Abstract
Although theoretical models of the filtration efficiency of fibrous filters are typically based on a single type of fiber in an ordered array, many actual fibrous filters comprises fibers that are inherently randomly distributed. It is desirable to be able to estimate the filtration efficiency of such non-uniform fibrous filters from their composition arrangement and the filtration property of individual fibers. Toward that end, we approximate the filter system as a series of cells comprising individual fibers with random distribution, deviating from the homogeneity assumption in the classical models. With better characterization of the filter structure based on the Voronoi diagram, we theoretically revisit filtration efficiency by the top-down (TD) approach and the bottom-up approach. The proposed models are compared with the existing experimental and numerical results under different fiber volume fractions, indicating the high accuracy of the TD model for the filtration of submicron aerosol particles. The influence of the degree of randomness of fiber distribution on filtration efficiency is also quantified.
Copyright 2015 American Association for Aerosol Research
1. INTRODUCTION
The increasing awareness of health and environmental agencies, together with the public, of the need for clean air has promoted a race around the world to develop high-performance filters for particle filtration. Fine particles come in many forms and sizes such as mist, smoke, dust, fog, and fume, and are released into the atmosphere from car engines, dust storms, power plants, bushfires, volcanoes, and so forth. Inhalation of these fine particles poses an irreversible threat to human health, and mortality rates are higher for individuals exposed to environments with high levels of fine particles such as particulate matter (PM) 2.5 (Tonne and Wilkinson Citation2013).
Among various filter systems, fibrous media comprising nano and micro cellulose, glass, or polymer fibers are the key elements of effective filtration devices for removing aerosol particles from air or gas streams (Brown Citation1993; Qin and Wang Citation2006). A commercial fibrous filter typically comprises a pad of loosely and randomly layered fibers that are predominantly normal to the aerosol flow direction (Figure S1 in the online supplemental information (SI)) (Wang and Otani Citation2013). Classical filtration theory of fiber-based filters is based on the principle of single fiber efficiency, defined as the quotient of the number of aerosol particles captured by a unit length of fiber to the total number of particles in the undisturbed mainstream passing through the projected area (Brown Citation1993). The filtration property is highly dependent on the flow field around the individual fiber, which is interfered by the effects of neighboring fibers (Lee and Liu Citation1982a). In a series of studies of the flow field around a fiber in a fiber array (Jackson and James Citation1986), the cell models (Happel Citation1959; Kuwabara Citation1959) have been verified as the most accurate ones (Dhaniyala and Liu Citation1999). However, the assumption of cell models is not met unless the fibers are in parallel and distributed in an orderly fashion (Figure S2a in the SI). An empirical inhomogeneity factor has been therefore added to account for the discrepancy between the order-based model and the experimental results of non-uniform fibrous filters (Lee and Liu Citation1982a). Yet this measure is not sufficient to characterize the non-uniformity of filter performance across different operational conditions (Dhaniyala and Liu Citation2001a).
To account for the effects of non-uniform and inhomogeneous fiber distribution, Schweers and Loffler Citation(1994) subdivided fibrous filters into a series of cells with different local directionally dependent permeabilities and calculated the local particle filtration based on the resulting three-dimensional (3D) flow field. Then a generalized model was developed for the calculation of aerosol filtration in a porous filter by considering the spatial distribution of local porosities (Shapiro Citation1996). Predicted results based on that model agreed qualitatively with the experimental data for a granular bed filter. Variations in local filtration efficiency have been experimentally observed by a filter scanner, indicating the packing density distribution of fibrous filters (Dhaniyala and Liu Citation2001b). Subsequently, the effect of the large-scale inhomogeneity or randomness of fiber distribution was characterized based on variations in the media packing density that were normal to the flow direction (Dhaniyala and Liu Citation2001a). These models were validated by experimental data for certain values of media inhomogeneity, namely the geometric standard deviation of the distribution.
With the development of high computational power, numerical reconstruction of the microstructures of actual fibrous filters has become possible. Dhaniyala and Liu Citation(1999) employed a 3D fiber system comprising square fibers to represent fibrous filters, and calculated the filtration efficiency using a finite volume approach. A better estimate of fiber drag was obtained and the influence of inter-fiber distance was characterized numerically. By analyzing microscopic images of lightweight spun-bonded filter media, Wang et al. Citation(2006) numerically generated a virtual 3D web based on the information about fiber orientation and packing. The effects of fiber volume fraction, filter thickness, and fiber and particle diameters were systematically analyzed, and the simulated results obtained were in a relatively good agreement with the experimental data obtained from a TSI 8130 filter tester. Later, it was found that the simulated pressure drop of a fibrous filter comprising randomly located fibers was lower than the theoretical model based on homogeneity assumption, whereas the estimation of filtration efficiency was consistent with the existing filtration models (Hosseini and Tafreshi Citation2010). It had been noted earlier that the classic models based on individual fibers or parallel fibers agreed fairly well with experimental results for randomly layered fibers with different in-fiber orientations (Lee and Liu Citation1982a,Citationb). In a recent numerical simulation, filtration efficiency was found to be unaffected by in-plane fiber orientation in the capture of nano- and submicron particles (i.e., 0.05–1 μm), but it increased with an increase in fiber directionality for large particles (i.e., 3–5 μm) (Fotovati et al. Citation2010).
Random lattices have been accurately characterized using the Voronoi diagram (Chen and Hlavacek Citation1994). This method was extended to model transverse and longitudinal flows across randomly distributed fibers, and the predicted permeabilities were well verified by experimental results (Shou et al. Citation2011, Citation2015). In this paper, we employ the Voronoi diagram to explore the filtration of submicron particles by non-uniform fibrous filters comprising randomly distributed circular fibers with a uniform diameter (Figure S2b in the SI). Here each fiber is embedded in a cell in which the Kuwabara flow field is considered. The cell areas vary, following the Gamma distribution based on the Voronoi diagram approximation (Chen and Hlavacek Citation1994; Ferenc and Neda Citation2007). The filtration efficiency is modified from that of the uniform filter in two ways: top-down (TD) and bottom-up (BU). In the TD approach, the whole non-uniform fibrous filter is assumed to be uniform, with a randomness-modified flow field. In the BU approach, the total filtration efficiency is an integrated result of the filtration efficiencies in all randomly located cells. The filtration efficiencies obtained based on the two estimates are compared with the existing experimental, numerical, and theoretical results. The effect of the micro-scale randomness of fiber distribution is also explored.
2. THEORETICAL MODELING
Determining the exact permeability or flow behavior of actual fibrous systems is not possible, considering the random nature of porous geometry (Tamayol and Bahrami Citation2009). Therefore, simplifying the representation of the non-uniform fibrous filter is required to model its filtration efficiency. Commercial fibrous filters always comprise randomly layered fibers in a nonwoven form (Wang and Otani Citation2013). Considering the negligible effect of in-plane fiber orientation on the filtration of submicron particles (Fotovati et al. Citation2010), we approximate non-uniform fibrous filters as parallel fiber bundles that are randomly distributed in 2D domain. As seen in Figure S2b, the center points of the fibers are assumed to be randomly located, and each point is included in a polygonal cell, namely the Voronoi cell. The boundary walls of the cells are defined by the perpendicular bisector between neighboring center points. The Gamma distribution has been successful in quantifying the size distribution of Voronoi cells (Ferenc and Neda Citation2007). In each cell, the flow field is assumed to follow the Kuwabara expression. Since we are concerned with a loose fibrous filter, the cell shape can be neglected as in the Kuwabara's Citation(1959) case. As well, the slip boundary condition at the fiber surface is assumed negligible, as we are focused on commercial micro-scale fibrous filters.
The total filtration efficiency of a uniform fibrous filter is derived on the basis of single fiber efficiency, making a mass balance for particles in a given filter and integrating from the filter face to the exit (Brown Citation1993),[1] where
is the filter efficiency, c is the fiber volume fraction, h is the filter thickness,
is the fiber diameter, and E is the single fiber efficiency. Inertial impaction of low-speed submicron particles is relatively less significant and is thus often negligible (Hosseini and Tafreshi Citation2010). The total single fiber efficiency is given by Brown Citation(1993),
[2] where
and
are single fiber efficiencies caused by interception and the Brownian diffusion, respectively. The expressions of
and
are given by Lee and Liu Citation(1982a),
[3] and
[4] respectively, where
is the Kuwabara hydrodynamic factor and
is the ratio of the particle diameter
to the fiber diameter
. In Equation Equation(4)
[4] , the Péclet number
is given by Brown Citation(1993),
[5] where
is the flow velocity in the filter and
is the particle diffusion coefficient, which is widely evaluated by the Stokes–Einstein equation (Wang and Otani Citation2013),
[6] where
is the temperature,
is the Boltzmann's constant, and
is the mean free path of gas molecules. Since
and
are very small, single fiber efficiency is always expressed in an additive form (Lee and Liu Citation1982a),
[7]
Based on Equations Equation(3)[3] and Equation(4)
[4] , it is evident that the Kuwabara hydrodynamic factor
is critical for filtration performance. Variations in flow fields due to non-uniformity may account for discrepancies in filtration efficiency. Here filtration efficiency is modified from that of the uniform fibrous filter by the TD and BU approaches.
In the TD approach, the entire non-uniform filter is assumed to be a uniform fibrous medium with a randomness-modified flow field. The permeability of ordered fiber arrays and each cell is given by[8] where the expression of
is given in accordance with (Kuwabara Citation1959):
[9]
For a fibrous system with randomly distributed fibers, cell permeability varies with the fiber volume fraction of the cell. It has been verified that the effective permeability of non-uniform fibrous media is area-averaged (Shou et al. Citation2011),
[10] where
indicates area-averaged, and the mean area
is given by
. It is evident that the mean fiber volume fraction of the non-uniform filter is given by
, where
is the area of the solid fiber. Here the probability density distribution function of cell areas
is expressed as follows (Ferenc and Neda Citation2007):
[11] where β is the scale parameter, and
is a Gamma distribution. The term
in Equation Equation(10)
[10] can be rewritten as
[12]
The term in Equation Equation(12)
[12] is area-averaged, viz,
[13] where s is the dimensionless area
. Analogously, the area-averaged values of
and
in Equation Equation(12)
[12] are given as follows:
[14] and
[15] respectively.
When the fiber distribution is fully random, β is found to be equal to 3.61, and the ratio is calculated as 1.28. When the fibers are packed in order, we can easily obtain
. Thus,
may indicate the degree of randomness of fiber distribution. With Equations Equation(10)
[10] –Equation(15)
[15] and
, we have the area-averaged form of
,
[16]
The modified hydrodynamic factor for a fully random fiber arrangement is readily obtained as follows:
[17]
For different values of , i.e., 1.21, 1.14, and 1.07, the corresponding modified Kuwabara hydrodynamic factors with different degrees of randomness are given by
[18]
[19] and
[20] respectively.
Hence, the TD filtration efficiency for a non-uniform fibrous filter is obtained based on Equations Equation(2)[2] –Equation(4)
[4] , Equation(16)
[16] –Equation(20)
[20] as
[21]
In the BU approach, the total filtration efficiency is integrated from the contributions of filtration efficiency in all cells. In this study, we assume a mesoscale local region that contains many fibers and is much smaller than the whole filter, so the cell areas in the local region also follow the Gamma distribution. As well, the concentration of aerosol particles is assumed to be uniform in the local region, considering the very small single fiber efficiencies. It is evident that the collected particles in a cell with given single fiber efficiency are proportional to the cell area. Thus, the total amount of collected particles per area by
fibers can be expressed as
[22] or
[23] where
is the number of particles per area, and
is the total area. Based on Equations Equation(22)
[22] and Equation(23)
[23] , we have the BU-based filtration efficiency as
[24]
Thus, based on Equations (2)–(4), the area-averaged results of and
in Equation Equation(24)
[24] are given by
[25] and
[26] respectively.
3. RESULTS AND DISCUSSION
Flow patterns, which are critical for determining the filtration behavior of fibrous filters, are highly dependent on the hydrodynamic factor. In , we compare our model of the hydrodynamic factor based on Equation Equation(17)[17] with experimental results and the Kuwabara's Citation(1959) hydrodynamic factor of Equation Equation(9)
[9] . For a given fiber volume fraction, the randomness-based hydrodynamic factor is expressed as Ku = −0.64lnc − 0.737 + c − 0.25c2. The experimental values are obtained from the work of Ingmanson et al. Citation(1959), who measured transverse flow across randomly layered glass, nylon, and paper fibers, Wheat Citation(1963), who studied transverse flow across layered glass fibrous filters, and Labrecque Citation(1968), who calculated the through-plane liquid permeability of fibrous mats. As well, the widely used empirical correlation by Davies Citation(1952) that fits numerous experimental results of transverse permeability of nonwoven filters with the fiber volume fraction is added for comparison. The experimental data were reported as flow resistance, Kozeny constants, or pressure drop. For better comparison, all the collected data are converted to hydrodynamic factors. From , good agreement can be observed between the predictions of Ku and the experimental results. Moreover, it is noted that random fibrous media are more permeable than ordered fiber arrays, because the hydrodynamic factor of the former is moderately higher than the Kuwabara's model of Equation Equation(9)
[9] . In comparison with ordered fiber arrays with a uniform pore size, the random fibrous system consists of pores with a wide size distribution. Consequently, the increased magnitude of permeability or hydrodynamic factor of large pores is much greater than the decreased magnitude of permeability or hydrodynamic factor of smaller pores, as permeability scales with the square of pore size.
FIG. 1. Variations of hydrodynamic factor with fiber volume fraction for flow past the plane defined by randomly distributed fibers.
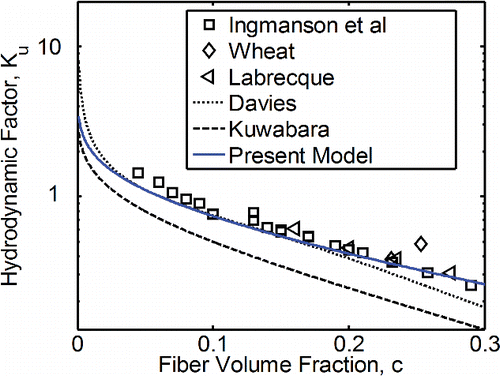
Next, we examine our models by comparison with experimental results for commercial filters comprising uniformly sized Dacron fibers (Lee and Liu Citation1982b). The structural parameters of the fibrous filters are summarized in . The mean free path of air molecules is 65 nm, the Boltzmann's constant
is 1.38 × 10−23 J/K, the air viscosity
is 18.27
, and the temperature is assumed to be 293 K. To assess the accuracy of the TD and BU models based on Equations Equation(21)
[21] and Equation(24)
[24] , respectively, we compare their results with the experimental results of Filter 1 with
in . The two models are based on the fully random approximation of fiber arrangements. shows that the TD model agrees fairly well with the experimental data over the range of particle diameters
, whereas the BU model slightly over-estimates the single filtration efficiency, especially for large particles. These results indicate that the TD approximation is reasonably reliable with the direct modification of the Kuwabara's flow field for the entire non-uniform fibrous filter. For the BU model, however, the assumption of uniform concentration of particles in the local area may be too rough, leading to less accurate prediction. In the following section, only the TD model is used for comparison with existing theoretical, numerical, and experimental results.
FIG. 2. Comparison of the predicted single fiber efficiencies based on the TD and BU models with experimental results (exp) (Lee and Liu Citation1982b), and at .
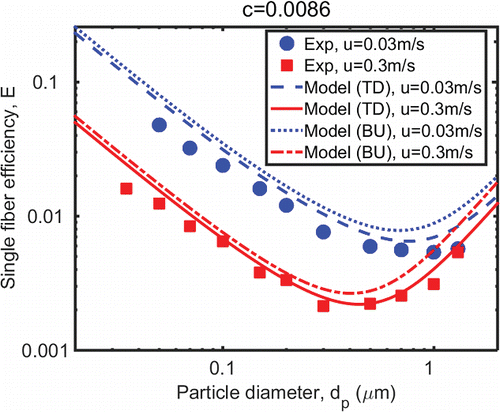
TABLE 1 Structural and physical parameters for the evaluation of commercial Dacron fibrous filters (Lee and Liu Citation1982b)
In , we compare the results from the TD model with the experimental results for Filter 2 with and the classical filtration model (Lee and Liu Citation1982a), named as the LL model. The curve of the TD model coincides with the plots of experimental data at
, but is slightly above the plots of nanoparticles (
) at
. The LL model is generally consistent with the experimental data, but it underestimates the single filtration efficiency in the medium regime of particles (
). Similar comparative phenomena are observed for denser filters (with
in Figure S3 and c =
in Figure S4 in the SI). The minor over-estimation of the TD model for nanoparticles (
) may be caused by particle rebound. In this diffusion-dominated regime, thermal rebound of nanoparticles occurs as their kinetic energy exceeds the adhesion energy, reducing the filtration efficiency of fibrous filter. The kinetic energy is even reversible by regaining from the thermal energy of the fiber (Brown Citation1993). For interception filtration, the gas flow velocity close to the fiber surface is very small, so the particles captured by the fiber seldom bounce and are difficult to escape from the surface. Thus, the TD model agrees closely with experimental data in the interception-dominated regime (
). Particle bounce has been experimentally observed by comparing the filtration behaviors between liquid and solid aerosols (Mullins et al. 2003). The solid aerosols exhibited smaller filtration efficiency than the liquid particles with much greater bonding capability (Mullins et al. 2003). It is noted that for the filtration of aerosol nanoparticles below 20 nm, thermal rebound was found only for the particles smaller than 2.5 nm (Heim et al. Citation2005). , S3, and S4 also show that filtration efficiency is higher under a lower flow velocity, a finding that is attributed to more adequate interaction between fibers and particles under a lower velocity flow field, especially for nanoparticles, due to diffusion filtration.
FIG. 3. Comparison of the predicted single fiber efficiencies based on the TD model with the theoretical model (Lee and Liu Citation1982a) and experiential results (Lee and Liu Citation1982b), and at .
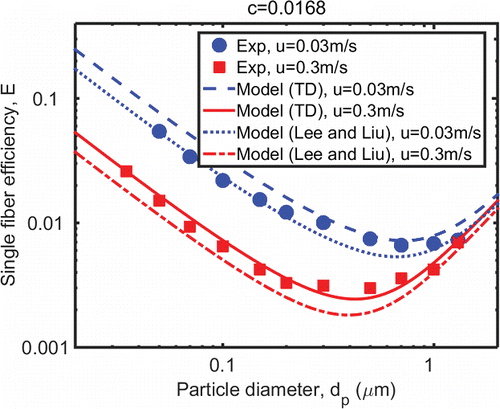
Single filtration efficiencies are dimensionalized by the order-based model of Equation Equation(7)[7] to compare the TD and LL models in . It is evident that the dimensionless single fiber efficiency of the LL model is close to 0.6. The deviation from 0.6 in the range of nanoparticles is caused by the minor simplification from Equation Equation(2)
[2] to Equation Equation(7)
[7] . However, by comparing Equation Equation(3)
[3] with Equation Equation(4)
[4] , we find that the diffusion filtration is less sensitive to the hydrodynamic factor or the flow stream than the interception filtration at a given Péclet number. It is expected that the diffusion filtration is the main result of the random Brownian motion of gas molecules, although the particles also move with the flow stream. For the TD model, it is found that the dimensionless single fiber efficiencies for nanoparticles are much greater than 0.6, whereas those for the coarse particles are close to the LL model. Hence, the TD-based findings agree qualitatively with the theoretical analysis. It is also shown that the dimensionless single fiber efficiencies decrease with an increase in the fiber volume fraction, indicating that the randomness effect is more pronounced for denser filters. This effect is consistent with the observation attributed to that variations in flow fields are less significant in looser fibrous media (Shou et al. 2014).
FIG. 4. Comparison of the predicted single fiber efficiencies based on the TD model with the theoretical model (Lee and Liu Citation1982a) under different fiber volume fractions.
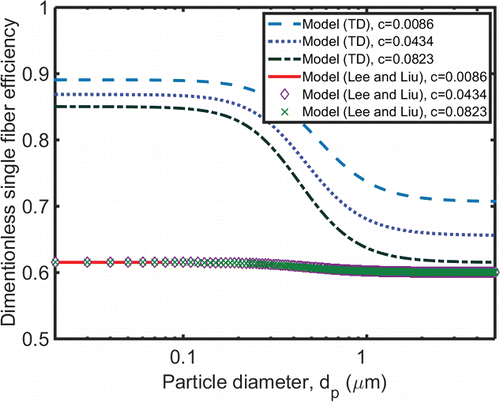
In particular, we compare the TD model with numerical results at (Hosseini and Tafreshi Citation2010) in when diffusion filtration dominates. The LL model is also added for comparison at
,
, and
(in Figures S5a–c, respectively, in the SI). In the numerical simulation, the fibers are randomly distributed in a 2D fashion, consistent with our assumption. These figures show the excellent accuracy of the TD model relative to the numerical results, whereas the result from the LL model is slightly lower than the numerical data. It is expected that the empirical factor is appropriate for the total single fiber efficiency of Equation Equation(7)
[7] , but that factor might not be best suited directly for the model of Equation Equation(4)
[4] for diffusion filtration.
FIG. 5. Comparison of the TD model for diffusion filtration with numerical simulations (num) (Hosseini and Tafreshi Citation2010) and the theoretical model (Lee and Liu Citation1982a) versus Péclet number at .
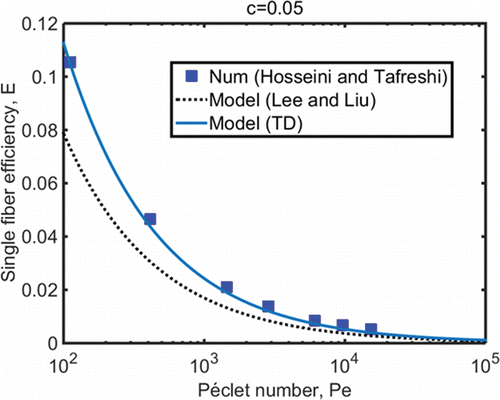
Finally, we explore the effect of the degree of randomness on filtration performance. In , the single filtration efficiencies for decrease with the randomness factor
for the filtration of three types of particles. The decrease is dramatic for the microparticle with
but is slow for the submicron particles with
and
. This phenomenon is attributed to the fact that the dominant diffusion filtration for filtration of nano- and submicron particles is less dependent on the flow field or the fiber arrangement. It is interesting to note that the decrease in single filtration efficiency is almost linear with
. In , similar results are shown for the filter with
. Nevertheless, the decrease in filtration efficiency due to higher randomness is even greater than that for
because the randomness effect is more critical for denser filters. Hence, based on the structural information, the present models can be used to model filtration efficiency ranging from fully random filters to ordered fiber arrays, depending on the filter manufacture.
4. CONCLUSIONS
Classical filtration theory for fibrous filters, widely used for several decades, has been established based on ordered fiber arrays. To better predict filtration efficiency, we modify filtration models by considering the randomness of fiber distribution in actual non-uniform fibrous filters. Introduction of randomness influence significantly improves the accuracy of filtration models and is expected to demonstrate the critical role of non-uniformity in fibrous filters. The proposed models are compared with experimental and numerical results under different fiber volume fractions, and satisfactory agreement is observed from hydrodynamic behavior to filtration efficiency. It is also found that diffusion filtration in a flow field is less sensitive than interception filtration. Furthermore, the degree of randomness of fiber distribution is quantified by a simplified randomness factor.
The present models cannot be directly used to characterize the influence of varying sizes and shapes of fibers. There are no simple expressions for predicting filtration efficiency of the filters comprising blends of fibers with varying sizes (Tafreshi et al. Citation2009). As well, the flow field around an elliptical fiber with different orientation angles is much more complicated than around a circular fiber, so the filtration efficiency of elliptical fibers has been generally modeled based on numerical simulation rather than analytical computation (Regan and Raynor Citation2009; Wang et al. Citation2014; Wang and Zhao Citation2015). It is also very computationally expensive to examine slip effect in the non-uniform fibrous filters at high Knudsen numbers. Hence, the above concerns will be addressed in the future work with the assistance of the Computational Fluid Dynamics (CFD) technique.
SUPPLEMENTAL MATERIAL
Supplemental data for this article can be accessed on the publisher's website.
UAST_1083092_Supplemental_Information.zip
Download Zip (826.8 KB)FUNDING
The co-authors Jintu Fan and Xiaoming Qian acknowledge the funding support of Tianjin City Applied Foundation & Emerging Technology Research Program (Grant No. 15JCZDJC38500).
REFERENCES
- Brown, R. C. (1993). Air Filtration: An Integrated Approach to the Theory and Application of Fibrous Filters. Pergamon Press, Oxford, UK.
- Chen, V., and Hlavacek, M. (1994). Application of Voronoi Tessellation for Modeling Randomly Packed Hollow-Fiber Bundles. Aiche J., 40:606–612.
- Davies, C. N. (1952). The Separation of Airborne Dust and Particles. Proc. Inst. Mech. Eng., B1:185–213.
- Dhaniyala, S., and Liu, B. Y. H. (1999). An Asymmetrical, Three-Dimensional Model for Fibrous Filters. Aerosol Sci. Technol., 30:333–348.
- Dhaniyala, S., and Liu, B. Y. H. (2001a). Theoretical Modeling of Filtration by Nonuniform Fibrous Filters. Aerosol Sci. Technol., 34:170–178.
- Dhaniyala, S., and Liu, B. Y. H. (2001b). Experimental Investigation of Local Efficiency Variation in Fibrous Filters. Aerosol Sci. Technol., 34:161–169.
- Ferenc, J. S., and Neda, Z. (2007). On the Size Distribution of Poisson Voronoi Cells. Physica A, 385:518–526.
- Fotovati, S., Tafreshi, H. V., and Pourdeyhimi, B. (2010). Influence of Fiber Orientation Distribution on Performance of Aerosol Filtration Media. Chem. Eng. Sci., 65:5285–5293.
- Happel, J. (1959). Viscous Flow Relative to Arrays of Cylinders. Aiche J., 5:174–177.
- Heim, M., Mullins, B. J., Wild, M., Meyer, J., and Kasper, G. (2005). Filtration Efficiency of Aerosol Particles Below 20 Nanometers. Aerosol Sci. Technol., 39:782–789.
- Hosseini, S. A., and Tafreshi, H. V. (2010). Modeling Particle Filtration in Disordered 2-D Domains: A Comparison with Cell Models. Sep. Purif. Technol., 74:160–169.
- Ingmanson, W. L., Andrews, B. D., and Johnson, R. C. (1959). Internal Pressure Distributions in Compressible Mats Under Fluid Stress. TAPPI, 42:840–849.
- Jackson, G. W., and James, D. F. (1986). The Permeability of Fibrous Porous-Media. Can. J. Chem. Eng., 64:364–374.
- Kuwabara, S. (1959). The Forces Experienced by Randomly Distributed Parallel Circular Cylinders or Spheres in a Viscous Flow at Small Reynolds Numbers. J. Phys. Soc. Jpn., 14:527–532.
- Labrecque, R. P. (1968). The Effects of Fiber Cross-Sectional Shape on the Resistance to the Flow of Fluids through Fiber Mats. TAPPI, 51:8–15.
- Lee, K. W., and Liu, B. Y. H. (1982a). Theoretical-Study of Aerosol Filtration by Fibrous Filters. Aerosol Sci. Technol., 1:147–161.
- Lee, K. W., and Liu, B. Y. H. (1982b). Experimental-Study of Aerosol Filtration by Fibrous Filters. Aerosol Sci. Technol., 1:35–46.
- Mullins, B. J., Agranovski, I. E., and Braddock, R. D. (2003). Particle Bounce During Filtration of Particles on Wet and Dry Filters. Aerosol Sci. Technol., 37:587–600.
- Qin, X. H., and Wang, S. Y. (2006). Filtration Properties of Electrospinning Nanofibers. J. Appl. Polym. Sci., 102:1285–1290.
- Regan, B. D., and Raynor, P. C. (2009). Single-Fiber Diffusion Efficiency for Elliptical Fibers. Aerosol Sci. Technol., 43:533–543.
- Schweers, E., and Loffler, F. (1994). Realistic Modeling of the Behavior of Fibrous Filters through Consideration of Filter Structure. Powder Technol., 80:191–206.
- Shapiro, M. (1996). An Analytical Model for Aerosol Filtration by Non-uniform Filter Media. J. Aerosol Sci., 27:263–280.
- Shou, D., Fan, J., and Ding, F. (2011). Hydraulic permeability of fibrous porous media. Int. J. Heat Mass Trans., 54:4009–4018.
- Shou, D., Ye, L., and Fan, J. (2014). Gas Transport Properties of Electrospun Polymer Nanofibers. Polymer, 55:3149–3155.
- Shou, D., Ye, L., and Fan, J. (2015). On the Longitudinal Permeability of Aligned Fiber Arrays. J. Compos. Mat., 49:1753–1763.
- Tafreshi, H. V., Rahman, M. S. A., Jaganathan, S., Wang, Q., and Pourdeyhimi, B. (2009). Analytical Expressions for Predicting Permeability of Bimodal Fibrous Porous Media. Chem. Eng. Sci., 64:1154–1159.
- Tamayol, A., and Bahrami, M. (2009). Analytical Determination of Viscous Permeability of Fibrous Porous Media. Int. J. Heat Mass Transfer, 52:2407–2414.
- Tonne, C., and Wilkinson, P. (2013). Long-Term Exposure to Air Pollution is Associated with Survival Following Acute Coronary Syndrome. Eur. Heart J., 34:1306–1311.
- Wang, C.-S., and Otani, Y. (2013). Removal of Nanoparticles from Gas Streams by Fibrous Filters: A Review. Ind. Eng. Chem. Res., 52:5–17.
- Wang, H., Zhao, H., Wang, K., and Zheng, C. (2014). Simulating and Modeling Particulate Removal Processes by Elliptical Fibers. Aerosol Sci. Technol., 48:207–218.
- Wang, K., and Zhao, H. (2015). The Influence of Fiber Geometry and Orientation Angle on Filtration Performance. Aerosol Sci. Technol., 49:75–85.
- Wang, Q., Maze, B., Tafreshi, H. V., and Pourdeyhimi, B. (2006). A Case Study of Simulating Submicron Aerosol Filtration via Lightweight Spun-Bonded Filter Media. Chem. Eng. Sci., 61:4871–4883.
- Wheat, J. A. (1963). The Air Flow Resistance of Glass Fiber Filter Paper. Can. J. Chem. Eng., 41:67–72.