citation1994). Virtanen et al. (Citation2011) found that freshly nucleated biogenic particles having a lower oxidation degree or containing a greater number of sulfates (i.e., liquid phase) bounced less than the aged particles (solid phase). They also utilized the particle bounce behavior to estimate the physical phase of secondary organic aerosols (SOA). Matthew et al. (Citation2008) reported that liquid and liquid-coated particles were collected with an efficiency of nearly 100%, while the CE of solid particles was as low as 20%. The low CE was for the most part caused by particle bounce. The CE of the internally mixed particles fell between these values. They found that the CE of internally mixed particles was independent of their chemical species, and the same CE can be used for the internally mixed particles. In case of the CE of the AMS, a 50% CE was assumed to obtain consistent results with collocated measurements at urban area (Canagaratna et al. Citation2007). Docherty et al. (2013) reported that the CE of chamber-generated SOA varied from 100 to <15% depending on the composition of organic fraction (i.e., the extent of oxidation of organics). Recently, Takegawa et al. (Citation2013) suggested that the anisotropy of elastic properties and the brittleness of solid particles should also play an important role in controlling their adhesion and bounce. In general, particles of smaller size and low hardness were known to have less probability of particle bounce (Dahneket Citation1971; Hinds Citation1999).
In addition to the physical properties of particles, the velocity of particles, surrounding relative humidity (RH), and collection substrate properties can affect particle bounce. The impact velocity of particles in the AMS under high vacuum is known to be approximately 80–200 m/s, and the higher impact velocity (i.e., higher kinetic energy) can cause the higher bounce (Jayne et al. Citation2000). The slower impaction velocity cannot be achieved in the AMS due to high vacuum pressure system. Bateman et al. (Citation2014) examined the effect of RH on particle bounce, and found that particle bounce was strongly dependent on the surrounding RH, even for non-hygroscopic particles, due to increased adhesion energy by capillary force. However, the RH inside the AMS should be low. The coating of substrate with oily materials can reduce particle bounce, but this is not applicable for the AMS vaporizer or impactor substrates for chemical measurements due to the contamination problem of oily materials. Modification of the substrate (i.e., vaporizer in the AMS) is one possible solution to reduce particle bounce, leading to an increase in CE in the AMS. The box type of vaporizer can be used to cause multiple hits of rebound particles (i.e., secondary impacts) to increase CE (Chang et al. Citation1999). However, the performance of substrates with varying materials, shapes, and surface properties (flat, porous, and mesh surface) has not been fully assessed.
In this study, the effects of collection substrate properties on particle bounce were examined. The DMA-impactor method (Stein et al. Citation1994; Bateman et al. Citation2014) was applied to evaluate particle bounce behavior under a constant impact velocity (∼104 m/s) and a dried condition (RH <20%), which is similar to the condition whereby particle bounce can occur in the AMS vaporizer. Collection substrates (i.e., vaporizers) of various materials, geometry, porosity, and mesh were constructed and tested by using solid and liquid particles. Sub-micrometer polystyrene latex (PSL) and sodium chloride (NaCl) particles were used for the solid ones, while dioctyl phthalate (DOP) and oleic acid particles were used for the liquid ones. Our data will be useful to examine the effect of substrate types on particle bounce and will elucidate the selection of vaporizer for more accurate quantification of chemical constituents in the AMS.
EXPERIMENTAL
A schematic of the DMA-impactor method is displayed in . A constant output atomizer (TSI 3075, USA) is used to produce particles from PSL (Duke Scientific, USA, Sigma-Aldrich, USA), sodium chloride (99.4% purity, Sigma, USA), oleic acid (90% purity, Aldrich, USA), or DOP (99% purity, Aldrich, USA) in deionized (DI) water solutions. The PSL and sodium chloride particles were used as a proxy for solid particles, while the DOP and oleic acid particles were used as liquid particles to evaluate the effect of particle phase on particle bounce. They were dried out by a series of diffusion driers and passed through neutralizer (TSI 3077, USA) before being introduced into the DMA (TSI 3989, USA). The DMA with aerosol and sheath flow rates of 1 and 5 liters per minute (Lpm), respectively, selected particles of a certain mobility-equivalent size. The PSL particles that were already monodisperse were also introduced in the DMA to select particles of a given size excluding small residual particles that can exist in DI water. The mobility-equivalent sizes of tested particles were converted to the aerodynamic-equivalent sizes by using density values (1.05, 2.20, 0.98, and 0.89 g/cm3 for PSL, sodium chloride, DOP, and oleic acid, respectively) (Hinds Citation1999). All particles were assumed to be spherical except for the sodium chloride particles with a cubic shape (dynamic shape factor = 1.07) (Hinds Citation1999). Hereafter, the aerodynamic-equivalent diameter was used as particle size in this study. The selected particles were introduced into a single stage of the impactor having a theoretical cutoff size of ∼230 nm for the particles with unit density (1 g/cm3) at a flow rate of 10 Lpm (Lee Citation2004). The calculated aerosol velocity is ∼104 m/s, and the distance between the end of nozzle (a nozzle diameter of 0.58 mm) and the collecting surface was 1 mm with S/W (jet-to-plate distance/jet width) and T/W (nozzle throat length/jet width) (Marple and Willeke Citation1976) values of 1.72 and 10.34, respectively. The pressure drop through the impactor was around 10 cm H2O. The low vacuum pressure with long particle trajectory in the AMS could not be simulated in the current experimental setup. To account for the effects of particle wall losses or other losses in the particle bounce fraction, three types of impactor setups were used (Bateman et al. Citation2014) as displayed in (i.e., impactors with no substrate, grease-coated substrate, and uncoated substrate). A Dow Corning 976V high vacuum grease (Dow Corning, USA) was used to coat various substrates tested here. The thickness of grease was estimated to be less than 0.05 mm. The grease-coated substrate removes particle bounce. Number of concentrations downstream of each impactor (impactors with no substrate [N1], grease-coated substrate [N2], and uncoated substrate [N3]) were measured with one condensation particle counter (CPC) (3772 TSI, USA). The input concentration was monitored for ensuring stable particle generation during the measurement, and each measurement and switching over to different substrate configuration was completed within 5–10 min. The particle bounce fraction was calculated by the number difference between the uncoated substrate and coated substrate (N3–N2) divided by the number difference between no substrate and coated substrate (N1–N2) (Bateman et al. Citation2014). The transmission efficiency of particles on the coated substrate and uncoated substrate was calculated by the ratio of N2 to N1, and the ratio of N3 to N1, respectively.
Particles larger than the cut-off size for impactor were used for the evaluation of particle bounce behavior. Humid conditions (i.e., high RH) have been shown to significantly reduce particle bounce (Stein et al. Citation1994; Bateman et al. Citation2014). The measured RH inside the impactor was 15–20% (i.e., dry condition) for all measurements that were conducted. The particle number concentration before entering the impactor was maintained at higher than ∼500 particles/cm3 to obtain reliable counting statistics. To prevent heavy particle loading on the surface of the collection surface (as the surface becomes loaded with particles, the incoming particles will strike on previously deposited particles) (Tsai and Cheng Citation1995), the particle bounce measurement was completed within 10 min. The frequent thermal desorption of the sample in the AMS would prevent particles from being heavily loaded on substrate.
Various collection substrates having different materials, shapes, pores, and mesh were constructed, as summarized in . Flat substrates made of stainless steel, copper, tungsten, and molybdenum were prepared to evaluate the effect of substrate materials on the particle bounce fraction. To assess the effect of porosity on the particle bounce fraction, stainless steel substrates having average pore sizes (porosity) of 0.2 μm (15%), 1 μm (25%), 5 μm (35%), 20 μm (45%), and 100 μm (55%) were constructed (Table S2 in the online supplementary information [SI]). In addition, a stainless steel substrate having a mesh size of 77 μm (∼35% opening area on the surface) was tested. Various shapes of substrates (cylindrical, reverse-conical, trapezoidal, cup, and reverse-T), as displayed in were constructed to observe the effect of shape on particle bounce.
TABLE 1 Various collection substrates having different materials, shapes, pores (pore size and porosity), and mesh
RESULTS AND DISCUSSION
The transmission efficiency of sodium chloride particles as a function of aerodynamic size through the impactor with a grease-coated substrate and an uncoated substrate (stainless steel flat substrate) is displayed in . The mobility-equivalent size of sodium chloride particles selected by the DMA was converted to the aerodynamic-equivalent size by using a density of 2.2 g/cm3 and a dynamic shape factor of 1.07. The general shape of the transmission efficiency curve is affected by the impactor type. It was reported that a higher steepness (i.e., a higher resolution) can be obtained with a narrower particle impaction velocity distribution (Bateman et al. Citation2014). The measured cut-off size was around 210 nm, which is close to the theoretical cut-off size of ∼186 nm for sodium chloride particles. The transmission efficiency of PSL particles as a function of aerodynamic size with grease-coated substrate, as displayed in b, has a similar shape of transmission curve as that obtained by using sodium chloride particles. As not shown here, transmission efficiency values for 283.7- and 472.8-nm oleic acid particles were 15% and 1%, respectively, and those of 297.4- and 495.7-nm DOP particles were 7% and 2%, respectively, which were smaller than the solid particles (i.e., the higher CE of liquid particles than solid particles).
FIG. 2. Transmission efficiency of sodium chloride particles as a function of aerodynamic size through the impactor with a grease-coated substrate and an uncoated substrate (stainless steel flat substrate) (the transmission curve of PSL particles with a grease-coated substrate was also included).
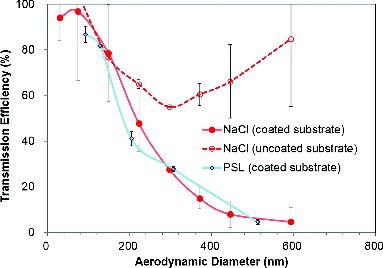
The particle bounce fractions of PSL particles with aerodynamic sizes of 307.4 nm and 512.4 nm for collection substrates made of stainless steel, copper, molybdenum, and tungsten are displayed in . The flat-shaped surface was used for all substrates. As indicated in , the copper substrate had the lowest particle bounce fraction (61.1% and 76.5% for the 307.4- and 512.4-nm particles, respectively). Even though the difference between copper, tungsten, and molybdenum is not large, the difference between stainless steel and other materials (91% versus 82%) is significant. The hardness (e.g., rebound hardness) and elasticity of copper is the lowest among tested materials (see Table S1 in the SI for hardness and elasticity of each material), possibly leading to the lowest particle bounce fraction (Miyakawa et al. Citation2013). Molybdenum and tungsten substrates also had the lower particle bounce fraction than the stainless steel substrate. Stainless steel, which had the highest particle bounce fraction (85% and 91% for the 307.4- and 512.4-nm particles, respectively), contains a film of chromium oxide (passive layer) to prevent corrosion by oxygen diffusion, providing more flat surface (i.e., less roughness) compared with other types of substrates. Note that when the substrate was coated with grease, the particle bounce fraction decreased significantly. However, the greased substrate cannot be used for the AMS vaporizer due to chemical interference of grease.
FIG. 3. Particle bounce fractions of PSL particles with aerodynamic sizes of 307.4 nm and 512.4 nm for flat substrates made of stainless steel, copper, tungsten, and molybdenum.
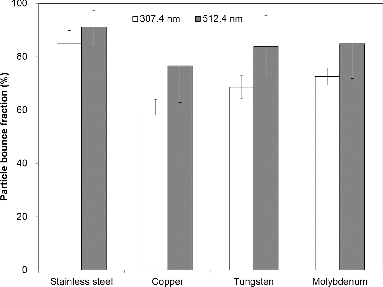
Porosity or pore size may be one of the important parameters affecting particle bounce fractions. Particles with high velocity that accelerate at the exit of the nozzle can be entrapped in the pores. displays the particle bounce fractions of PSL particles with aerodynamic sizes of 307.4 nm and 512.4 nm as a function of average pore sizes of 0.2, 1, 5, 20, and 100 μm, and porosity of 15, 25, 35, 45, and 55% (refer to ). The pores are randomly positioned on the substrate surface as well as inside the substrate. Substrate with the highest pore size has the highest porosity (=volume of pore/total volume) in the tested substrates. It was found that as the pore size or porosity increased, the particle bounce fraction significantly decreased. The existence of pores would lead to a higher probability of the impacted particles being captured inside the pore and/or the rebound particles being impacted again on other surfaces. However, for substrates having pore sizes larger than 20 μm, the decreasing trend of the particle bounce fraction with increasing pore size was not significantly observed. This might occur because the large pore space inside the substrate caused the rebounded particles to successfully escape (i.e., there was insufficient time for rebounded particles to have multiple collisions inside the pores due to their large space). Our data suggest that pore size or porosity should be sufficiently large for particles to be introduced into and captured by the pores, and that there is an upper limit for the pore size above which the particle bounce fraction no longer changed significantly.
FIG. 4. Particle bounce fractions of PSL particles with aerodynamic sizes of 307.4 nm and 512.4 nm for flat substrates with average pore sizes (porosity) of 0.2 μm (15%), 1 μm (25%), 5 μm (35%), 20 μm (45%), and 100 μm (55%), and mesh size of 74 μm (34% opening area).
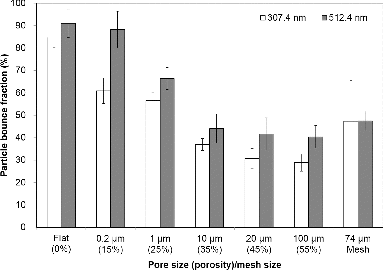
A mesh substrate was also constructed, as displayed in . The square mesh was regularly distributed on the surface of substrate, contrary to porous substrates (the porous substrate has randomly oriented pores on and inside the surface). The 200 mesh indicates that the number of mesh is 40,000 in the 1-inch × 1-inch surface, and the size of each mesh is calculated to be approximately 74 μm (∼34% opening area). It was also found that the mesh substrate led to the lower particle bounce fraction than that of the flat substrate as shown in . Takegawa et al. (Citation2012) also showed that the newly designed particle trap comprising mesh layers led to significant reduction in particle bounce.
Results for particle bounce fractions for the varying shapes of substrates (flat, cylindrical, reverse-conical, cup, trapezoidal, and reverse-T) (), which were made of stainless steel without pores, are displayed in . Compared with the stainless steel flat substrate (i.e., the worst case), substrates having more available surfaces and space for particle entrapment had the lower particle bounce fraction. The reverse-T shape of substrates had the lowest particle bounce fractions (40.4 and 65.0% for 307.4- and 512.4-nm particles, respectively) among the tested ones, although the difference between varying shapes (except the flat shape) was not very large. We speculated that more multiple striking of particles on additional surface and the higher possibility of entrapment in such substrates led to the lower particle bounce fraction. The particles lose their initial kinetic energy upon impaction, and on second impaction, the lower kinetic energy led to the higher probability of adhesion. Our data suggest that for the limited space in the AMS ionization regime, the vaporizer that provides traps for multiple collisions of rebounding particles with an appropriate porosity or mesh is desirable to minimize particle bounce.
FIG. 5. Particle bounce fractions of PSL particles for varying shapes of substrates (flat, cylindrical, reverse-conical, cup, trapezoidal, and reverse-T).
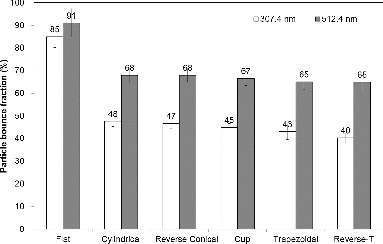
Effects of particle phase (i.e., particle properties) were examined by using PSL (solid), oleic acid (liquid), and DOP (liquid) particles on the flat stainless steel substrate, as displayed in . Liquid particles such as DOP and oleic acid would not bounce very much compared with solid particles (PSL). Thus, the effect of geometries on the CE of liquid particles should not be significant. Liquid particles typically had a lower elasticity than solid particles having little bounce (Matthew et al. Citation2008). Also, it is possible to have shattering of liquid particles after impaction. In terms of size, the larger particles tend to have a higher bounce fraction due to their higher incident kinetic energy throughout this study. Thus, size and phase-dependent CE would be required to accurately quantify chemical species in the AMS.
CONCLUSIONS
Particle bounce fractions for various collection substrates with shapes (flat, cylindrical, reverse-conical, cup, trapezoidal, and reverse-T), materials (stainless steel, copper, tungsten, and molybdenum), pore sizes (porosity) (0.2 μm [15%], 1 μm [25%], 5 μm [35%], 20 μm [45%], and 100 μm [55%]), and mesh (mesh size of 79 μm) were determined using the DMA-impactor technique. As the pore size and porosity increased to 10 μm (35% porosity), the particle bounce fraction significantly decreased to 37% and 44% from 85% and 91% (flat substrate without pores) for 307.4- and 512.4-nm particles, respectively. However, the decreasing behavior of the particle bounce fraction was not significant above the pore size of 20 μm. This occurred possibly because the rebounded particles successfully escaped due to insufficient multiple collisions inside the pores (i.e., large space in the pores). Substrates having mesh on the surface also led to lower bounce fraction compared with the flat substrate. The material types also affected the particle bounce fraction, although not to the same extent as the porous or mesh substrates. The copper substrate had the lowest particle bounce fraction (61.1 and 76.5% for 307.4- and 512.4-nm particles, respectively) among the tested ones due to the lowest hardness and elasticity of copper. Among the varying shapes of substrates, the reverse-T shape substrate had the lowest particle bounce fractions (40.4 and 65.0% for 307.4- and 512.4-nm PSL particles, respectively) due to the more efficient entrapment of incoming or rebounded particles. In conclusion, although no substrate materials, porosity, or shape was able to completely remove particle bounce, the non-flat substrate with appropriate porosity (not excessively high) or mesh made with low-hardness and low-elasticity materials could be recommended for AMS vaporizer with the minimal of particle bounce.
SUPPLEMENTAL MATERIAL
Supplemental data for this article can be accessed on the publisher's website.
UAST_A_1028518_Supplementary_Files.zip
Download Zip (11.9 KB)FUNDING
This research was supported by the National Leading Research Laboratory program (NRF-2011-0015548) and PM2.5 research consortium (NRF-2014M3C8A5028593) funded by the Ministry of Science, ICT, and Future Planning (MSIP) and the National Research Foundation (NRF) of Korea.
REFERENCES
- Allan, J. D., Alfarra, M. R., Bower, K. N., Williams, P. I., Gallagher, M. W., Jimenez, J. L., McDonald, A. G., Nemitz, E., Canagaratna, M. R., Jayne, J. T., Coe, H., and Worsnop, D. R. (2003). Quantitative Sampling using an Aerodyne Aerosol Mass Spectrometer 2. Measurements of Fine Particulate Chemical Composition in Two UK Cities. J. Geophys. Res. D 108:4091, doi:10.1029/2002JD002359, D3.
- Bateman, A. P., Belassein, H., and Martin, S. T. (2014). Impactor Apparatus for the Study of Particle Rebound: Relative Humidity and Capillary Forces. Aerosol Sci. Technol. 48:42–52.
- Canagaratna, M., Jayne, J., Jimenez, J., Allan, J., Alfarra, M., Zhang, Q., Onasch, T., Drewnick, F., Coe, H., and Middlebrook, A. (2007). Chemical and Microphysical Characterization of Ambient Aerosols with the Aerodyne Aerosol Mass Spectrometer. Mass Spectrom. Rev., 26:185–222.
- Chang, M., Kim, S., and Sioutas, C. (1999). Experimental Studies on Particle Impaction and Bounce: Effects of Substrate Design and Material. Atmos. Environ., 33(15):2313–2322.
- Dahneket, B. (1971). The Capture of Aerosol Particles by Surfaces. J. Colloid Interface Sci., 37(2):342–353.
- Dall’Osto, M., Beddows, D. C. S., Kinnersley, R. P., Harrison, R. M., Donovan, R. J., and Heal, M. R. (2004). Characterization of Individual Airborne Particles by using Aerosol Time-of-Flight Mass Spectrometry at Mace Head, Ireland. J. Geophys. Res. D, 109:D21302, doi:10.1029/2004JD004747.
- DeCarlo, P. F., Dunlea, E. J., Kimmel, J. R., Aiken, A. C., Sueper, D., Crounse, J., Wennberg, P. O., Emmons, L., Shinozuka, Y., Clarke, A., Zhou, J., Tomlinson, J., Collins, D. R., Knapp, D., Weinheimer, A. J., Montzka, D. D., Campos, T., and Jimenez, J. L. (2007). Fast Airborne Aerosol Size and Chemistry Measurements with the High Resolution Aerosol Mass Spectrometer During the MILAGRO Campaign. Atmos. Chem. Phys. Discuss., 7:18269–18317.
- Docherty, K. S., Jaoui, M., Corse, E., Jimenez, J. L., Offenberg, J. H., Lewandowski, M., and Kleindienst, T. E. (2013). Collection Efficiency of the Aerosol Mass Spectrometer for Chamber-Generated Secondary Organic Aerosols. Aerosol Sci. Technol., 47:294–309.
- Drewnick, F., Jayne, J. T., Canagaratna, M., Worsnop, D. R., and Demerjian, K. L. (2004). Measurement of Ambient Aerosol Composition During the PMTACS-NY 2001 Using an Aerosol Mass Spectrometer. Part II: Chemically Speciated Mass Distributions Special Issue of Aerosol Science and Technology on Findings from the Fine Particulate Matter Supersites Program. Aerosol Sci. Technol., 38:104–117.
- Hinds, C. W. (1999). Aerosol Technology: Properties, Behavior, and Measurement of Airborne Particles. 2nd ed. John Wiley, New York, NY.
- Huffman, J. A., Jayne, J. T., Drewnick, F., Aiken, A. C., Onasch, T., Worsnop, D. R., and Jimenez, J. L. (2005). Design, Modeling, Optimization, and Experimental Tests of a Particle Beam Width Probe for the Aerodyne Aerosol Mass Spectrometer. Aerosol Sci. Technol., 39:1143–1163.
- Jayne, J. T., Leard, D. C., Zhang, X., Davidovits, P., Smith, K. A., Kolb, C. E., and Worsnop, D. R. (2000). Development of an Aerosol Mass Spectrometer for Size and Composition Analysis of Submicron Particles. Aerosol Sci. Technol., 33:49–70.
- Jimenez, J. L., Jayne, J. T., Shi, Q., Kolb, C. E., Worsnop, D. R., Yourshaw, I., Seinfeld, J. H., Flagan, R. C., Zhang, X., Smith, K. A., Morris, J. W., and Davidovits, P. (2003). Ambient Aerosol Sampling Using the Aerodyne Aerosol Mass Spectrometer. J. Geophys. Res. D, 108:8425, doi:10.1029/2001JD001213, D7.
- Kamphus, M., Ettner-Mahl, M., Brands, M., Curtius, J., Drewnick, F., and Borrmann, S. (2008). Comparison of Two Aerodynamic Lenses as an Inlet for a Single Particle Laser Ablation Mass Spectrometer. Aerosol Sci. Technol., 42:970–980.
- Lanz, V. A., Alfarra, M. R., Baltensperger, U., Buchmann, B., Hueglin, C., and Prévôt, A. S. H. (2007). Source Apportionment of Submicron Organic Aerosols at an Urban Site by Factor Analytical Modelling of Aerosol Mass Spectra. Atmos. Chem. Phys., 7:1503–1522.
- Lee, C. (2004). Design and Evaluation of 4-Stage Low Pressure Cascade Impactor using Electrical Measurement System. Master's thesis, Gwangju Institute of Science and Technology, Gwangju, Republic of Korea.
- Liu, P. S. K., Deng, R., Smith, K. A., Williams, L. R., Jayne, J. T., Canagaratna, M. R., Moore, K., Onasch, T. B., Worsnop, D. R., and Deshler, T. (2007). Transmission Efficiency of an Aerodynamic Focusing Lens System: Comparison of Model Calculations and Laboratory Measurements for the Aerodyne Aerosol Mass Spectrometer. Aerosol Sci. Technol., 41:721–733.
- Marple, V. A. and Willeke, K. (1976). Impactor Design. Atmospheric Environ., 10:891–896.
- Matthew, B. M., Middlebrook, A. M., and Onasch, T. B. (2008). Collection Efficiencies in an Aerodyne Aerosol Mass Spectrometer as a Function of Particle Phase for Laboratory Generated Aerosols. Aerosol Sci. Technol., 42: 884–898.
- McMurry, P. H. (2000). A Review of Atmospheric Aerosol Measurements. Atmos. Environ., 34:1959–1999.
- Middlebrook, A. M., Murphy, D. M., Lee, S. H., Thomson, D. S., Prather, K. A., Wenzel, R. J., Liu, D. Y., Phares, D. J., Rhoads, K. P., Wexler, A. S., Johnston, M. V., Jimenez, J. L., Jayne, J. T., Worsnop, D. R., Yourshaw, I., Seinfeld, J. H., and Flagan, R. C. (2003). A Comparison of Particle Mass Spectrometers During the 1999 Atlanta Supersite Project. J. Geophys. Res. D, 108:8424, doi:10.1029/2001JD000660, D7.
- Miyakawa, T., Matsuzawa, R., Katayama, M., and Takegawa, N. (2013). Reconsidering Adhesion and Bounce of Submicron Particles Upon High-Velocity Impact. Aerosol Sci. Technol., 47:472–481.
- Nash, D. G., Baer, T., and Johnston, M. V. (2006). Aerosol Mass Spectrometry: An Introductory Review. Int. J. Mass Spectrom., 258:2–12.
- Ng, N. L., Canagaratna, M. R., Jimenez, J. L., Zhang, Q., Ulbrich, I. M., and Worsnop, D. R. (2011). Real-Time Methods for Estimating Organic Component Mass Concentrations from Aerosol Mass Spectrometer Data. Environ. Sci. Technol., 45:910–916.
- Noble, C. A., and Prather, K. A. (2000). Real-Time Single Particle Mass Spectrometry: A Historical Review of a Quarter Century of the Chemical Analysis of Aerosols. Mass Spectrom. Rev., 19:248–274.
- Paatero, P. (1997). Least Squares Formulation of Robust Non-Negative Factor Analysis. Chemometr. Intell. Lab. Syst., 37:23–35.
- Paatero, P., and Tapper, U. (1994). Positive Matrix Factorization: A Non-Negative Factor Model with Optimal Utilization of Error Estimates of Data Values. Environmetrics, 5:111–126.
- Quinn, P., Bates, T., Coffman, D., Onasch, T., Worsnop, D., Baynard, T., De Gouw, J., Goldan, P., Kuster, W., and Williams, E. (2006). Impacts of Sources and Aging on Submicrometer Aerosol Properties in the Marine Boundary Layer Across the Gulf of Maine. J. Geophys. Res. D, 111:D23S36, doi:10.1029/2006JD007582.
- Salcedo, D., Onasch, T., Canagaratna, M., Dzepina, K., Huffman, J., Jayne, J., Worsnop, D., Kolb, C., Weimer, S., and Drewnick, F. (2007). Technical Note: Use of a Beam Width Probe in an Aerosol Mass Spectrometer to Monitor Particle Collection Efficiency in the Field. Atmos. Chem. Phys., 7:549–556.
- Stein, S. W., Turpin, B. J., Cai, X., Huang, P. F., and McMurry, P. H. (1994). Measurements of Relative Humidity-Dependent Bounce and Density for Atmospheric Particles using the DMA-Impactor Technique. Atmos. Environ., 28(10):1739–1746.
- Suess, D. T., and Prather, K. A. (1999). Mass Spectrometry of Aerosols. Chem. Rev., 99:3007–3035.
- Takami, A., Miyoshi, T., Shimono, A., and Hatakeyama, S. (2005). Chemical Composition of Fine Aerosol Measured by AMS at Fukue Island, Japan During APEX Period. Atmos. Environ., 39:4913–4924.
- Takegawa, N., Miyazaki, Y., Kondo, Y., Komazaki, Y., Miyakawa, T., Jimenez, J. L., Jayne, J. T., Worsnop, D. R., Allan, J. D., and Weber, R. J. (2005). Characterization of an Aerodyne Aerosol Mass Spectrometer (AMS): Intercomparison with Other Aerosol Instruments. Aerosol Sci. Technol., 39:760–770.
- Takegawa, N., Miyakawa, T., Nakamura, T., Sameshima, Y., Takei, M., Kondo, Y., and Hirayama, N. (2012). Evaluation of a New Particle Trap in a Laser Desorption Mass Spectrometer for Online Measurement of Aerosol Composition. Aerosol Sci. Technol., 46:428–443.
- Takegawa, N., Moteki, N., Koike, M., Oshima, N., and Kondo, Y. (2013). Condensation Particle Counters Combined with a Low-Pressure Impactor for Fast Measurement of Mode-Segregated Aerosol Number Concentration. Aerosol Sci. Technol., 47:1059–1065.
- Topping, D., Coe, H., McFiggans, G., Burgess, R., Allan, J., Alfarra, M. R., Bower, K., Choularton, T. W., Decesari, S., and Facchini, M. C. (2004). Aerosol Chemical Characteristics from Sampling Conducted on the Island of Jeju, Korea During ACE Asia. Atmos. Environ., 38:2111–2123.
- Tsai, C.-J., and Cheng, Y.-H. (1995). Solid Particle Collection Characteristics on Impaction Surfaces of Different Designs. Aerosol Sci. Technol., 23:96–106.
- Ulbrich, I. M., Canagaratna, M. R., Zhang, Q., Worsnop, D. R., and Jimenez, J. L. (2008). Interpretation of Organic Components from Positive Matrix Factorization of Aerosol Mass Spectrometric Data. Atmos. Chem. Phys. Discuss., 8:6729–6791.
- Virtanen, A., Joutsensaari, J., Koop, T., Kannosto, J., Yli-Pirilä, P., Leskinen, J., Mäkelä, J. M., Holopainen, J. K., Pöschl, U., and Kulmala, M. (2010). An Amorphous Solid State of Biogenic Secondary Organic Aerosol Particles. Nature, 467:824–827.
- Virtanen, A., Kannosto, J., Kuuluvainen, H., Arffman, A., Joutsensaari, J., Saukko, E., Hao, L., Yli-Pirilä, P., Tiitta, P., and Holopainen, J. (2011). Bounce Behavior of Freshly Nucleated Biogenic Secondary Organic Aerosol Particles. Atmos. Chem. Phys., 11:8759–8766.
- Wall, S., John, W., Wang, H.-C., and Goren, S. L. (1990). Measurements of Kinetic Energy Loss for Particles Impacting Surfaces. Aerosol Sci. Technol., 12:926–946.
- Zhang, Q., Jimenez, J. L., Canagaratna, M. R., Ulbrich, I. M., Ng, N. L., Worsnop, D. R., and Sun, Y. (2011). Understanding Atmospheric Organic Aerosols via Factor Analysis of Aerosol Mass Spectrometry: A Review. Anal. Bioanal. Chem., 401:3045–3067.
- Zhang, Q., Rami Alfarra, M., Worsnop, D. R., Allan, J. D., Coe, H., Canagaratna, M. R., and Jimenez, J. L. (2005). Deconvolution and Quantification of Hydrocarbon-Like and Oxygenated Organic Aerosols Based on Aerosol Mass Spectrometry. Environ. Sci. Technol., 39:4938–4952.