Abstract
Because of the rise of anthropogenic sources of NP human exposure to NP has dramatically increased in the recent years, in the general population as well as in workers (e.g., welders). This raises the question of the potential adverse effects of NP on human health, particularly at the respiratory level, since it represents the main route of exposure for air pollutants. Since inhalation exposure is the most relevant but yet under evaluated route of exposure for the evaluation of NP toxicity our study was aimed to design, build, and characterize a safe inhalation system dedicated to evaluate the respiratory effects of NP in mice. We chose to focus on the generation of iron (Fe) and manganese (Mn) oxide NP distributions centered on 20–25 nm diameters at concentrations of 107–108 NP/cm3 as it is representative of the occupational exposure of welders. Fe and Mn NP aerosols were generated with the spark discharge generator technique with air as a carrier gas conditio sine qua non for the mice. Indeed, the spark generator has been widely studied and used but in noble gases (N2, Ar, . . .) suggests the absence of oxidation. Aerosol particles are characterized in terms of size distribution, concentration, morphology, and chemical composition. Exposure of mice for 1 to 4 days (3 h a day) to these occupationally relevant aerosol concentrations induces inflammatory effects (increased lung total protein content). Moreover, clusters of particles were observed throughout the lungs.
Copyright © 2015 American Association for Aerosol Research
INTRODUCTION
Nanoparticles can be found naturally or as a result from human activities. These latter NP can be produced either intentionally because of the novel properties—useful for various applications—they display (engineered NP) or generated unintentionally (during domestic activities such as cooking, smoking, incense burning, or in welding fumes) (Stephenson et al. Citation2003; Marconi et al. 2006; Ji et al. Citation2010). Because of the rise of anthropogenic sources of NP (and particularly the engineered ones) human exposure to NP has dramatically increased in the recent years, in the general population as well as in the context of occupational exposure (Seaton et al. Citation2010). This is accompanied by questions on the potential adverse effects of NP on human health, particularly at the respiratory level since it represents the major route of exposure for air pollutants (Boczkowski and Lanone Citation2012).
In the literature the most commonly used experimental set-up to explore the issue of NP respiratory effects is to expose animals (usually mice or rats) by intra-tracheal or oro-pharyngeal instillation (Zhu et al. Citation2008; Beck-Speier et al. Citation2009; Zhu et al. Citation2009; Roulet et al. Citation2012; Saber et al. Citation2012). Although such route of exposure is easy to install and minimizes the risk of exposure of laboratory workers it also presents several drawbacks. First, although instillation allows the bench scientist to assure the actual dose delivered to the lungs of each animal, in such experimental set-up NP are given as a (usually single) bolus, under anesthesia, bypassing the upper respiratory tract which can be an important target site in real-life exposure (Driscoll et al. Citation2000). Second, the use of instillations implies that NP are provided as suspension not as powders. These suspensions depending on the dispersant will lead to the formation of agglomerates/aggregates of different number concentration, size, stability, and will behave differently from a powder of similar NP composition. These parameters can largely influence the subsequent biological effects of a given NP (Monteiro-Riviere et al. Citation2005; Li et al. Citation2007; Shvedova et al. Citation2008; Tabet et al. Citation2009; Andujar et al. Citation2014). Andujar et al. (Citation2014). Recently evidenced the presence of such NP in lung tissue from welders, and demonstrate their importance in the lung tissue alterations observed in welders.
Altogether these considerations lead to a preference of inhalation exposure for the evaluation of NP. This route of exposure is indeed the most relevant to real-life exposure as it provides a natural route of entry to the host without any dispersant needed and allows daily repeated exposures without any anesthesia. However, inhalation exposure is not often used in toxicological studies as designing and building an inhalation exposure system with appropriate generation and characterization of exposure atmospheres involves special expertise and equipment that are not commonly used in biology laboratories. Moreover, since the material is administrated as a powder, safety issues are particularly important to consider with such inhalation system and can put a break on its development.
The aim of the present work is therefore to design, build, and characterize a safe generation and inhalation system dedicated to study the respiratory effects of NP in mice. We chose to focus on the generation of iron (Fe) and manganese (Mn) oxide NP diameter distribution centered on 20–25 nm diameters, at concentrations of 107–108 NP/cm3. This is representative of the occupational exposure of welders (Li, 2004; Flynn and Susi Citation2009; Simon-Deckerset al. 2013; Antonini et al. Citation2011; Andujaret al. 2014; Park et al. Citation2014).
MATERIALS AND METHODS
Experimental Set-Up
The experimental set-up is shown in . It consists of an aerosol generation system coupled to an airtight enclosure containing the inhalation chamber. The aerosol is produced by a spark discharge generator (SDG) using dry and filtered compressed air as the carrier gas rather than nitrogen used usually. The aerosol is directly delivered to the inhalation chamber. The entire set-up is placed under a laminar fume hood, to avoid atmospheric particles to enter the inhalation chamber and thus make sure only the generated NP are inhaled by the animals.
Aerosol Generation and Characterization
Generation System
The generation system used in this study is based on the spark discharge method initially described by Andreas Schmidt-Ott in 1988 (Schwyn et al. Citation1988) and widely described in the literature (Evans et al. Citation2003; Roth et al. Citation2004; Szymczack et al. Citation2006; Tabrizzi et al. Citation2009; Boddu et al. Citation2011) This homemade spark generator consists of five arms high vacuum NW 40 stainless steel cross (Kurt Lesker Company, UK). Two electrical feedthrough are used to hold the grounded and the high voltage cylindrical electrodes to supply the potential difference between them. The two opposite arms are used as the inlet of the carrier gas and as the outlet of the generated aerosol, respectively. The fifth arm is equipped with a viewport to enable the observation of the sparks produced between the electrodes.
The position of the grounded electrode inside the chamber can be adjusted to vary the gap distance between both electrodes from zero up to a few millimeters. The electrodes (supplied from Good Fellow®, Lille, France) are 6 mm in diameter and 2 cm in length. They are connected to a high voltage power supply (0–10 kV and 0–10 mA,) and placed in parallel to a high voltage capacitor. The power supply (Sefelec, Lognes, France: 0–10 kV 30 mA maximum) delivers a constant current which is periodically recharging a high voltage capacitor after the discharge has occurred at the breakdown voltage. The energy dissipated by every discharge is given by the capacitance C and the discharge voltage V. The voltage V itself depends on the electrode gap distance. The energy E (Joules) per spark is given by:[1]
C is the capacitance of the high voltage capacitor in Farads, and V the voltage of the power supply in Volts. The capacitance C was maintained at 8.8 nF during all experiments. The voltage at the power supply was set between 2 and 6 kV. The compressed filtered and dry air flow rate is varied between 10 and 30 L/min.
Aerosol Characterization
Size Distribution and Concentration
The size distribution of the NP produced by the SDG is measured with a scanning mobility particle sizer (SMPS, TSI Inc, St. Paul, MN, USA) with a long DMA. The flow rates in the DMA were 1 lpm aerosol and 10 lpm sheath flow. The typical scanning times were 120 and 60s (up and down, respectively). With this scanning rate, the convective diffusion effect in tube flow from the DMA to the CPC described by Gomes et al. (Citation2012) was negligible. Fifteen measurements were performed for each aerosol distribution.
In the other hand particles are collected on TEM grids and further analyzed with Image J software (Image J, 1.46, 32-bit Java, National Institutes of Health, Bethesda, MD: http://rsb.info.nih.gov/ij/) for size determination.
Morphology and Chemical Composition
In order to characterize the morphology and chemical composition of the NP, they were collected on several TEM grids disposed on a 40 mm polycarbonate membrane with 0.8 μm pore diameters. The polycarbonate membrane is disposed in a 40 mm filter holder. The Grids were analyzed under an analytic transmission electron microscopy (ATEM, JEOL-JEM-1400 Electron Microscope, Oxford Instruments) coupled to an Inca X-sight camera. NP chemical composition was assessed by energy dispersive x-ray (EDX) analysis.
Inhalation Chamber
Ensuring the nose-only exposure of mice to the NP aerosol, we designed and built (Anitech, Pantin, France) a dedicated nose-only laminar exposure system with a common distribution system. It consists of a stainless steel central tube (where NP flow), with 12 containment tubes (6 on each side) connected all along (). The central tube presents a 3.6 cm2 cross section and is 38.7 cm long. The inlet and outlet of the tube are conically shaped; the NP aerosol enters from left to right at a flow rate of 10 lpm. Each containment tube (11 cm in length) is dedicated to host a mouse. A small (0.8 cm) circular opening at one end is dedicated to the muzzle of the animal. The other end of the tube can be sealed by a closing trap door that pushes the animal inside its compartment to prevent a retreat movement. The inner diameter of the tube measures 30 cm, small enough to prevent any turning or moving back of the mouse from the inhalation opening.
In order to avoid any potential contamination of external air with the generated NP aerosol, the inhalation exposure system itself has been placed inside a closed larger box (‘chamber of exposure’, 500 × 500 mm).
Animal Exposure
Our protocol of exposure has been approved by our institutional Ethic Committee (C2EA-16, Comité d’éthique ComEth ANSES/ENVA/UPEC). C57/Bl6 mice were exposed to the NP aerosols or to filtered air (n = 4–6 mice per group) for 3 h a day during 1 and/or 4 days. Twenty-four after the last exposure, mice were anesthetized, a broncho-alveolar lavage (BAL) was performed, and the lungs were removed, and further fixed in 4% formaldehyde and embedded in paraffin. Total and differential cellularity and total protein content (Bradford assay) were analyzed as previously described (Roulet et al. Citation2012). IL-1ß, TNF-α and TGF-ß content in BAL were measured by ELISA (R&D Systems, France), as per the manufacturer instructions. Histological analysis was performed on sagittal sections (5 μm) of paraffin-embedded lungs stained with hematoxylin eosin safran (HES).
Statistical Analysis
The statistical analyzes were made on GraphPadPrism software (GraphPadPrism 5.01, La Jolla, CA, USA). The different comparisons were performed with a Kruskal–Wallis test (nonparametrical) combined with a Dunn post test. The results were considered as significant if p < 0.05.
RESULTS
Combined variations of voltage of the power supply and carrier gas flow rate can largely influence the size distribution of the generated NP (Tabrizi et al. Citation2009; Park et al. Citation2014). We therefore varied these parameters sequentially.
Effect of the Voltage V on the Aerosol Size Distribution
At first, we applied a constant flow rate (10 lpm) and varied the voltage applied between the electrodes from 2 to 6 kV. As shown in a constant flow rate of 10 lpm, and a voltage of 3 kV is enough to generate a diameter distribution around 20–25 nm, at a concentration between 107 and 108 particle/cm3. Higher voltage (4 or 6 kV) leads to the generation of larger NP. As for Mn NP, even using the lowest voltage of 2 kV, the mean diameter of the generated NP was larger than what was requested; the diameters obtained were centered on 40 nm or 30 nm at 2.4 or 2 kV, respectively.
Effect of the Gas Flowrate on the Aerosol Size Distribution
In a subsequent attempt to obtain NP of required distribution, we applied a constant voltage 3 for Fe and 2 kV Mn electrodes, while varying the flow rate of the carrier gas. From , it can be seen that the peaks of size for both distributions shift to smaller sizes with increasing flow rates. In a, we observed that a flow rate of 10 lpm is needed to generate an aerosol of Fe NP centered around 20–25 nm of diameter, at a concentration of 3 × 107 p/cm3. A three-fold higher flow rate (30 lpm) is needed to generate similar amounts of Mn NP centered on the same diameters (b). The flowrate of the carrier gas in the spark chamber has two different effects on the size and concentration of the generated particles. For the same electrical conditions (electrical power via the voltage the current and the capacitance) the flowrate of gas introduced in the chamber to carry the vapors (which will form particles by condensation) will cool down more or less the plasma. The concentration of the produced vapors is proportional to the temperature of the plasma. This can be seen clearly on and b.
Morphology, Size, and Chemical Composition of the NP
shows typical TEM images of Fe and Mn NP generated with our experimental set-up. Fe and Mn form oxides nanoparticles as single spheres and or aggregates of spheres.
The size distribution of the NP measured using Image J software is in agreement with data obtained with an SMPS and shows a size distribution centered around 20–25 nm for both aerosols. EDX analysis confirmed the chemical nature of the NP generated ().
FIG. 6. Energy dispersive x-ray (EDX) analysis of Mn and Fe oxides nanoparticles. (a) Iron oxide. (b) Mn oxide.
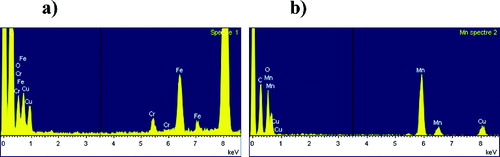
Chromium and cupper elements are also detected because of the chemical nature of the TEM observation grids. Their percentage is negligible compared to that related to the different NP.
Stability of the Aerosol Generation
As our experimental set-up is dedicated to perform repeated long-term (3 h a day over several weeks) exposure of mice, it was important to ensure the stability of the aerosol generation. Twelve h stability has been measured without changing the gap between the electrodes. The concentration number and size distribution show a high stability. demonstrates the stability of each aerosol particles over only 2 h (to facilitate readability) in terms of size distribution and concentration.
Flow Rate and Losses in the Inhalation Chamber
The flow inside the inhalation chamber is characterized by the Reynolds number (Re), which is given by:[2]
ρ is the density of the fluid in kg/m3; V is the mean velocity of the fluid in m/s; L is the traveled length of the fluid in meters; μ is the dynamic viscosity of the fluid in Pa.s. The flow rate inside the inhalation chamber is crucial, as NP concentration has to be the same for all mice throughout the inhalation chamber. To achieve this aim, the losses of particles inside the inhalation chamber have to be minimized at most. For particles of more or less 20 nm the diffusion is high and the effect of the inertia is negligible. Therefore a relatively high flow rate is needed to minimize particle losses. At the same time, the mice need to breathe calmly without a stream that could occur with a strong air draft. A flow rate of 10 lpm has therefore been chosen in our experiments (Re = 424). The velocity of the air is 0.16 m/s.
The experimental results for diffusion losses obtained at different points were compared to theoretical results calculated using the equation of Gormley and Kennedy (Citation1949) below:[3]
[4]
a = πDL/Q.
D is the diffusion coefficient of the particle given by the Einstein relation:[5]
Dp is the diameter of the particle,
k is the Boltzmann constant,
L is the travelled length in the inhalation chamber,
Q is the flow rate of air inside the chamber,
μ is the viscosity of the air,
C = 1+ (λ/Dp)(2.514 + 0.800 exp(−0.55Dp/λ)) the Cunningham coefficient, and λ the mean free path of the gas molecules.
We measured the concentrations of particles at the inlet and at different locations of the inhalation system for different diameters using two CPCs. The results with error bars of 1% are given in the online supplementary information. These results show that the losses in the chamber (at the different wholes of the chamber of inhalation) at a flow rate of 10 lpm are negligible even for particles of 10 nm.
Animal Exposure
No major histological modification was observed after exposure for 1 or 4 days to the different NP aerosols. However, clusters of NP were present in Mn-exposed mice already after 1 day exposure, and after a 4 days exposure to both NP aerosols (), mainly in alveolar and sub-pleural regions.
FIG. 8. Lung histology of mice after exposure to NP aerosol for 4 days. Representative images of mice lungs are given for Fe oxide (left column images), Mn oxide (middle column images) or unexposed (right column images) mice. White Circles surround NP clusters. Magnification: ×630.
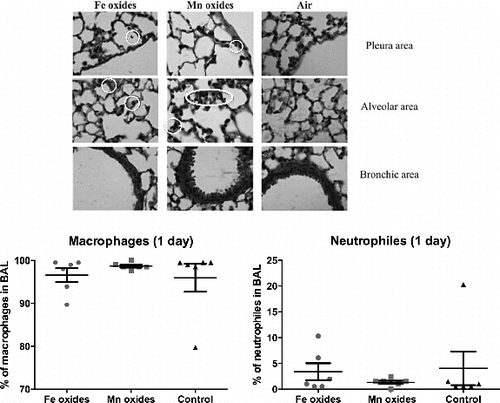
Total BAL fluid cellularity was not modified by exposure to NP aerosols. Moreover, no modification of BAL cell differential was observed in the exposed animals. However, the total protein content of the BAL fluid was significantly increased after a 4 days exposure to Mn NP aerosols. Finally, no modification of cytokine content (IL-1ß, TNF-α and TGF-ß) was observed after exposure of mice to the different NP.
DISCUSSION
In summary, the results of this work demonstrate the feasibility of our experimental setup to be used as a safe inhalation system dedicated to study the respiratory effects of NP in mice.
A continuous stable generation system of particles was needed to achieve our aim with a spark generator with air as carrier gas which produces a narrow peak of NP by the evaporation condensation of metallic vapors emitted continuously from a plasma between two electrodes kept at high voltage. The hot vapors cooled by a streamline of clean air at room temperature are oxidized before they condense and form an aerosol of NP in less than a second (Tabrizi et al. Citation2009). This aerosol is further sent through a very short tube (11 cm) inside the inhalation chamber with a flow rate of 10 lpm. This flow rate is important to consider as compared to the minute ventilation rate of mice of about 0.04 lpm (Mendez et al. Citation2010; Möller et al. Citation2013). Indeed, if the flow inside the chamber approaches too much the minute ventilation rate of the animal, the flow may be insufficient to clear the exhaled atmosphere away from the animal. The animal will begin to (re)-breathe its exhaled atmosphere, reducing the delivered dose and oxygen concentration while increasing the carbon dioxide concentration (Wong et al. 2007).
Compressed and filtered air is used contrary to the normal use of the spark method where chemically inert gases (nitrogen, argon) are in use. Oxides nanoparticles are then produced with high concentration high flowrate (up to 30 lpm) and high stability for the first time. Based on the number concentration of NP generated (107 p/cm3) and the respective density of Mn and Fe (7.3 g/cm3 and 7.86 g/cm3), the calculated mass concentration of each generated aerosol was ≈ 306 μg/m3 and 330 μg/m3 for Mn and Fe NP, respectively. Welders’ personal exposures to manganese has been measured at concentrations up to 4.93 mg/m3 and up to 16.29 mg/m3 for iron (Korczynski Citation2000; Flynn and Susi Citation2009), with total welding particulate levels up to 30 mg/m3 (Susi et al. Citation2000; Meeker et al. Citation2007; Zeidler-Erdely et al. Citation2012). Given that NP can represent up to 80% in number and 16% in mass of the total particles emitted during welding (Stephenson et al. Citation2003; Dasch and D’Arcy Citation2008), the aerosol generated in our study is therefore representative of welder's occupational environment. This is important to consider as our experimental set-up is dedicated to expose the animals repeatedly, over long periods of time, in order to mimic a worker's lifetime occupational exposure. Given the respiratory frequency and the tidal volume of a mouse, and assuming a respiratory minute volume of 0.04 l/min and a deposition fraction of 0.35 of the inhaled aerosol (Mendez et al. Citation2010; Möller et al. Citation2013), the estimated pulmonary deposited dose per h is 0.26 μg and 0.28 μg for Mn and Fe aerosol, respectively. This deposited dose, even after 3 h inhalation, is therefore by orders of magnitudes lower compared to other toxicological studies, which usually use single bolus instillation of particles (Kreyling et al. Citation2012). It surely adds to the relevance of our exposure system in the context of realistic toxicological studies.
The effect of the energy and the flow rate has been used as variable parameters to adjust the generated particle size to the requirements. We have chosen to work with the smallest value of voltage for evident security reasons. The flow rate has two effects. One is to cool the plasma and the vapors, while the second has to do with the dilution of the produced particles. The dilution given by the high gas flow rate decreases the probability for the particles to ‘meet’ and grow by coagulation. The stability of the aerosol generated was addressed in terms of generation but also of losses inside the inhalation chamber. Indeed, an important condition that has to be considered during the inhalation study is the requirement that all animals breathe the same air in terms of NP concentration; this concentration must be the same for the first animal to the last one in the inhalation chamber. This is challenging because of possible losses (diffusion losses for this diameter are the most important losses) inside the inhalation chamber. Particles below 50 nm present a high diffusion coefficient that makes them highly diffusive and give an increasing probability for deposition on the walls of the tubes with increasing diffusion coefficient meaning decreasing particle size. The way to minimize these diffusion losses is to work at high flow rate without any dilution of the concentration. In our experiments the flow rate inside the inhalation chamber was kept at 10 lpm. For this flow rate the losses were less than 1% which is remarkable in aerosol science.
We chose to develop a nose-only inhalation exposure system as it presents several advantages compared to whole-body systems. Even if whole-body chambers represent a “natural” exposure method, they allow other routes of exposure, such as the dermal and/or oral ones (Wong Citation2007). Nose-only systems imply the containment of the animals, and no water or food during the exposure, which could be a source of stress. Therefore, we performed preliminary experiments to address this issue, and observed that the animals didn't show any sign of discomfort after 2 weeks of daily exposure in such conditions (3 h a day, 5 days a week). Inhalation systems present large advantages compared to instillation. As stated earlier, instillation of particles implies that NP are given not as powders but as suspensions. In such preparations, NP are not present as single NP but usually as (large) agglomerates (Tabet et al. Citation2009; Porter et al. 2008; Möller et al. Citation2013), which will determine how many and where the particles may deposit within the respiratory tract. Consequently, it will also have implications in the translocation of the NP, and further biological effects (Li et al. Citation2007; Shvedova et al. Citation2008). The efficiency of our exposure system is attested by the presence of clusters of NP in mice lung (for both aerosols), as well as the inflammatory effects (significant increase in total lung protein content) that can be observed after a 4 days exposure to Mn aerosols.
CONCLUSION
A spark generator with air as carrier gas is characterized in terms of size distribution of oxides nanoparticles of Fe and Mn at substantial high flowrates (10–30 lpm) for inhalation studies. A complete safe inhalation system was developed using a spark discharge generator montage. This experimental set-up is fully adaptable in terms of chemical nature, size, concentrations of the NP generated and could be used to evaluate the effects of occupational-like exposures to NP. The preliminary results obtained in mice exposed up to 4 days demonstrate the relevance of our exposure system in terms of respiratory toxicology.
SUPPLEMENTAL MATERIAL
Supplemental data for this article can be accessed on the publisher's website.
UAST_1052037_Supplementary_File.zip
Download Zip (533.7 KB)REFERENCES
- Andujar, P., Simon-Deckers, A., Galateau-Sallé, F., Fayard, B., Beaune, G., Clin, B., Billon-Galland, M. A., Durupthy, O., Pairon, J. C., Doucet, J., Boczkowski, J., and Lanone, S. (2014). Role of Metal Oxide Nanoparticles in Histopathological Changes Observed in the Lung of Welders. Part FibreToxicol., 13:11–23.
- Antonini, J. M., Roberts, J. R., Stone, S., Chen, B. T., Schwegler-Berry, D., Rebecca Chapman, R., Zeidler-Erdely, P. C., Andrews, R. N., and Frazer, D. G. (2011). Persistence of Deposited Metals in the Lungs After Stainless Steel and Mild Steel Welding Fume Inhalation in Rats. Arch. Toxicol., 85:487–498.
- Beck-Speier, I., Kreyling, W. G., Maier, K. L., Dayal, N., Schladweiler, M. C., Mayer, P., Semmler-Behnke, M., and Kodavanti, U. P. (2009). Soluble Iron Modulates Iron Oxide Particle-Induced Inflammatory Responses via Prostaglandin E (2) Syntheses: In vitro and in vivo Studies. Part FibreToxicol., 6:34.
- Boddu, S. R., Gutti, V. R., Ghosh, T. K., Tompson, R. V., and Loyalka, S. K. (2011). Gold, Silver, and Palladium Nanoparticle/Nano-Agglomerate Generation, Collection, and Characterization. J. Nanopart. Res., 13:6591–6601.
- Boczkowski, J., and Lanone, S. (2012). Respiratory Toxicities of Nanomaterials—A Focus on Carbon Nanotubes. Adv. Drug. Deliv. Rev., 65:1694–1699.
- Brand, P., Bischof, K., Siry, L., Bertram, J., Schettgen, T., Reisgen, U., Kraus, T., and Gube, M. (2013). Exposure of Healthy Subjects with Emissions from a Gas Metal Arc Welding Process : Biological Effect Markers and Lung Function. Int. Arch. Occupat. Environ. Health, 86:39–45.
- Dasch, J., and D’Arcy, J. (2008). Physical and Chemical Characterization of Airborne Particles from Welding Operations in Automotive Plants. J. Occup. Environ. Hyg., 5:444–454.
- Driscoll, K. E., Costa, D. L., Hatch, G., Henderson, R., Oberdorster, G., Salem, H., and Schlesinger R. B. (2000). Intratracheal Instillation as an Exposure Technique for the Evaluation of Respiratory Tract Toxicity: Uses and Limitations. Toxicol. Sci., 55:24–35.
- Evans, D. E., Harrison, R. M., and Ayres, J. G. (2003). The Generation and Characterization of Elemental Carbon Aerosols for Human Challenge Studies. J. Aerosol Sci., 34:1023–1041.
- Flynn, M. R., and Susi, P. (2009). Manganese, Iron, and Total Particulate Exposures to Welders. J. Occup. Environ. Hyg., 7:115–126.
- Gomes, J. F. P., Albuquerque, P. C. S., Miranda, R. M. M., and Vieira, M. T. F. (2012). Determination of Airborne Nanoparticles from Welding Operation. J. Toxicol. Environ. Health, Part A, 75:747–755.
- Gormley, P. G., and Kennedy, M. (1949). Diffusion from a Stream Flowing Through a Cylindrical Tube. Proc. R. Irish Acad., 52:163.
- Ji, X., Le Bihan, O., Ramalho, O., Mandin, C., D’Anna, B., Martinon, L., Nicolas, M., Bard, D., and Pairon, J. C. (2010). Characterization of Particles Emitted by Incense Burning in an Experimental House. Indoor Air, 20(2):147–158.
- Korczynski, R. E. (2000). Occupational Health Concerns in the Welding Industry. Appl.Occup. Environ. Hyg., 15:936–945.
- Kreyling, W. G., Semmler-Behnke, M., Takenaka, S., and Müller, W. (2012). Differences in the Biokinetics of Inhaled Nano- Versus Micrometer-Sized Particles. Accounts Chem. Res., 46:714–722.
- Li, J. G., Li, W. X., Xu, J. Y., Cai, X. Q., Liu, R. L., Li, Y. J., Zhao, Q. F., and Li, Q. N. (2007). Comparative Study of Pathological Lesions Induced by Multiwalled Carbon Nanotubes in Lungs of Mice by Intratracheal Instillation and Inhalation. Environ. Toxicol., 22:415–421.
- Meeker, J. D., Susi, P., and Flynn, M.R. (2007). Manganese and Welding Fume Exposure and Control in Construction. J. Occup. Environ. Hyg., 4:943–951.
- Mendez, L. B., Gookin, G., and Phalen, R. F. (2010). Inhaled Aerosol Particle Dosimetry in Mice: A Review. InhalToxicol, 22(Suppl. 2):15–20.
- Möller, W., Gibson, N., Geiser, M., Pokhrel, S., Wenk, A., Takenaka, S., Schmid, O., Bulgheroni, A., Simonelli, F., and Kozempel, J. (2013). Gold Nanoparticle Aerosols for Rodent Inhalation and Translocation Studies. J. Nanoparticle Res., C7-1574 15:1–13.
- Monteiro-Riviere, N. A., Inman, A. O., Wang, Y. Y., and Nemanich, R. J. (2005). Surfactant Effects on Carbon Nanotube Interactions with Human Keratinocytes. Nanomedicine, 1:293–299.
- Park, J. H., Mudunkotuwa, I. A., Sung Kim, J. S., Stanam, A., Thorne, P. S., Grassian, V. H., and Peters, T.S. (2014). Physicochemical Characterization of Simulated WeldingFume from a Spark Discharge System. Aerosol Sci. Technol. 48:768–776.
- Roth, C., Ferron, G. A., Karg, E., Lentner, B., Schumann, G., Takenaka, S., and Heyder, J. (2004). Generation of Ultrafine Particles by Spark Discharging. Aerosol Sci. Technol., 38:228–235.
- Roulet, A., Armand, L., Dagouassat, M., Rogerieux, F., Simon-Deckers, A., Belade, E., Van Nhieu, J. T., Lanone, S., Pairon, J. C., Lacroix, G., and Boczkowski, J. (2012). Intratracheally Administered Titanium Dioxide or Carbon Black Nanoparticles Do Not Aggravate Elastase-Induced Pulmonary Emphysema in Rats. BMC Pulm. Med, 12:38.
- Saber, A. T., Jacobsen, N. R., Mortensen, A., Szarek, J., Jackson, P., Madsen, A. M., Jensen, K. A., Koponen, I. K., Brunborg, G., and Gutzkow, K. B. (2012). Nanotitanium Dioxide Toxicity in Mouse Lung is Reduced in Sanding Dust from Paint. Part. FibreToxicol, 9:4.
- Schwyn, S., Garwin, E., and Schmidt-Ott, A. (1988). Aerosol Generation by Spark Discharge. J. Aerosol Sci., 39–35:639–642
- Seaton, A., Tran, L., Aitken, R., and Donaldson, K. (2010). Nanoparticles, Human Health Hazard and Regulation. J. R. Soc. Interface, 7(Suppl 1):S119–129.
- Shvedova, A. A., Kisin, E. R., Murray, A. R., Johnson, V. J., Gorelik, O., Arepalli, S., Hubbs, A. F., Mercer, R. R., Keohavong, P., and Sussman, N. (2008). Inhalation Versus Aspiration of Single Walled Carbon Nanotubes in C57bl/6 Mice: Inflammation, Fibrosis, Oxidative Stress and Mutagenesis. Am. J. Physiol. Lung. Cell Mol. Physiol., 95:L552–565.
- Stephenson, D., Seshadri, G., and Veranth, J.M. (2003). Workplace Exposure to Submicron Particle Mass and Number Concentrations from Manual Arc Welding of Carbon Steel. AIHA J. (Fairfax, Va), 64:516–521.
- Susi, P., Goldberg, M., Barnes, P., and Stafford, E. (2000). The Use of a Task-Based Exposure Assessment Model (T-BEAM) for Assessment of Metal Fume Exposures During Welding and Thermal Cutting. Appl. Occup. Environ. Hyg, 15:26–38.
- Szymczak, W., Menzel, N., Kreyling, W. G., and Wittmaack, K. (2006). TOF-SIMS Characterization of Spark-Generated Nanoparticles Made from Pairs of Ir–Ir and Ir–C Electrodes. Intern. J. Mass Spec. 254:70–84.
- Tabet, L., Bussy, C., Amara, N., Setyan, A., Grodet, A., Rossi, M. J., Pairon, J. C., Boczkowski, J., Lanone, S. (2009). Adverse Effects of Industrial Multiwalled Carbon Nanotubes on Human Pulmonary Cells. J. Toxicol. Environ. Health, 72:60–73.
- Tabrizi, N. S., Ullmann, M., Vons, V. A., Lafont, U., and Schmidt-Ott, A. (2009). Generation of Nanoparticles by Spark Discharge. J. Nanopart. Res., 11:315–332.
- Wong, B. A. (2007). Inhalation Exposure Systems Design, Methods and Operation. Toxicol. Pathol., 35:3–14.
- Zeidler-Erdely, P. C., Erdely, A., and Antonini, J. M. (2012). Immunotoxicology of Arc Welding Fume: Worker and Experimental Animal Studies. J. Immunotoxicol., 9(4):411–425.
- Zhu, M.T., Feng, W. Y., Wang, B., Wang, T. C., Gu, Y. Q., Wang, M., Wang, Y., Ouyang, H., Zhao, Y. L., and Chai, Z. F. (2008). Comparative Study of Pulmonary Responses to Nano- and Submicron-Sized Ferric Oxide in Rats. Toxicology, 247:102–111.
- Zhu, M. T., Feng, W. Y., Wang, Y., Wang, B., Wang, M., Ouyang, H., Zhao, Y. L., and Chai, Z. F. (2009). Particokinetics and Extrapulmonary Translocation of Intratracheally Instilled Ferric Oxide Nanoparticles in Rats and the Potential Health Risk Assessment. Toxicol. Sci., 107:342–351.