EDITOR Jian Wang
1. Introduction
The Differential Mobility Analyzer (DMA) is the most commonly used instrument for sizing sub-micron particles suspended in gases. Numerous design variations, each of which aims toward optimizing a certain aspect of its performance such as the size range and resolution, have been proposed since the DMA was first introduced in the mid-70s (e.g., Zhang et al. Citation1995; Rossell-Lompart et al. Citation1996; de Juan and de la Mora Citation1998; Giamarelou et al. Citation2011). Despite the wide variety of DMA designs, however, little attention has been paid to other practical aspects such as their weight and manufacturing cost.
The weight of conventional DMAs is in the order of a few kg, limiting their portability and use in nanoparticle exposure studies or in airborne measurements using unmanned aerial vehicles (UAVs). What is more, their manufacturing cost is in the order of several thousand USD, which is significant, especially when a number of DMAs is needed for distributed measurements of atmospheric aerosols. Although recent efforts have led to simple and inexpensive tools for sizing aerosol particles (Bezantakos et al. Citation2015), using a DMA offers many advantages. An effort to reduce the manufacturing cost of DMAs was made by Mei et al. Citation(2011) who designed and built a DMA consisting of fewer parts. By doing so, they managed to produce a DMA that costs less than 50% to build compared to the classical design, and weighs ca. 350 g (D. R. Chen Citation2015, personal communication).
Existing DMAs are exclusively manufactured out of metal due to the necessary electrical conductivity for establishing a well-defined electric field. Using metals as building materials, however, is the very reason of their high weight and manufacturing cost. The possibility of manufacturing DMAs out of polymers, therefore, would be highly advantageous since many simple and inexpensive techniques (e.g., room temperature molding and three-dimensional (3D) printing) are available. In addition, avoiding using metals would allow one to choose from a vast number of materials with significantly lower density, thereby reducing instrument weight.
Here we describe an alternative method for manufacturing DMAs using low-cost and low-density polymers to build their body, and metal coatings for providing conductivity only where needed (i.e., on the inner DMA surface). More specifically, we have built a DMA using polyurethane and spray-coated the inner surface with a 0.5-mm thick conductive Nickel film. The weight of the polyurethane DMA (referred to as the PU-DMA from this point onward) is 95% lower compared to that of its metallic counterpart, while its cost is also reduced substantially. The performance of the PU-DMA is tested in comparison to that of an identical stainless-steel DMA (SS-DMA).
2. Manufacturing
The design of the PU-DMA developed in this work was based on a custom-made cylindrical SS-DMA consisting of five metallic parts (i.e., the inner and outer electrode, the aerosol entrance slit, and the inlet and outlet base; see Figure S1). To create these parts out of polyurethane we used a mold-casting process. The first step of the process was to create a mold for each part of the SS-DMA using flexible silicone rubber (see the online supplemental information [SI]). To do so, each piece was placed inside a container and liquid silicone rubber was poured until fully submerged. The metallic pieces were removed from their molds after ca. 1 h that was required for the silicone to harden. Liquid polyurethane was then poured into the mold and allowed to solidify for ca. 1 h.
In order to make the inner surfaces of the PU-DMA (i.e., those coming in contact with the sampled aerosols as indicated in Figure S1) conductive, we spray-coated them with a nickel film and allowed to dry. Three cycles of a spraying/drying process were sufficient to achieve full coverage of the surface, yielding a 0.5-mm thick conductive film on the surface of each part, as determined by profileometry. As a result of the thickness of the conductive film, the gap between the two electrodes in the PU-DMA is reduced by 1 mm compared to the SS-DMA. The geometric dimensions of both DMAs used in this work are summarized in Table S1.
The weight of the resulting PU-DMA is 150 g, which is significantly lower than that of the SS-DMA (3.5 kg), while the cost of the materials used for manufacturing is ca. 100 USD. Considering that multiple DMAs can be produced from a single mold, the associated cost can be further reduced, making the proposed method highly attractive.
3. Characterization
The performance of the two DMAs was initially compared by using them as part of a differential mobility particle sizer (DMPS) for measuring the particle size distribution of a stable laboratory-generated polydisperse aerosol (see route 1 in Figure S2 and associated discussion in the SI). Measurements were performed at aerosol to sheath flow rate ratios β of 1.5/5.0, 1.5/7.0, and 1.5/10.0.
The midpoint particle electrical mobility corresponding to each potential difference applied between the two electrodes of the test DMAs was calculated as (Knutson and Whitby 1975):[1] where Qsh is the sheath flow rate, V is the potential difference between the two DMA electrodes, L is the distance between the polydisperse aerosol inlet and the monodisperse aerosol outlet slit, while R1 and R2 are the inner and outer electrode radii, respectively. By definition, the electrical mobility of the particles is given by (Hinds Citation1999):
[2]
Here n is the number of elementary charges, e is the electron charge, η is the gas viscosity, dm is the particle diameter, and Cc is the Cunningham slip correction factor given by:[3] where λ is the gas mean free path. In all cases, we assume that the particles carry one elementary charge. The size of the particles classified by the DMA for every set of operating conditions (i.e., potential difference between the electrodes and sheath flow rate) can be estimated by equating Equations (Equation1
[1] ) and (Equation2
[2] ) and solving iteratively.
The transfer functions of both DMAs (i.e., the SS- and the PU-DMA) were measured with a tandem DMA configuration (Rader and McMurry Citation1986). Nearly monodisperse particles were produced by a glowing wire (GW) generator in series with a long SS-DMA (LSS-DMA; see route 2 in Figure S2). The transfer function of the tested DMA was determined by stepping their voltage across the mobility range of the monodisperse particles produced by the GW generator and the LSS-DMA. The measured signal of a tandem DMA system expressed as a ratio of the concentrations of particles at the polydisperse aerosol inlet N1 and at the monodisperse aerosol outlet N2 of the test DMA, can be determined by:[4]
Here Ω1 and Ω2 are the transfer functions corresponding to the LSS-DMA and the test DMA, respectively, whereas is the midpoint electrical mobility (see Equation (Equation1
[1] )) of the monodisperse aerosol particles downstream the LSS-DMA. The key parameters defining the transfer functions of the test DMAs were determined using the tandem DMA measurements and the deconvolution scheme described by Stratmann et al. Citation(1997).
4. Results and discussion
shows the normalized concentration of the charged tungsten particles (produced by the GW generator) measured by the PU- or the SS-DMA when set at different voltages and at β = 1.5/5.0. Log-normal curves were fitted to the data using a nonlinear least-square method. The mean particle size determined by the measurements using the PU-DMA was in excellent agreement with those using the SS-DMA. The total number concentration measured by the PU-DMA, however, was found to be 68% lower compared to that measured by the SS-DMA.
Figure 1. Size distribution measurements of polydisperse charged tungsten aerosol nanoparticles measured using either the PU-DMA (circles) or the SS-DMA (squares) at an aerosol to sheath flow ratio of 1.5/5.0.
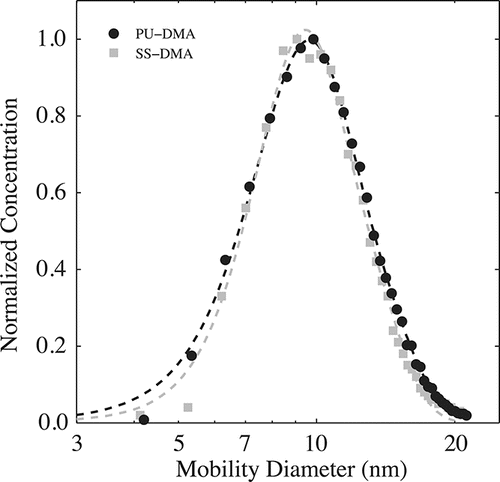
When the sheath flow rate through the two DMAs was increased to 7.0 (β = 1.5/7.0) and 10.0 lpm (β = 1.5/10.0), the difference between the mean particle mobility diameter measured by the two classifiers increased to 2.9 and 8.9%, respectively (see Figure S3). We attribute this difference to structural non-idealities of the PU-DMA, which can cause small flow disturbances close to the electrode walls and thus to the trajectories of the particles as the flow rate through the classifier increases. Such non-idealities can originate from surface defects (e.g., variability of the coating thickness), or from misalignment of the inner electrode, which in turn can cause non-idealities in the electric and flow fields that would require numerical models to evaluate them.
compares measured and predicted (using Equation (Equation4[4] )) N2/N1 ratios for 10-nm monodisperse particles when either the PU- or the SS-DMA were used in the tandem DMA system. The height and full width at half maximum (FWHM) of the transfer functions of both DMAs determined by these measurements and the deconvolution scheme described by Stratmann et al. Citation(1997) are shown in Figure S4. Although the height and FWHM of the transfer functions of both DMAs as a function of particle size follow the same trend with the theory, the PU-DMA exhibits larger deviations compared to the SS-DMA. These deviations can be explained by small geometrical differences between the two DMAs that cannot be captured by Equation (Equation4
[4] ). The 0.5-mm thick coating, for instance, reduces the gap of every channel in the DMA, with the most sever being that of the aerosol inlet slit, which narrows down to half the size of that of the SS-DMA. This can lead to pronounced aerosol recirculation affecting the performance of the PU-DMA (Chen et al. Citation1999). To fully capture that, however, a particle-trajectory model would be required, which is out of the scope of the current study.
Figure 2. Predicted (line) and measured response of the tandem DMA system using either the PU-DMA (circles) or the SS-DMA (squares) determined with 10-nm particles. Inset shows the correlation between measured and calculated midpoint mobility diameter for both DMAs.
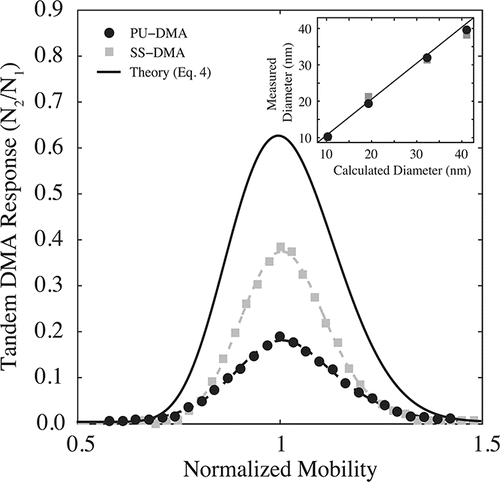
The inset of shows the mean mobility diameter measured by the test DMAs versus the midpoint mobility diameter calculated by Equations (Equation1[1] ) and (Equation2
[2] ) using the dimensions provided in Table S2. Evidently agreement for these specific operating conditions (β = 1.5/5.0) is well within a 3% experimental uncertainty that is typical for DMAs (Kinney et al. Citation1991), showing that the PU-DMA performs equally well compared to the SS-DMA in this respect. Repeated measurements over a period of 6 months showed similar performance, indicating that the PU-DMA is also durable.
5. Conclusions
In this work, we introduce a new method for manufacturing DMAs with extremely small weight and low manufacturing cost. We do so by avoiding using metals as building material, which has been the common practice thus far. Instead, we use parts made of a polymer that are coated with a conductive film. We prove this concept by constructing a DMA out of polyurethane, achieving more than 95% reduction in weight and a significant decrease in manufacturing cost. The performance of this DMA (i.e., the PU-DMA) was evaluated in comparison to an identical metallic DMA made of stainless steel (i.e., the SS-DMA). The measurements performed with the PU-DMA show good overall agreement with the ones performed with the SS-DMA, providing confidence in using it for routine particle mobility measurements. Differences between the measured mean size of particles from a polydisperse aerosol using the PU- and the SS-DMA was within experimental uncertainty when low sheath flow rate (i.e., 5.0 lpm) was used through the classifiers, but increased up to 8.9% for higher flows. These quantitative differences are attributed to manufacturing uncertainties of the PU-DMA.
Despite these differences, the performance of the PU-DMA manufactured with the method described in this work is encouraging, providing a first step toward the development of cost-effective lightweight instruments. An upgrade of this method, in terms of manufacturing precision, can involve the use of 3D printing technology for building DMA bodies out of a number of polymers. Doing so will offer the possibility of improving the accuracy and precision of the classifiers and further reducing their production costs.
UAST_1130216_Supplementary_File.zip
Download Zip (704.6 KB)Funding
This work is supported by NanoNextNL, a micro and nanotechnology consortium of the Government of the Netherlands and 130 partners.
References
- Bezantakos, S., Huang, L., Barmpounis, K., Attoui, M., Schmidt-Ott, A., and Biskos, G. (2015). A Cost-Effective Electrostatic Precipitator for Aerosol Nanoparticle Segregation. Aerosol Sci. Technol., 49:IV–VI.
- de Juan, L., and de la Mora, J. F. (1998). High Resolution Size Analysis of Nanoparticles and Ions: Running a Vienna DMA of Near Optimal Length at Reynolds Numbers up to 5000. J. Aerosol Sci., 29:617–626.
- Chen, D.-R., Pui, D. Y. H., Mulholland, G. W., and Fernandez. M., (1999). Design and Testing of an Aerosol/Sheath Inlet for High Resolution Measurements with a DMA. J. Aerosol Sci., 30(8):983–999.
- Giamarelou, M., Stolzenburg, M., and Biskos, G. (2011). The Multiple Monodisperse Outlet Differential Mobility Analyzer: Derivation of Its Transfer Function and Resolution. Aerosol Sci. Technol., 46:951–965.
- Hinds, W. C. (1999). Aerosol Technology: Properties, Behavior, and Measurement of Airborne Particles, 2nd Ed. John Wiley & Sons, Inc., New York.
- Kinney, P. K., Pui, D. Y. H., Mulholland, G. W., and Bryner, N. P. (1991). Use of the Electrostatic Classification Method to Size 0.1-µm SRM Particles—A Feasibility Study. J. Res. Natl. Inst. Stand. Technol., 96(2):147–176.
- Knutson, E. O., and Whitby, K. T. (1975). Aerosol Classification by Electric Mobility: Apparatus, Theory, and Applications. J. Aerosol Sci., 6:443–451.
- Mei, F., Huijing, F., and Chen, D. R. (2011). A Cost-Effective Differential Mobility Analyzer (cDMA) for Multiple DMA Column Applications. J. Aerosol Sci., 42:462–473.
- Rader, D. J., and McMurry, P. H. (1986). Application of the Tandem Differential Mobility Analyzer to Studies of Droplet Growth or Evaporation. J. Aerosol Sci., 17:771–787.
- Rossell-Lompart, J., Loscertales, T. G., Bingham, D., and de la Mora, J. F. (1996). Sizing Nanoparticles and Ions with a Short Differential Mobility Analyzer. J. Aerosol Sci., 27:695–720.
- Stratmann, F., Kauffeldt, Th., Hummes, D., and Fissan, H. (1997). Differential Electrical Mobility Analysis: A Theoretical Study. Aerosol Sci. Technol., 26:368–383.
- Zhang, S.-H., Aksutsu, Y., Russel, L. M., Flagan, R. C., and Seinfeld, J. H. (1995). Radial Differential Mobility Analyzer. Aerosol Sci. Technol., 23:357–372.