Abstract
The NCTU micro-orifice cascade impactor (NMCI) was redesigned and tested to enable the measurement and adjustment of the jet-to-plate distance (S) of the impactors by using a micrometer. Each stage of the NMCI contains an impaction plate and a nozzle plate which are separated. The bottom casing for impaction plate assembly and the nozzle plate holder of each stage are separated. Calibration results show that the cutoff aerodynamic diameter (dpa50) of the three lower stages (dpa50 = 180, 100, and 56 nm) are close to the nominal values given in Marple et al. Citation(1991). In addition, the S/W (W: nozzle diameter) effects on the dpa50 for the three lower stages were investigated and an empirical equation was developed to facilitate the prediction of dpa50 when the nozzle diameters may change slightly from one batch to another. The relationship between the nozzle diameter and pressure drop of the micro-orifice impactors at the fixed operational flow rate of 30 L/min was also established so that the nozzle diameters can be predicted from the pressure drop measurement. An empirical equation was proposed to express the correlation between the dimensionless cutoff size and the S/W ratio when considering isentropic flow to facilitate the design of micro-orifice impactors for S/W > 4. It is expected that the present NMCI could improve the accuracy of size classification of submicron particles below 180 nm, especially for nanoparticles.
Copyright 2015 American Association for Aerosol Research
1. INTRODUCTION
Cascade impactors are widely used to measure aerosol mass size distributions and collect samples for chemical analysis (Zhu et al. Citation2010, Citation2012; Chen et al. Citation2010, Citation2013; Kim et al. Citation2012; Kudo et al. Citation2012; Canepari et al. Citation2013; Li et al. Citation2013; Liu et al. Citation2013; Fang et al. Citation2014; Lin et al. Citation2014). Commercial cascade impactors with the cutoff aerodynamic diameter (dpa50) covering the nanosize range include the micro-orifice uniform deposit impactor (MOUDI) (MSP Corp., Shoreview, MN, USA; Marple et al. Citation1991), the quartz crystal microbalance (QCM) cascade impactor (California Measurements, Inc., Sierra Madre, CA, USA; Chaun Citation1970), and the electric low-pressure impactor (ELPI) (Dekati Ltd.; Keskinen et al. Citation1992). The Berner low-pressure impactor could also collect particles of very small sizes at the lower stages (Berner et al. Citation1979; Wang and John Citation1988). Extensive studies have been devoted to resolve the three major problems which may distort the size distributions when using cascade impactors. These problems include particle bounce from the collection surface, overloading of collected particles on the impaction plate, and interstage loss (Marple and Olson Citation2011). Oil-coated substrates (Reischl and John Citation1978; Turner and Hering Citation1987; Pak et al. Citation1992; Peters et al. Citation2001; Gulijk et al. Citation2003; Liu et al. Citation2011; Tsai et al. Citation2012), porous substrates (Huang et al. Citation2001, Citation2005), and specially designed substrates (Tsai and Cheng Citation1995; Chang et al. Citation1999) were used as impaction substrates to reduce solid particle bounce. For uncoated substrates used as a collection surface to avoid interference with chemical analysis from coating materials, conditioning the relative humidity of the incoming aerosols of the MOUDI was suggested to ensure that the measurement of mass distributions is not influenced by particle bounce (Vasiliou et al. Citation1999; Chen et al. Citation2011). To increase the particle loading capacity on impaction substrates, many efforts have been made using oil-soaked Teflon filters (Turner and Hering Citation1987; Tsai et al. Citation2012), rotating substrates (Marple et al. Citation1991; Tsai et al. Citation2012), and specially designed impaction plates (Tsai and Cheng Citation1995).
The most important characteristics for cascade impactors is the particle collection efficiency curve, while interstage loss may result in the shift of the curve to the smaller size (Liu et al. Citation2011) or even lift-up of the left tail end of the curve (Hillamo and Kauppinen Citation1991). Among the commercial cascade impactors, the MOUDI is one of the most commonly used devices for sampling nanoparticles (Chow and Watson Citation2007). To solve the problems of gear wear and sometimes failure of gear motor, a second generation MOUDI, MOUDI-II, was developed using internal electric motors to replace external gear system for rotating the impaction plates (Marple et al. Citation2014). For the MOUDI, the interstage loss was measured during its initial development (Marple et al. Citation1991). The losses were found to be >10% in the inlet and the first two stages and were several percent in the intermediate stages. However, the loss for nanoparticles in all stages was not tested. In our recent study, the NCTU micro-orifice cascade impactor (NMCI) was developed and tested to avoid possible particle clogging in the nozzles using new nozzle plates with smooth nozzle shape of 120 μm in thickness fabricated by the LIGA (Lithography, Electroplating, and Molding) process to replace the 7th–10th stages in one of the MOUDI (MSP Model 110) (Liu et al. Citation2013). The NMCI and the MOUDI were studied for the particle collection efficiency and nanoparicle interstage loss. The dpa50 of the 7th–10th stages of the NMCI was shown to be very close to the nominal values given in Marple et al. Citation(1991). Liu et al. Citation(2013) concluded that the nanoparticle loss in the 10th stage of the NMCI and the MOUDI should be considered when determining the mass distributions of nano-particles.
The design of the nozzle plates of Liu et al. Citation(2013) was also shown to improve the problem of nozzle clogging which results in an increase in the pressure across the cascade impactors and a decrease in the dpa50 values of the lower stages. As compared to the fragile structure of the existing nozzle plates of the MOUDI, the nozzle plates of Liu et al. Citation(2013) with a sturdy nozzle structure allow ultrasonication of the nozzle plates for better cleaning efficiency of deposited particles in the nozzles. The nozzle strength test for the 9th-stage nozzles of the NMCI was further conducted in this study. The results can be seen in Figure S1, which shows that the nozzle remains well-rounded without cracking after 1-h ultra-sonication.
Inertial impactors have also been studied through theoretical analysis by numerical methods (Marple Citation1970; Marple and Liu Citation1974; Marple and Willeke Citation1976; Rader and Marple Citation1985). The design guidelines of singe nozzle impactors were developed based on the theoretical analysis which predicts the cutoff diameter and the shape of the particle collection efficiency curves reasonably well. It was concluded that an impactor would provide sharp and predicable collection efficiency curves if the value of the jet Reynolds number (Re) was between 500 and 3000, and if the S/W ratios (S: jet-to-plate distance; W: nozzle diameter) was greater than 0.5 for round-nozzle impactors. Rader and Marple Citation(1985) recommended that the S/W ratio should be greater than unity for round-nozzle impactors to avoid sizable changes in the dpa50 resulting from small variations in the jet-to-plate distance. They indicated that the S/W ratio from 1 to 5 has almost no effect on the square root of the cutoff Stokes number at 50% particle collection efficiency, (Stk50)1/2, for single-nozzle impactors. In addition, the effects of gravitational force (Huang and Tsai Citation2001), impaction plate diameter and particle density (Huang and Tsai Citation2002), and porous substrates (Huang et al. Citation2001, Citation2005; Huang and Tsai Citation2003) on the particle collection efficiency were further considered to extend the previous traditional theory.
In the case of multinozzle impactors, quantitative analysis has not been performed numerically due to the complexity of the flow fields. Nearly all the theoretical work and much of the experimental work have been conducted on single nozzle impactors. The results of single-nozzle impactor analysis have often been applied to multinozzle impactors which are assumed to act as a group of individual nozzles (Fang et al. Citation1991; Kwon et al. Citation2002). However, this approach ignores an important fact that the interaction between the flows from adjacent nozzles can change the fluid mechanics in the impaction region, the location of the jet stagnation points, and the impaction characteristics of each nozzle. The particle collection efficiency was found to be influenced by the cross flow (Fang et al. Citation1991) and nozzle arrangement (Kwon et al. Citation2002). Moreover, the effect of the S/W ratio on the cutoff characteristics of the multinozzle impactors (i.e., micro-orifice impactors) has been studied (Gudmundsson et al. Citation1995; Kwon et al. Citation2002; Yao and Mainelis Citation2006). In Gudmundsson et al. Citation(1995), when , (Stk50)1/2 increased with an increasing S/W but decreased with an increasing Re at a fixed porosity (Po) which is defined as the total cross-sectional area of nozzles divided by the area of nozzle plate. An increase in the porosity also increased (Stk50)1/2 at similar Re. Kwon et al. Citation(2002) indicated that a slight change in S/W may influence the collection efficiency even when the S/W ratio remains within the recommended range for single-nozzle impactors. Increasing the S/W ratio from 1.75 to 2.25 was shown to decrease particle collection efficiency by more than 10%. Yao and Mainelis Citation(2006) investigated the effect of the S/W ratio on the physical collection efficiency of the multinozzle bioaerosol impactors. Results showed that the dpa50 decreased when the S/W ratio was decreased over the range from 2.7 to 9. The theoretical dpa50 were also found to be lower than the experimental values. Tsai et al. Citation(2012) and Liu et al. Citation(2013) found that the cutoff characteristics of the micro-orifice impactors are very sensitive to the S/W ratios for
. The results of these studies are limited to the variation of S/W ratio with the corresponding cutoff characteristics. A design guidance for the multinozzle impactors similar to that of the single-nozzle impactors is currently not available.
In the MOUDI, each stage contains an impaction plate and a nozzle plate. Both the bottom casing for impaction plate assembly and the nozzle plate holder are fixed together. The Teflon gasket between the bodies of two stages may wear off after long-term usage, leading to the change of jet-to-plate distance. This problem can be ignored for the upper stages of the MOUDI because of the relatively smaller change in the thickness of Teflon gasket as compared to the jet-to-plate distance. However, for the three lower stages of the MOUDI (dpa50 = 180, 100, and 56 nm), any tiny change in the thickness of Teflon gasket may result in a shift in the dpa50. To measure the S/W ratio accurately is important to maintain the correct dpa50 values to achieve the accurate size-classified measurements of submicron particles below 180 nm, especially for nanoparticles.
In this study, the NMCI was redesigned to enable the measurement and adjustment of the jet-to-plate distance of the impactors by using a micrometer. shows the schematic diagram of the present NMCI stage which is a modification of the typical MOUDI stage. Differing from the MOUDI, each stage of the NMCI contains an impaction plate and a nozzle plate for itself. The bottom casing for impaction plate assembly and the nozzle plate holder of each stage are separated. For all stages, the geometry and dimensions of the upper casing for the nozzle plate as well as the bottom casing for the impaction plate assembly are the same. The jet-to-plate distance of the NMCI impactors can be adjusted by placing a Teflon gasket with a known thickness between the upper and bottom casings. As shown in , the distance from the top of upper casing to the impaction substrate and the nozzle plate is determined by using a micrometer. The jet-to-plate distance (S) is obtained by deducting the distance from the top of upper casing to the impaction substrate (S1, to be measured when the nozzle plate holder is removed) from that to the top of the nozzle plate (S2) and the thickness of nozzle plate (S3).
FIG. 1. (a) Schematic diagram of the present NMCI stage and (b) the method to determine the jet-to-plate distance.
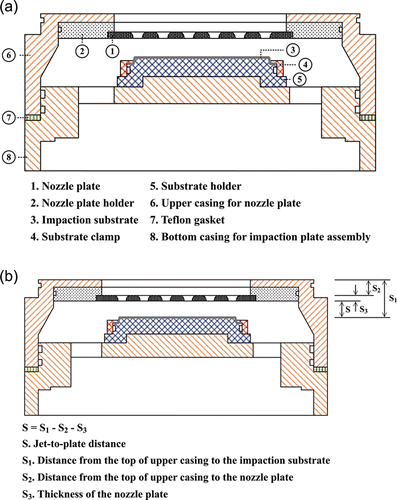
The nozzle plates of Liu et al. Citation(2013) with the Po of 0.8% were used in the 7th–10th stages of the present NMCI, while the nozzles of the nozzle plates in the 1st–6th stages were made by a mechanical drilling technique. To achieve a uniform particle deposition on substrate, the nozzle plate of each stage of the NMCI rotates while the impaction plate is fixed. In the laboratory, the S/W effects on the dpa50 for the three lower stages of the NMCI were investigated and an empirical equation was developed to facilitate the prediction of dpa50 when the nozzle diameters may change slightly from one batch to another. The relationship between the nozzle diameter and pressure drop of the micro-orifice impactors at the fixed operational flow rate of 30 L/min was also established so that the nozzle diameters can be predicted from the pressure drop measurement. Furthermore, the relationship between the dimensionless cutoff size and the S/W ratio when considering isentropic flow was investigated to facilitate the design of micro-orifice impactors.
2. EXPERIMENTAL METHOD
Before calibration, the diameter of the nozzles, both the inlet and outlet diameter, was examined using an optical microscope (model IM35, ESPA SYSTEMS Co., Ltd., Hsinchu, Taiwan). The experimental setup for measuring particle collection efficiency, which is the same as that in the earlier work (Liu et al. Citation2013), is described briefly in the following. Polydisperse aerosol particles were first generated by atomizing a solution containing dioctyl sebacate (DOS) with the concentration from 0.001 to 0.1% (v/v) using a TSI 3076 constant output atomizer and then passed through a tubular furnace (Lindberg/Blue M, model HTF55322C, Thermo Fisher Scientific Inc., Asheville, NC, USA) at a fixed temperature of 300°C by the evaporation–condensation technique. Monodisperse particles with the aerodynamic diameter from 15 to 500 nm were then generated by the electrostatic classifier (model 3080, TSI, Inc., Shoreview, MN, USA) equipped with the nanodifferential mobility analyzer (nano-DMA, TSI model 3085) or the long-DMA (TSI model 3081). A ball valve was used to simulate the pressure drop created by all previous upstream stages for calibrating the particle collection efficiency of a single lower stage of the present NMCI. The pressure difference across the impactor was determined from the inlet and corresponding exit pressures by a pressure gauge. The particle collection efficiency was calculated as the percentage of the aerosol currents at the inlet to that at the outlet of the tested impactors measured by the TSI 3068 aerosol electrometer equipped with a Faraday cage of our own design.
The square root of the cutoff Stokes number at 50% particle collection efficiency (Stk50)1/2 is defined as:[1] where ρp is the particle density (kg/m3), Cc is the slip correction factor, μ is the air dynamic viscosity (kg/m-s), W is the nozzle diameter (m), and V is the average air velocity at the outlet of the nozzle (m/s) determined using the mass conversion principle:
[2] where
is the volumetric flow rate at the inlet of the impactor (m3/s),
is the total pressure (Pa), and
is the air temperature upstream of the nozzle (K), P is the pressure (Pa) and T is the air temperature downstream of the nozzle (K), and A is the jet cross-sectional area (m2). The slip correction factor depending on temperature and pressure was calculated using the temperature and pressure upstream of the nozzle (Flagan Citation1982; Arffman et al. Citation2011). Jet velocity was calculated using the measured volumetric flow rate and stage operating pressures in this study. The sharpness of the collection efficiency curves was also characterized by the square root of the ratio of particle diameter with 84% collection efficiency to that with 16% efficiency.
In order to facilitate comparison when assuming isentropic flow (i.e., assuming compressible flow in the nozzle), for the 8th–10th stages of the NMCI, the square root of the cutoff Stokes number (Stk50, isen)1/2 was calculated with the nozzle velocity given by Equation (3) (Hering Citation1987; Hillamo and Kauppinen Citation1991):[3] where β is the average jet velocity for isothermal conditions:
[4] and k is the ratio of specific heats (1.4 for air), Ma is the average molecular weight of gas (28.96 kg/kmol for air),
is the universal gas constant (J/K-kmol), m is the mass flow rate (kg/s), and
is the stagnation air density upstream of the nozzle (kg/m3). Derivation of Equations (3) and (4) can be seen in the online supplementary information.
3. RESULTS AND DISCUSSION
3.1. Calibration Results
During the manufacturing process for the nozzle plates of the 7th–10th stages of the NMCI by the LIGA technique, a slight difference (±5%) in the nozzle diameters was found from one batch to another. There are 900–2000 micro-orifices with the diameter ranging from 140 to 52 μm used in these nozzle plates. As an alternative to the computer-controlled microscopic method for determining nozzle diameters, this study proposed an alternative method in which the relationship between the nozzle diameter and pressure drop of the micro-orifice impactors was established at the fixed flow rate of 30 L/min for estimating nozzle size from the pressure drop measurement.
For a flow through a perforated plate with rounded orifice edge for the Re greater than 3000, the resistance coefficient (K) exists, which can be calculated as (Fried and Idelchik Citation1989):[5] where
is the pressure difference (Pa),
is the velocity at the inlet of orifice (m/s),
is a coefficient depending on the shape of the orifice inlet edge and contraction angle determined from diagrams 3–7, 4–13, and 8–4 in Fried and Idelchik Citation(1989), and
is the free-area coefficient defined as:
[6] where
and
are the total cross-sectional area at the inlet and outlet of orifice (m2), respectively.
The Re of 7th–10th stages of the original NMCI of Liu et al. Citation(2013) is around 300–450. To correct Equation (5) for , the pressure drop across four nozzle plates with known nozzle inlet diameters of 261.3 ± 0.9, 260.1 ± 1.2, 334.6 ± 1.8, and 354.3 ± 1.5 μm and outlet diameters of 50.7 ± 0.3, 54.4 ± 0.4, 115.6 ± 0.5, and 141.8 ± 0.6 μm, respectively, at the flow rate of 30 L/min was measured. As shown in , an empirical equation was established to express the correlation between K and
as:
FIG. 2. Relationship of the resistance coefficient versus the free-area coefficient for the nozzles with known diameters at the flow rate of 30 L/min.
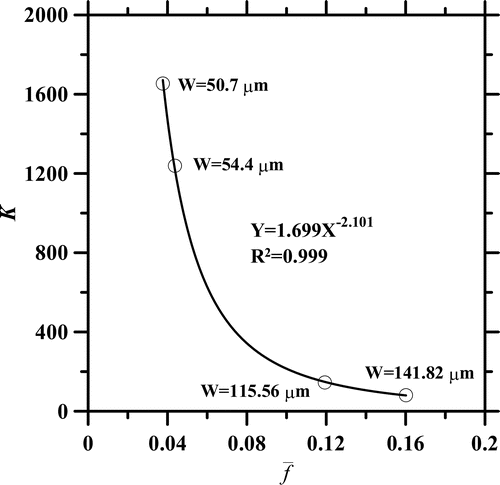
By subsituting , Equation (8) can be rearranged as:
[9]
Once F is obtained, the estimated nozzle diameter (We) can be calculated as:[10] where n is the number of nozzles. The average measured nozzle diameters of the 7th–10th stages of the NMCI were compared with the estimated values to examine the accuracy of this equation.
The nozzle diameters of the 7th–10th stages of the original NMCI were 138.7, 109.5, 54.3, and 50.9 μm, respectively, in which the nozzle diameter of the 10th stage was slightly smaller than the design value of 52 μm given in Marple et al. Citation(1991). It is expected that such a small nozzle used in the lowest stage of the redesigned NMCI could lead to a large pressure drop across the impactor. In this study, the nozzle plate of the 10th stage was replaced by one of the nozzle plates intended to be used for the 9th stage. The calibration results together with the design parameters are summarized in . The average nozzle diameters of the 7th–10th stages of the present NMCI were found to be 135.8±0.9, 108.1±0.8, 54.1±0.6, and 53.8±0.6 μm, respectively, which are very close to the predicted values with a deviation smaller than 1%. Equations (9) and (10) were verified by the experimental data and found to be correct. That is, the nozzle diameters of the micro-orifice impactors can be estimated from the pressure drop measurement as an alternative method in addition to traditional observation method by using an optical microscope.
TABLE 1 Calibration results and the design parameters of the four lower stages of the original and redesigned NMCI
shows the calibrated particle collection efficiency curves of the 8th–10th stages of the NMCI. The present design of the NMCI enables measurement and adjustment of the jet-to-plate distance of the impactors. After adjusting proper S/W ratios, calibration results show that the dpa50 of the 8th–10th stages of the NMCI are 180.4, 101.7, and 56.4 nm, respectively, which are close to the nominal values given in Marple et al. Citation(1991). The corresponding S/W ratios are 2.63, 14.27, and 12.64 at the jet-to-plate distance of 580, 780, and 680 μm, respectively. –c indicate that a change in jet-to-plate distance (i.e., S/W ratio) has a great influence on the dpa50 value for the micro-orifice impactor. For example, when the jet-to-plate distance of the 8th stage is increased from 210 to 980 μm, the dpa50 is found to increase from 155.5 to 214.8 nm. Similarly, the dpa50 increases with an increasing jet-to-plate distance for the 9th–10th stages. However, the variation of the sharpness of collection efficiency curves is not strongly related to the change in the jet-to-plate distance. For example, the sharpness of the collection efficiency curve are 1.21, 1.22, and 1.28 at the jet-to-plate distance of 210, 580, and 980 μm, respectively, for the 8th stage, while for the 9th stage the sharpness of the collection efficiency curve are 1.67, 1.52, and 1.63 at the jet-to-plate distance of 290, 780, and 980 μm, respectively. A decrease in the jet-to-plate distance resulting in a steeper collection efficiency curve was found in the 8th and 10th stages, but not found in the 9th stage. Results show that the dpa50 value was significantly affected by the S/W ratio for the three lower stages of the NMCI. Therefore, the relationship between the S/W ratio and dimensionless cutoff diameter of the micro-orifice impactors needs to be investigated.
3.2. Effect of S/W on Cutoff Characteristics
shows the effect of the S/W ratio on the pressure drop across the 8th–10th stages of the NMCI. For the 8th stage, when the S/W ratio is less than 4, the pressure drop increases sharply as the S/W ratio decreases. However, the pressure drop remains almost constant when the S/W ratio is greater than 4. A similar trend for the 8th stage is also observed for the 9th–10th stages when the S/W ratio is less than a certain value. This is due to a boundary layer effect of the exit flow from the impaction plate. Furthermore, the S/W ratios less than the minimum values shown in for these three stages were not considered in this study because of very large pressure drop.
The effect of the S/W ratio on the cutoff characteristics of the micro-orifice impactors was investigated by calibrating the dpa50 of the 8th–10th stages of the NMCI at several S/W ratios from 0.08 to 19.70. Results are shown in where (Stk50)1/2 is plotted against the S/W ratio. When the S/W ratio is less than 4, (Stk50)1/2 is found to approach a constant value with an increasing S/W, which agrees with the traditional theory of single-nozzle impactor (Marple and Liu Citation1974). However, when the S/W ratio is greater than 4, (Stk50)1/2 increases gradually with an increasing S/W. (Stk50)1/2 is seen to deviate from the theoretical value of Marple and Liu Citation(1974), 0.477 and that of Radar and Marple Citation(1985), 0.49. This finding is consistent with that found by Tsai et al. Citation(2012). In addition, the minimum S/W ratio of the 9th–10th stages corresponds to a higher (Stk50)1/2 than that of the 8th stage, because the pressure drop across the former is larger than the latter. The linearity of the S/W ratio and (Stk50)1/2 is found to be good over the S/W range of 4–20 with the coefficient of determination (R2) of 0.959.
The effect of the S/W ratio on the cutoff characteristics was further examined assuming isentropic flow in the 8th–10th stages of the NMCI and results are shown in . Compared to (Stk50)1/2 shown in , the values of (Stk50, isen)1/2 are nearly the same as (Stk50)1/2 for the 8th stage over the studied S/W range. However, (Stk50, isen)1/2 is found to decrease from 0.537 to 0.972 to 0.472 to 0.833 for the 9th stage and from 0.565 to 0.881 to 0.551 to 0.860 for the 10th stage. This is because the pressure ratio of downstream to upstream absolute pressure of the 9th and 10th stages ( is much less than 0.97, the limit to distinguish between compressible and incompressible flow stages (Biswas and Flagan Citation1984; Hillamo and Kauppinen Citation1991). The Mach number of the 8th–10th stages of the NMCI is also listed in Table S1. Over the S/W range of 4–20, the S/W ratio and (Stk50, isen)1/2 are found to be better correlated with the R2 of 0.986 by the following relationship:
FIG. 6. (a) Effect of the S/W ratio on the cutoff characteristics assuming isentropic flow of the 8th–10th stages of the NMCI and (b) comparison with the experimental data of Gudmundsson et al. Citation(1995).
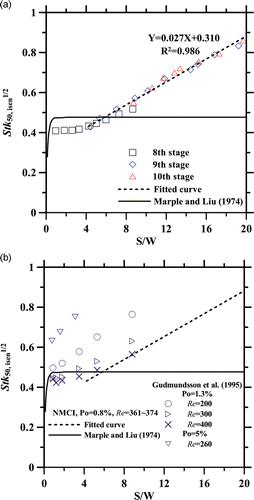
It is suggested that the nozzle velocity should be estimated by using the isentropic flow relationship for characterizing the cutoff characteristics of the three lower stages of the NMCI. Equation (11) was further validated by the experimental data of the micro-orifice impactor with smooth nozzle shape in Tsai et al. Citation(2012) with the porosity of 0.9% and the Re of 385. The dpa50 value estimated by Equation (11) is 120 nm which is close to the design value of 100 nm. Results show that the empirical formula derived from the three lower stages of the NMCI, which is suitable for predicting (Stk50, isen)1/2 as a function of the S/W ratio, can be used to facilitate the prediction of dpa50 and the design of the micro-orifice impactors with smooth nozzle shape manufactured by the LIGA method for . Furthermore, the normalization of dimensionless particle diameter can be seen in Figure S2, which shows that the collection efficiency curves of different jet-to-plate distance of individual stage shown in overlap when (Stkisen/Stk50, isen)1/2 is less than 1.0.
shows the comparison of the cutoff characteristics assuming isentropic flow of the present study with the previous experimental data in the literature. In , the experimental data of Gudmundsson et al. Citation(1995) was converted from (Stk50)1/2 to (Stk50, isen)1/2. The values of (Stk50, isen)1/2 of the three lower stages of the NMCI are all smaller than the values in Gudmundsson et al. in the entire range of S/W. This is due to a smaller porosity of 0.8% for the NMCI compared to that in Gudmundsson et al. Citation(1995), which is 1.3% for Re = 200, 300, and 400. A smooth nozzle shape in the NMCI also is different from the nozzle design of Gudmundsson et al. Citation(1995). However, (Stk50, isen)1/2 of the NMCI increases gradually with an increasing S/W even when the Re is increased. This means that the effect of Re on cutoff characteristics is negligible in this study because the varition of Re in the three lower stages of the NMCI is very small.
By adjusting the S/W ratios, the influence of the nozzle diameter on the cutoff characteristics was examined so that stage dpa50 is close to 100 nm when the nozzle plates have five different nozzle diameters of 52.7 ± 0.4, 53.7 ± 0.4, 54.2 ± 0.5, 54.8 ± 0.4, and 57.2 ± 0.4 μm, as shown in . The corresponding jet-to-plate distance were 894, 813, 781, 737, and 602 μm to achieve dpa50 of 99.8, 100.1, 100.2, 99.9, and 99.0 nm, respectively. Since the typical range of the manufactured nozzle diameter for the 9th stage of the NMCI is 53.7–54.8 μm, the jet-to-plate distance has to be adjusted from 813 to 737 μm.
FIG. 7. Relationship between nozzle diameter and (a) jet-to-plate distance and (b) cutoff aerodynamic diameter when the dpa50 is close to 100 nm.
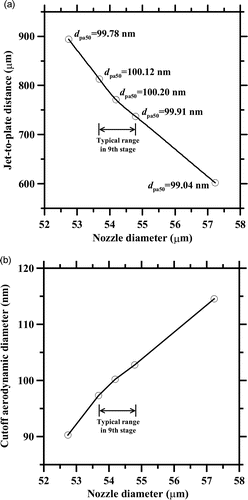
As shown in , when the jet-to-plate distance was fixed at 780 μm for the 9th stage with the nozzle diameter of 53.7–54.8 μm, the dpa50 was found to range from 97.3 to 102.8 with the average of 100 ± 3 nm. For 8th and 10th stages, a similar narrow manufactured nozzle size range yields dpa50 which are close to the specified values within 3 nm at fixed jet-to-plate distances of 580 and 680 μm, respectively. That is, the current LIGA method allows accurate manufacturing of nozzle diameters and guarantees accurate dpa50 values when the jet-to-plate distance is fixed. With new stage design, measurement and adjustment of the jet-to-plate distance becomes even easier than the exiting micro-orifice cascade impactors.
4. CONCLUSION
This study redesigned the NMCI of Liu et al. Citation(2013) to enable measurement and adjustment of the jet-to-plate distance of the impactors by using a micrometer. Differing from the MOUDI, each stage of the NMCI contains an impaction plate and a nozzle plate for itself. The bottom casing for impaction plate assembly and the nozzle plate holder of each stage are separated. The distance from the top of upper casing to the impaction substrate and the nozzle plate can be measured by using a micrometer for the determination of the jet-to-plate distance. The nozzle plates of Liu et al. Citation(2013) were used for the four lower stages to aviod possible nozzle clogging. To facilitate quick determination of nozzle diameter, the relationship between the nozzle diameter and pressure drop of the micro-orifice impactors for at the fixed operational flow rate of 30 L/min was established for estimating nozzle size from the pressure drop measurement. However, Equations (9) and (10) were only valid for the micro-orifice impactors with smooth nozzle shape manufactured by the LIGA method. Further correction for the vena contracta effect needs to be investigated to account for different nozzle contours.
Calibration results show that after adjusting the S/W ratios to proper values, the dpa50 of the 7th–10th stages of the NMCI are very close to the nominal values given in Marple et al. Citation(1991). The effect of the S/W ratio on the cutoff characteristics of the micro-orifice impactors was also investigated. When , (Stk50)1/2 increases gradually with an increasing S/W. This finding is different from the traditional theory for the single-nozzle impactors (Marple and Liu Citation1974; Radar and Marple Citation1985). If the size distribution of nanoparticles smaller than 56 nm is to be determined by using the instrument such as the 13-stage MOUDI-II (MSP Model 122 or 125), the S/W effects on the cutoff characteristics should be considered to aviod possible shift of dpa50. In addition, it is also suggested that regular examination on the thickness of the Teflon spacer and the jet-to-plate distance of the lower stages of the NMCI and the MOUDI is necessary to increase the accuracy of sampling. When operating the NMCI and the MOUDI, the pressure drop of the lower stages is found to begin to rise while the flow rate remains constant. This indicates that the nozzles in the final stages are becoming partially clogged due to particle deposition (Ji et al. Citation2006). When this occurs, it is time for the nozzle plates of the lower stages to be cleaned.
An empirical equation valid for was derived by fitting the experimental data for
to describe the correlation between the dimensionless cutoff size and the S/W ratio when considering isentropic flow. The equation can be used to facilitate the design of the micro-orifice impactors with smooth nozzle shape. Further study for other nozzle designs with different profiles to extend the applicable range of Re is necessary. A better understanding of the performance of multinozzle impactor operating at compressible flow conditions is also worth further investigating in the future as well. This study showed that the current LIGA method allows accurate manufacturing of nozzle diameters and guarantees accurate dpa50 values when the jet-to-plate distance is fixed. It is expected that the present NMCI could improve the accuracy of size-classification of submicron particles below 180 nm. Future work should be conducted to explore the interaction between the flows and the orifices by employing the numerical method to simulate the lower stages of the NMCI under practical working conditions in the compressible flow regime.
SUPPLEMENTAL MATERIAL
Supplemental data for this article can be accessed on the publisher's website.
UAST_1089976_Supplemental_File.zip
Download Zip (149.9 KB)FUNDING
The financial support of the Taiwan Ministry of Science and Technology (NSC 102-2221-E-009-008-MY3) is gratefully acknowledged.
REFERENCES
- Arffman, A., Marjamäki, M., and Keskinen, J. (2011). Simulation of Low Pressure Impactor Collection Efficiency Curves. J. Aerosol Sci., 42:329–340.
- Berner, A., Lürzer, C., Pohl, F., Preining, O., and Wagner, P. (1979). The Size Distribution of the Urban Aerosol in Vienna. Sci. Total Environ., 13:245–261.
- Biswas, P., and Flagan, R. C. (1984). High-velocity Inertial Impactors. Environ. Sci. Technol., 18:611–616.
- Canepari, S., Padella, F., Astolfi, M. L., Marconi, E., and Perrino, C. (2013). Elemental Concentration in Atmospheric Particulate Matter: Estimation of Nanoparticle Contribution. Aerosol Air Qual. Res., 13:1619–1629.
- Chang, M., Kim, S., and Sioutas, C. (1999). Experimental Studies on Particle Impaction and Bounce: Effects of Substrate Design and Material. Atmos. Environ., 33:2313–2322.
- Chaun, R. L. (1970). An Instrument for the Direct Measurement of Particulate Mass. J. Aerosol Sci., 1:111–114.
- Chen, S. C., Tsai, C. J., Chou, C. C. K., Roam, G. D., Cheng, S. S., and Wang, Y. N. (2010). Ultrafine Particles at Three Different Sampling Locations in Taiwan. Atmos. Environ., 44:533–540.
- Chen, S. C., Tsai, C. J., Chen, H. D., Huang, C. Y., and Roam, G. D. (2011). The Influence of Relative Humidity on Nanoparticle Concentration and Particle Mass Distribution Measurements by the MOUDI. Aerosol Sci. Technol., 45:596–603.
- Chen, S. C., Hsu, S. C., Tsai, C. J., Chou, C. C. K., Lin, N. H., Lee, C. T., Roam, G. D., and Pui, D. Y. H. (2013). Dynamic Variations of Ultrafine, Fine and Coarse Particles at the Lu-Lin Background Site in East Asia. Atmos. Environ., 78:154–162.
- Chow, J. C., and Watson, J. G. (2007). Review of Measurement Methods and Compositions for Ultrafine Particles. Aerosol Air Qual. Res., 7:121–173.
- Fang, C. P., Marple, V. A., and Rubow, K. L. (1991). Influence of Cross-flow on Particle Collection Characteristics of Multi-Nozzle Impactors. J. Aerosol Sci., 22:403–415.
- Fang, G. C., Chang, C. Y., Tsai, J. H., and Lin, C. C. (2014). The Size Distributions of Ambient Air Metallic Pollutants by Using a Multi-Stage MOUDI Sampler. Aerosol Air Qual. Res., 14:970–980.
- Flagan, R. C. (1982). Compressible Flow Inertial Impactors. J. Colloid Interf. Sci., 87:291–299.
- Fried, E., and Idelchik, I. E. (1989). Flow Resistance: A Design Guide for Engineers, Hemisphere Publishing Corp., New York.
- Gudmundsson, A., Bohgard, M., and Hansson, H. C. (1995). Characteristics of Multi-Nozzle Impactors with 50 μm Laser-Drilled Nozzles. J. Aerosol Sci., 26:915–931.
- Gulijk, C. V., Marijnissen, J. C. M., Makkee, M., and Moulijn, J. A. (2003). Oil-Soaked Sintered Impactors for the ELPI in Diesel Particulate Measurements. J. Aerosol Sci., 34:635–640.
- Hering, S. V. (1987). Calibration of the QCM Impactor for Stratospheric Sampling. Aerosol Sci. Technol., 7:257–274.
- Hillamo, R. E., and Kauppinen, E. I. (1991). On the Performance of the Berner Low Pressure Impactor. Aerosol Sci. Technol., 14:33–47.
- Huang, C. H., and Tsai, C. J. (2001). Effect of Gravity on Particle Collection Efficiency of Inertial Impactors. J. Aerosol Sci., 32:375–387.
- Huang, C. H., Tsai, C. J., and Shih, T. S. (2001). Particle Collection Efficiency of an Impactor with Porous Metal Substrates. J. Aerosol Sci., 32:1035–1044.
- Huang, C. H., and Tsai, C. J. (2002). Influence of Impaction Plate Diameter and Particle Density on Collection Efficiency of Round-Nozzle Inertial Impactors. Aerosol Sci. Technol., 36:714–720.
- Huang, C. H., and Tsai, C. J. (2003). Mechanism of Particle Impaction and Filtration by the Porous Metal Substrate of an Inertial Impactor. Aerosol Sci. Technol., 37:486–493.
- Huang, C. H., Chang, C. S., Chang, S. H., Tsai, C. J., Shih, T. S., and Tang, D. T. (2005). Use of Porous Foam as the Substrate of an Impactor for Respirable Aerosol Sampling. J. Aerosol Sci., 36:1373–1386.
- Ji, J. H., Bae, G. N., and Hwang, J. (2006). Observation and Evaluation of Nozzle Clogging in a Micro-Orifice Impactor Used for Atmospheric Aerosol Sampling. Particul. Sci. Technol., 24:85–96.
- Keskinen, J., Pietarinen, K., and Lehtimaki, M. (1992). Electrical Low Pressure Impactor. J. Aerosol Sci., 23:353–360.
- Kim, P. R., Han, Y. J., Holsen, T. M., and Yi, S. M. (2012). Atmospheric Particulate Mercury: Concentrations and Size Distributions. Atmos. Environ., 61:94–102.
- Kudo, S., Sekiguchi, K., Kim, K. H., Kinoshita, M., Möller, D., Wang, Q., Yoshikado, H., and Sakamoto, K. (2012). Differences of Chemical Species and Their Ratios Between Fine and Ultrafine Particles in the Roadside Environment. Atmos. Environ., 62:172–179.
- Kwon, S. B., Kim, M. C., and Lee, K. W. (2002). Effects of Jet Configuration on the Performance of Multi-Nozzle Impactors. J. Aerosol Sci., 33:859–869.
- Li, Y. P., Zhang, H. F., Qiu, X. H., Zhang, Y. R., and Wang, H. R. (2013). Dispersion and Risk Assessment of Bacterial Aerosols Emitted from Rotating-Brush Aerator During Summer in a Wastewater Treatment Plant of Xi'an, China. Aerosol Air Qual. Res., 13:1807–1814.
- Lin, C. C., Huang, K. L., Chen, H. L., Tsai, J. H., Chiu, Y. P., Lee, J. T., and Chen, S. J. (2014). Influences of Beehive Firework Displays on Ambient Fine Particles During the Lantern Festival in the YanShuei Area of Southern Taiwan. Aerosol Air Qual. Res., 14:1998–2009.
- Liu, C. N., Chen, S. C., and Tsai, C. J. (2011). A Novel Multi-Filter PM10–PM2.5 Sampler (MFPPS). Aerosol Sci. Technol., 45:1480–1487.
- Liu, C. N., Awasthi, A., Hung, Y. H., and Tsai, C. J. (2013). Collection Efficiency and Interstage Loss of Nanoparticles in Micro-Orifice-Based Cascade Impactors. Atmos. Environ., 69:325–333.
- Marple, V. A. (1970). A Fundamental Study of Inertial Impactors. Ph.D. thesis, Univ. of Minnesota, MN.
- Marple, V. A., and Liu, B. Y. H. (1974). Characteristics of Laminar Jet Impactors. Environ. Sci. Technol., 8:648–654.
- Marple, V. A., and Willeke, K. (1976). Impactor Design. Atmos. Environ., 10:891–896.
- Marple, V. A., Rubow, K. L., and Behm, S. M. (1991). A Microorifice Uniform Deposit Impactor (MOUDI): Description, Calibration and Use. Aerosol Sci. Technol., 14:434–446.
- Marple, V. A.. and Olson, B. A. (2011). Sampling and Measurement Using Inertial, Gravitational, Centrifugal and Thermal Techniques, Chapter 8, Aerosol Measurement: Principles, Techniques and Applications, Third Edition, P. Kulkarni, P. A. Baron, and K. Willeke, eds., John Wiley & Sons, Inc., Hoboken, NJ.
- Marple, V., Olson, B., Romay, F., Hudak, G., Geerts, M. S., and Lundgren, D. (2014). Second Generation Micro-Orifice Uniform Deposit Impactor, 120 MOUDI-II: Design, Evaluation, and Application to Long-Term Ambient Sampling. Aerosol Sci. Technol., 48:427–433.
- Pak, S. S., Liu, B. Y. H., and Rubow, K. L. (1992). Effect of Coating Thickness on Particle Bounce in Inertial Impactors. Aerosol Sci. Technol., 16:141–150.
- Peters, T. M., Vanderpool, R. W., and Wiener, R. W. (2001). Design and Calibration of the EPA PM2.5 Well Impactor Ninety-Six (WINS). Aerosol Sci. Technol., 34:389–397.
- Rader, J. D., and Marple, V. A. (1985). Effect of Ultra-Stokesian Drag and Particle Interception on Impaction Characteristics. Aerosol Sci. Technol., 4:141–156.
- Reischl, G. P., and John, W. (1978). The Collection Efficiency of Impaction Surfaces: A New Impaction Surface. Staub-Reinhalt. Luft, 38:55–58.
- Tsai, C. J., and Cheng, Y. H. (1995). Solid Particle Collection Characteristics on Impaction Surfaces of Different Designs. Aerosol Sci. Technol., 23:96–106.
- Tsai, C. J., Liu, C. N., Hung, S. M., Chen, S. C., Uang, S. N., Cheng, Y. S., and Zhou, Y. (2012). Novel Active Personal Nanoparticle Sampler for the Exposure Assessment of Nanoparticles in Workplaces. Environ. Sci. Technol., 46:4546–4552.
- Turner, J. R., and Hering, S. V. (1987). Greased and Oiled Substrates as Bounce-Free Impaction Surfaces. J. Aerosol Sci., 18:215–224.
- Valiliou, J. G., Sorensen, D., and McMurry, P. H. (1999). Sampling at Controlled Relative Humidity with a Cascade Impactor. Atmos. Environ., 33:1049–1056.
- Wang, H. C., and John, W. (1988). Characteristics of the Berner Impactor for Sampling Inorganic Ions. Aerosol Sci. Technol., 8:157–172.
- Yao, M., and Mainelis, G. (2006). Investigation of Cut-off Sizes and Collection Efficiencies of Portable Microbial Samplers. Aerosol Sci. Technol., 40:595–606.
- Zhu, C. S., Chen, C. C., Cao, J. J., Tsai, C. J., Chou, C. C. K., Liu, S. C., and Roam, G. D. (2010). Characterization of Carbon Fractions for Atmospheric Fine Particles and Nanoparticles in a Highway Tunnel. Atmos. Environ., 44:2668–2673.
- Zhu, C. S., Tsai, C. J., Chen, S. C., Cao, J. J., and Roam, G. D. (2012). Positive Sampling Artifacts of Organic Carbon Fractions for Fine Particles and Nanoparticles in a Tunnel Environment. Atmos. Environ., 54:225–230.