ABSTRACT
Sampling and dilution of flame-generated, fractal-like ZrO2 aerosols is investigated by aerosol mass/mobility measurements and microscopy. Two broadly used sampler configurations, a straight-tube (ST) and a hole-in-a-tube (HiaT), at three different in-flow orientations and hole diameters are evaluated. The mobility size distributions, mass-mobility exponent, Dfm, prefactor, kfm, and average primary particle diameter are obtained at 10–60 cm height above the burner (HAB) of fuel-rich (hot) and fuel-lean (cold) spray flames by differential mobility analyzer (DMA) and aerosol particle mass (APM) measurements using a recent power law for fractal-like particles. The primary particle diameter, agglomerate size distributions, and corresponding standard deviations from aerosol measurements are compared to those by counting images of particles collected by thermophoretic sampling along the flame centerline. Once new particle formation is completed in the flame, both sampler configurations result in nearly identical particle size distributions. Furthermore, all HiaT samplers result in similar mobility size distributions at all orientations, regardless of hole size. Sampling using a downstream in-flow hole orientation results in slightly larger Sauter mean diameters than those obtained by upstream or sidestream ones, especially for the cold flame. Additionally, a correlation is developed by Discrete Element Modeling (DEM) for the agglomerate Dfm evolution to its asymptotic value of 2.2 as function of the average number of primary particles per agglomerate, nva, or the relative particle density with pre-exponential constant kfm = 1.18, regardless of primary particle size. This is in good agreement with an experimentally obtained correlation in terms of relative particle density as well as with experimental data for ZrO2, Ag, and Cu nanoparticles.
© 2016 American Association for Aerosol Research
EDITOR:
1. Introduction
Aerosol particle formation in the atmosphere as well as in flame and hot-wall reactors frequently results in fractal-like aggregates or agglomerates, with constituent particles held together by chemical or physical forces, respectively. Depending on their number concentration, such aerosols can impact health (Oberdörster et al. Citation1995) and environment as they exhibit negative radiative forcing (Haywood and Boucher Citation2000). Furthermore, they are encountered in aerosol manufacture of nanostructured commodities and specialty products (Strobel and Pratsinis Citation2007).
Online and real-time monitoring of fractal-like aerosols is important for controlling their synthesis and quantifying their impact. Aerosol characteristics often change during sampling by diffusion, coagulation, and/or fragmentation (Maricq Citation2004). In addition, perturbation of aerosol generation by the sampler (as with any intrusive sampling or diluting probe) can distort the measured particle concentration (Kasper et al. Citation1997), as well as shape and spread of size distribution.
Ulrich et al. (Citation1976) extracted and rapidly diluted SiO2 aerosols from a turbulent flame by a straight-tube (ST) sampler. Kasper et al. (Citation1997) extracted and rapidly diluted Pt, Pd, and soot nanoparticles from a laminar diffusion flame with a ST sampler at different heights above the burner (HAB) and found no major contribution of homogeneous nucleation in the actual particle size distribution by sampling artifacts. Maricq (Citation2004) compared mobility size distributions using a hole-in-a-tube (HiaT) sampler (Zhao et al. Citation2003) and an angled ST probe (Maricq et al. Citation2003) for sampling and diluting soot nanoparticles from premixed ethylene flames. He found that the flame perturbation is modest and does not qualitatively affect the soot size distribution even though the temperature significantly decreases by the sampler presence (Zhao et al. Citation2003) as confirmed by De Filippo et al. (Citation2009).
Particle size and morphology or structure affect particle properties and performance. Eggersdorfer et al. (Citation2012a) used an ST sampler to distinguish flame conditions for synthesis of aggregates and agglomerates by power laws and mass/mobility measurements downstream of the flame. Stein et al. (Citation2013) characterized online Cu nanoparticles by combining a scanning mobility particle sizer (SMPS) and an electrical low pressure impactor (ELPI) using an ejector (ST-like) sampler and proposed an expression that relates the mass-mobility exponent with the relative particle density. Gröhn et al. (Citation2014a) investigated the detailed axial and radial evolution of flame-made zirconia primary and agglomerate particle diameters and associate standard deviations using a HiaT sampler.
Here the effect of ST and HiaT sampler designs and operations on the characteristics of highly concentrated flame-made ZrO2 nanoparticles at different formation and growth stages is elucidated. Measurements of aerosol size distributions by differential mobility analyzer (DMA) are compared to scanning transmission electron microscopy (STEM) images obtained by thermophoretic sampling at the flame centerline. Combined DMA and aerosol particle mass (APM) measurements are used to determine the mass-mobility exponent, Dfm, and average primary particle diameter by a power law correlation that is independent of material composition and polydispersity (Eggersdorfer and Pratsinis Citation2013). The results are compared to Dfm obtained by discrete element method (DEM) simulations and experimental measurements for Ag (Kim et al. Citation2009), ZrO2 (Eggersdorfer et al. Citation2012a) and Cu (Stein et al. Citation2013). The evolution of particle Dfm from spherical to fractal-like structures is obtained by DEM, for the first time, and quantified by two easy-to-use expressions in terms of the number of primary particles per agglomerate and the relative particle density that is compared to an experimentally obtained one (Stein et al. Citation2013).
2. Experimental
2.1. Nanoparticle synthesis
Zirconia nanoparticles are generated by flame spray pyrolysis (FSP: Mädler et al. Citation2002) of a precursor solution consisting of 1 M zirconium 2-ethylhexanoate (Zr-2EHA Valirex, 18 wt% Zr) dissolved in xylene (253-VL51TE; Thommen-Furler AG, Büren, Switzerland). So 5 (long, hot flame) or 2 mL/min (short, cold flame) of that solution are supplied with a continuous pump (MRZ-2905, HNP Mikrosysteme GmbH, Schwerin, Switzerland) through the center capillary of the FSP nozzle and atomized with 2 and 5 L/min, respectively, of dispersion O2 (99.95%, PanGas, Dagmersellen, Switzerland) by mass flow controllers at 2 bar pressure drop (Eggersdorfer et al. Citation2012a). The gas flows of the supporting flame are set to 1.25 L/min CH4 and 2.5 L/min O2 by mass flow controllers (EL-flow, Bornkhorst, Reinach, Switerland). The two flame temperatures are too different (see also Figure S1 in the online supplementary information (SI)) representing two extreme conditions of typical FSP synthesis. For example, at HAB = 10 cm the temperature difference between the two flames is ΔT = 837°C, which results in significantly different particle growth dynamics. Even a temperature difference of about 300°C can result in different particle characteristics, such as primary particle and agglomerate collision diameters and effective volume fraction, as has been shown by Heine and Pratsinis (2006: figures 1 and 3).
2.2. Aerosol sampling
shows the experimental setup and online diagnostics of flame-made aerosols and the ST (bottom) and HiaT (top) samplers in upstream orientation (in-flow hole is pointing toward the burner). Quenching and sampling of hot, highly concentrated ZrO2 nanoparticles was carried out at cross-flow to the flame centerline at 10–60 cm HAB by three stainless steel HiaT samplers of 6 mm inner tube diameter with 1, 2, or 4 mm in-flow hole diameter and an ST sampler with in-flow tube inner diameter of 2.5 mm becoming 5 mm after a 15 mm long entrance (). The samplers’ in-flow hole was oriented upstream (), sidestream (hole is rotated by 90° with respect to flame centerline), and downstream (rotated by 180°). The sample stream is mixed with the diluent flow resulting in a Reynolds number of about 260–3000 for the HiaT and 560–3100 for the body of the ST sampler (). The aerosol is cooled down upon entering each sampler resulting in reduced thermophoretic wall losses (Zhao et al. Citation2003) and slowing down to ceasing any ongoing reactions inside the samplers (Kasper et al. Citation1997). The total flow rate out of the first dilution stage (ST or HiaT sampler) is kept constant at Q = 14.6 L/min, regardless of sampler configuration, using a calibrated mass flow controller and a vacuum pump (Gröhn et al. Citation2014a). So the velocity, u, of the aerosol into a sampler is calculated by u = Q/A = 4Q/(πdS2), where A and ds are the cross-section area and diameter of the ST and HiaT samplers (). Therefore, at the entrance and body of the ST sampler the velocity is ∼50 and 12.4 m/s, respectively, while in all HiaT samplers it is ∼8.6 m/s. The velocity that the aerosol enters the sampler spans one order of magnitude and depends on the in-flow hole diameter.
Figure 1. Schematic of the experimental setup with diagnostics of flame-made aerosols and the hole-in-a-tube (HiaT, top) and straight-tube (ST, bottom) samplers in upstream orientation (hole is pointing toward the burner). HAB, height above the burner; DMA, differential mobility analyzer; APM, aerosol particle mass analyzer; CPC, condensation particle counter.
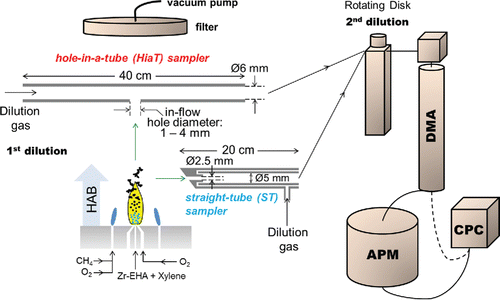
Subsequent to the dilution with particle-free compressed air in the above samplers (dilution ratio of 10–10,000), a second dilution takes place by a rotating disk diluter (MD 19-1E, Matter Engineering AG, dilution factor of ∼25) so that the particle number concentration is reduced to the operation limit of the aerosol sizing instruments while the particle number concentration in the samplers is in the range of 2.5·106–2.5·107 particles/cm3. The flow entering the rotation disk diluter (second dilution stage) is turbulent with Reynolds numbers up to Re ≈ 8500 while in the subsequent sampling lines and the aerosol instruments’ entrance the flow is laminar.
The diluted aerosol flows at 1.5 L/min to the DMA (TSI 3081; TSI Inc., Shoreview, MN, USA). Its charge distribution is equilibrated by a soft X-ray neutralizer (TSI 3087). Aerosols are size-classified with sheath flow ranging from 10 to 15 L/min. The aerosol stream flows from the DMA through insulation tubes (minimizing electrostatic deposition) and a stream of highly monodisperse particles (Ahonen et al. Citation2001; Kuga et al. Citation2001) is directed to a condensation particle counter (CPC, TSI 3775) either directly or through an aerosol particle mass analyzer (APM, APM-3600, Kanomax USA Inc., Andover, NJ, USA). In the DMA-CPC system (SMPS), the ZrO2 mobility size distribution is measured while in the DMA-APM-CPC system the average mass, m, is measured for mobility size-selected agglomerates (Eggersdorfer et al. Citation2012a).
2.3. Particle characterization
The product particle specific surface area, SSA, is determined by nitrogen adsorption (Micrometrics Tristar 3000; Micrometrics Tristar, Norcross, GA, USA). The corresponding average primary particle (PP) diameter is calculated for tetragonal zirconia (Mueller et al., Citation2004a) with ρb = 5720 kg/m3 (Whitney Citation1994) assuming monodisperse, spherical particles as dSSA = 6/(SSA· ρb). The crystal size is measured by X-ray diffraction (XRD) and Rietveld Analysis (Mueller et al. Citation2004a). Thermophoretic sampling (Dobbins and Megaridis Citation1987) is carried out along the flame centerline at HAB = 10–60 cm for both hot and cold flames using 40–100 ms grid residence time. The particle size and morphology are determined also by STEM (STEM HD 2700 CS, Hitachi High Technologies Europe GmbH, Krefeld, Germany). The STEM images are processed by a semi-automatic micrograph analysis program to obtain the Sauter mean PP and their corresponding number-based geometric standard deviation (Gröhn et al. Citation2012). Agglomerate mobility size distributions are obtained by SMPS while the PP diameter is determined by combining the SMPS and DMA-APM-CPC (Eggersdorfer et al. Citation2012a) data with a power law (Eggersdorfer and Pratsinis Citation2013) listed in theory below.
The particle structure is quantified by the mass-mobility exponent, Dfm, and prefactor, kfm, with a power law that scales agglomerate particle mass with its mobility diameter (Park et al. Citation2003). The Dfm and kfm of the gas-borne ZrO2 particles are obtained from the slope and intercept, respectively, in the log nva versus log (dm/dSSA) plot, where nva is the average number of PPs per agglomerate, dm the agglomerate mobility diameter, and dSSA the SSA-obtained PP diameter. The particle size distributions are corrected for diffusion losses (Gormley and Kennedy Citation1949) to the tube and manifold walls and are normalized with the total particle concentration.
3. Theory
3.1. Particle structure
The agglomerate dm scales with the particle mass (or number of PPs per agglomerate, nva) by Dfm (Park et al. Citation2003):[1] where dva and mva are the mean surface area-based PP diameter (or Sauter mean diameter) and mass, respectively. The asymptotic Dfm in the free molecular regime is 2.17 ± 0.10 (Sorensen Citation2011) while for large diffusion-limited cluster–cluster agglomeration (DLCA)-made particles (equivalent to continuum regime) of 20 nm PP diameter it is 2.15 ± 0.003 and kfm = 1.11 ± 0.01 (Eggersdorfer et al. Citation2012b). The Dfm can be related also to the effective particle density, ρeff, as (e.g., Wu and Friedlander Citation1993)
[2]
Stein et al. (Citation2013) showed that Dfm is related to agglomerate relative density, ρeff/ρb, obtained by ELPI and SMPS (Ristimäki et al. Citation2002) measurements by[3]
Eggersdorfer et al. (Citation2012a) estimated the dva of fractal-like particles based on ab initio calculations by[4] where kα and Dα are parameters that depend on agglomerate structure and υ is the agglomerate volume as obtained by APM measurements (υ = mAPM/ρb). Here kα = 1.0 and Dα = 1.07 are used for polydisperse aggregate PP diameters (Eggersdorfer and Pratsinis Citation2013). Equation (4) can be used to determine reliably the primary particle diameter, dva, of aggregates or agglomerates in the free molecular and transition regimes based only on online mass-mobility measurements.
3.2. Numerical implementation
Agglomerate formation by coagulation is investigated by discrete element modeling (DEM) in the absence of surface growth, sintering, or coalescence assuming that the clusters or agglomerates consist of spherical, monodisperse PPs in point contact (Goudeli et al. Citation2015). The particle trajectories are calculated (Pierce et al. Citation2006) in the free molecular, transition, and continuum regimes and rigorously validated as described in detail by Goudeli et al. (Citation2015). Particles collide and stick with unity sticking probability neglecting van der Waals, electric, and hydrodynamic interactions or particle rotation (Heine and Pratsinis Citation2007). The drag force of the resulting clusters is calculated using the Stokes friction coefficient with the Cunningham correction factor. The evolution of cluster size (nva, dm) is tracked down from spherical particles to fractal-like ones while kfm and Dfm are obtained by Equation (1).
3.3. DEM-derived evolution of mass-mobility exponent
shows the evolution of the DEM-derived agglomerate mass-mobility (a) exponent, Dfm, and (b) prefactor, kfm, of coagulating agglomerates in the gas-slip and continuum regimes as function of the average nva and (c) relative density for agglomerates of monodisperse PPs of radius, rp,0 = 30, 100, and 200 nm (average of five simulations for each rp,0). For all PP radii investigated here, little or no variation is observed in the corresponding agglomerate Dfm and kfm, as shown also for Df and pre-exponential prefactor, kn (Goudeli et al. Citation2015: figure 5). These clusters have PPs in point contact and tend to form when coagulation dominates over coalescence or sintering. Such conditions are encountered typically in the atmosphere as well as way downstream of flame, plasma, laser, and hot-wall reactors where low temperatures prevail.
Figure 2. Evolution of DEM-derived mass-mobility (a) exponent, Dfm, and (b) prefactor, kfm, of coagulating agglomerates as a function of the average number of primary particles per agglomerate, nva, and (c) as function of the relative density of agglomerates with primary particle radius rp,0 = 30, 100, and 200 nm. Error bars show the variation of five simulations for rp,0 = 100 nm. Initially, particles consist of a few primary particles (Dfm ≈ 3, kfm ≈ 1), but as they grow (nva > 10) they reach asymptotically Dfm = 2.2 and kfm = 1.18 ± 0.05. The Dfm evolution is consistent with the experimentally derived relative density correlation of Stein et al. (Citation2013, equation (3): dotted line).
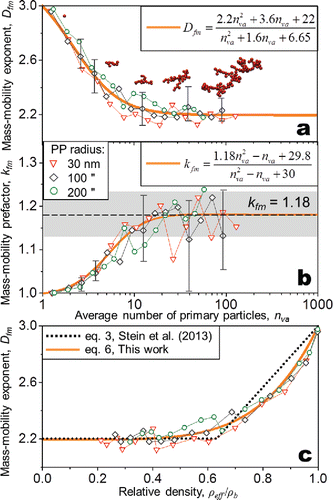
At any time, t, during coagulation–agglomeration, Dfm and kfm are calculated from Equation (1) by plotting nva versus dm/dva for all DEM-generated agglomerates. Initially, all particles are spheres and thus Dfm = 3 and kfm = 1. When agglomeration starts, particles grow so nva increases and Dfm decreases smoothly attaining an asymptotic value of ∼2.2 for nva > 10, consistent with Eggersdorfer et al. (Citation2012b). Such fractal-like particles are made by DLCA corresponding to agglomeration in the continuum regime and gradually evolve to their asymptotic fractal dimension Df = 1.78 ± 0.05 (Jullien et al. Citation1984) as was shown by Goudeli et al. (Citation2015) who proposed a simple expression for the evolution of Df and kn employing various PP sizes (1–500 nm). Here also the PP size does not affect the Dfm and kfm beyond their DEM variability. The Dfm and kfm evolutions from spheres to fractal-like particles are quantified from and b (solid lines) as[5a] and
[5b] which attain their asymptotic values of 2.2 and 1.18, respectively, for nva > 100, as the power law of Equation (1) is strictly valid for such large agglomerates (Forrest and Witten Citation1979). The kfm increases slightly with increasing nva up to about 10 and beyond that can be bracketed as kfm = 1.18 ± 0.05 (). These results are consistent with large DLCA-made agglomerates of dp = 20 nm where Dfm = 2.15 ± 0.003 and kfm = 1.11 ± 0.01 were obtained (Eggersdorfer et al. Citation2012b), as well as with DMA-APM-CPC measurements (see Figure S2 in the online SI). shows the above evolution of the Dfm from DEM (symbols) as function of relative agglomerate density, ρeff/ρb, quantified by the following expression (solid line),
[6] so that for large agglomerates for which ρeff/ρb → 0, Dfm = 2.2 and for compact particles (e.g., spheres) for which ρeff/ρb = 1, Dfm = 3. Equation (6) (: solid line) is obtained for unsintered agglomerates of monodisperse PPs and is in excellent agreement with the experimentally derived correlation of Stein et al. (Citation2013, equation (3): dotted line).
4. Results and discussion
4.1. Effect of sampler design on mobility size distribution
shows STEM images of thermophoretically sampled ZrO2 particles along the reactor centrerline at 10–60 cm HAB for (a) cold and (b) hot spray flames. At HAB = 10 cm, spherical particles and/or droplets are present especially at the long, hot, and fuel-rich flame () while small clusters have been formed already in the short, cold, and fuel-lean flame (). At this HAB, the temperature of the hot flame is 1340°C while in the cold flame it is much lower at 503°C (see Figure S1 in the online SI). With increasing HAB (left to right), the initially small and compact particles grow to fractal-like aggregates by coagulation. At the hot flame, agglomerates consist of larger (but fewer) PPs than the cold flame due to higher particle concentration and prolonged high temperature residence time (longer visible flame) that enhance particle sintering. Thermogravimetric analysis (TGA) coupled with mass spectrometry (MS) for the powder made in hot flame revealed that the product particles are carbon-free (see Figure S3 in the online SI), consistent with Mueller et al. (Citation2004b). Diluting the precursor concentration and increasing the dispersion gas flow rate (cold flame) decreases the high temperature particle residence time and thus the PP diameter, consistent with Gröhn et al. (Citation2014b). Here the Knudsen number, Kn (defined as Kn = 2λ/dm), ranges from 2.2 (HAB = 60 cm and cold flame: transition regime) to 30 (HAB = 10 cm and hot flame: free molecular regime) indicating a shift from ballistic to diffusion-limited coagulation.
Figure 3. STEM images of thermophoretically sampled ZrO2 particles along the reactor centerline at 10, 30, 40, 50, and 60 cm height above the burner (HAB) for (a) cold and (b) hot spray flames. Particles grow with increasing HAB forming aggregates that consist of larger primary particles (PPs) in the hot than in the cold flame.
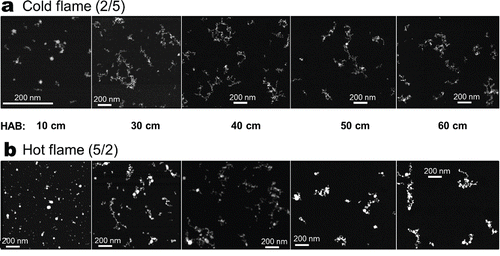
shows size distributions (SDs) of fractal-like particles made by (a) cold and (b) hot flames at HAB = 10–60 cm collected by the aerosol samplers (lines) as well as by thermophoretic sampling and counting STEM images (symbols). Two sampler designs are evaluated at upstream hole orientation (): three HiaT samplers with in-flow hole diameter of 1 (dot-broken, black lines), 2 (dotted, green lines), or 4 mm (broken, red lines) and one ST sampler (solid, blue lines). Samplers give similar mobility SDs and quite close to projected area-equivalent diameter, dpa, ones (obtained by counting particle images) except at the lowest HAB of the cold flame (: HAB = 10 cm) as DMA does not measure reliably particles smaller than 5 nm. It should be noted here that particles are small (dm < 1 μm) so isokinetic sampling takes place at all conditions (Stokes number, St << 0.01; see Equations (S1)–(S5) in the online SI). The shape of the STEM-obtained diameter of gyration-based, dgyr, SD is consistent with the dpa-based SD at all HAB; however, both SDs are broader with larger Sauter mean diameters ( and S1 [in the online SI]) than SMPS-obtained SDs, consistent with experiments (Gröhn et al. Citation2014a: figures 6 and 7) and simulations (Goudeli et al. Citation2015: figures 3 and 8). In addition, the large tail of the STEM-obtained SDs is shifted to larger particle sizes, especially at middle HAB (30–50 cm), due to particle recirculation that becomes important as the flame jet broadens. At all HAB investigated here (), coagulation by cluster–cluster agglomeration is the dominant mechanism (without concurrent chemical reaction, nucleation, or surface growth) and the role of the samplers is limited only in suppressing further coagulation. The mobility SDs by the HiaT and ST samplers are identical at all HAB except at HAB = 10 cm where the long tail of the SD by the ST sampler reveals slightly smaller particle sizes as it provides shorter aerosol residence times than the HiaT samplers. It should be noted that emitted particles from the stainless steel tube are too few (even at the hottest conditions, hot flame at HAB = 10 cm) compared to the measured ZrO2 nanoparticles and do not affect the SD measurements (see Figure S12 in the SI).
Table 1. Statistics of the STEM-obtained Sauter mean diameter of gyration and projected area-equivalent diameter and corresponding geometric standard deviations are shown for hot and cold flame conditions at HAB = 10–60 cm.
Figure 4. Size distributions (SDs) of fractal-like particles made by (a) cold and (b) hot spray flames sampled at HAB = 10–60 cm. Mobility SDs from four sampler configurations are shown: three HiaT samplers with hole diameter of 1 mm, dot-broken (black) lines, 2 mm, dotted (green) lines, and 4 mm, broken (red) lines, and one ST, solid (blue) lines. The mobility SDs are consistent with projected area-equivalent diameter-, dpa (squares), and diameter of gyration-based, dgyr (side triangles), SDs at all HAB, except for HAB = 10 cm of the hot flame where small particles (<5 nm) are formed, which cannot be measured by DMA.
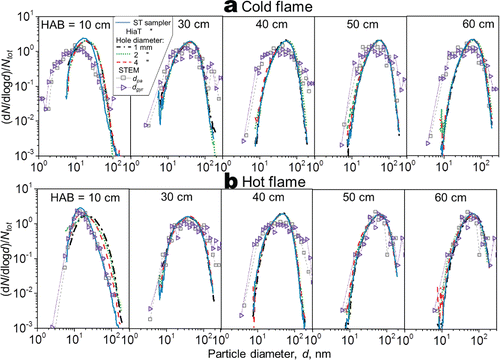
At hot flame conditions (), there is practically no variation among all samplers and even against the SDs obtained by microscopy, when new particle formation is completed (HAB > 10 cm), similar to the cold flame (). At these HAB, the mobility SDs are shifted to larger particle sizes indicating the formation of agglomerated structures that approach their self-preserving size distribution (SPSD) in the continuum regime (see Figures S4 and S5 in the online SI). When sampling takes place above the visible flame height (HAB > 20 cm), the differences between sampler configurations are wiped out (their mean mobility diameters differ less than 10%). The SMPS-obtained mobility SDs (lines), however, are consistently narrower than their corresponding STEM-obtained dpa- (squares) and dgyr-based SDs (side triangles), consistent with Gröhn et al. (Citation2014a). This is probably due to recirculation of particles with longer residence times in the flame jet, particle overlap in microscopy images, and determination of the characteristics of actual three-dimensional agglomerates based on their two-dimensional projection (Brasil et al. Citation1999).
In the transition and continuum regimes, the agglomerate dm is smaller than dpa (Rogak et al. Citation1993). At HAB = 10 cm of the hot flame, sampling takes place within the visible flame so new particle formation is not completed leading to variations between the SDs from the ST and the HiaT samplers attributed to their different particle residence time (particles in HiaT sampler stay ∼ 40% longer than in ST sampler due to differing Re numbers). The ST sampler cools down the aerosol and quenches coagulation much more effectively than all HiaT samplers. For example, for the hot flame at HAB = 10 cm, where sampling takes place inside the flame, fast cooling is essential. There the flame temperature is 1340°C. At the exit of the ST and HiaT samplers the diluted aerosol has been cooled to 264 and 673°C, respectively (see Figure S1 in the SI). The ST sampler gives a slightly smaller mean mobility diameter and smaller large tail of the distribution than all HiaT samplers, regardless of their in-flow hole diameter, but is in excellent agreement with the SD of dpa (squares) obtained by counting STEM images. At HAB = 10 cm, dgyr ≈ dpa (see also ) corroborating further the presence of spherical particles there. It should be noted that at HAB = 10 cm of the hot flame, larger and more spherical particles than those at the cold flame () are present, especially by the ST sampler, so STEM and DMA measure consistent particle sizes.
Some clogging is experienced with all samplers. For the measured mobility SDs such clogging is not significant as they last only 1 min. For the DMA-APM, however, this is important for the ST sampler at HAB = 10 cm in the hot flame (see Figure S6 in the SI) so the CPC concentration is monitored in-between each measurement. Even though the particle number concentration changes considerably, the measured SDs are practically not affected, as shown in Figure S6d (in the SI).
4.2. Effect of sampler orientation
shows the mobility SDs of particles made by (a) cold and (b) hot flames (inset) measured with a HiaT of hole Ø 4 mm (thick red lines) and an ST sampler (thin blue lines) in upstream (solid lines), downstream (broken lines), and sidestream (dotted lines) hole orientations at HAB = 10 cm. The heights of the cold and hot flames are about 5 and 20 cm, respectively (: insets). The SDs are averaged over three consecutive measurements and the highlighted regions show their standard deviation. At cold flame conditions, minor differences in SDs are observed among all sampler configurations as coagulation is suppressed quite effectively during sampling. The hole orientation of the ST sampler hardly affects the mobility SDs at both flames, even though it affects (up to 23%) the Sauter mean dm when the 4 mm HiaT is used. Differences in the measured SD due to samplers’ hole orientation in hot flames are attributed to the ongoing chemical reactions at that sampling height (HAB = 10 cm). The Sauter mean diameters obtained by downstream hole orientation are slightly larger than those obtained by upstream or sidestream ones for most samplers at hot but more consistently at cold flame conditions (see also Table S1 in the SI). The slightly longer trajectory that particles travel to enter the sampler at downstream orientation could explain this.
Figure 5. Mobility size distributions of fractal-like particles made by (a) cold and (b) hot flames (inset) sampled with a HiaT sampler of Ø 4 mm (thick [red] lines) and a straight-tube (ST), thin (blue) lines, in upstream (solid lines), downstream (broken lines), and sidestream (dotted lines) hole orientation at HAB = 10 cm. The hole orientation of the ST sampler hardly affects the mobility size distributions at both cold and hot flames but the deviation of the size distributions using the HiaT orientation is distinguishable. The Sauter mean diameters obtained by the upstream orientation are consistently smaller than those obtained by the downstream and sidestream ones both for the cold and hot flame conditions (see also Table S1 in the SI). The SDs are averaged over three consecutive measurements and the highlighted regions show their standard deviation.
![Figure 5. Mobility size distributions of fractal-like particles made by (a) cold and (b) hot flames (inset) sampled with a HiaT sampler of Ø 4 mm (thick [red] lines) and a straight-tube (ST), thin (blue) lines, in upstream (solid lines), downstream (broken lines), and sidestream (dotted lines) hole orientation at HAB = 10 cm. The hole orientation of the ST sampler hardly affects the mobility size distributions at both cold and hot flames but the deviation of the size distributions using the HiaT orientation is distinguishable. The Sauter mean diameters obtained by the upstream orientation are consistently smaller than those obtained by the downstream and sidestream ones both for the cold and hot flame conditions (see also Table S1 in the SI). The SDs are averaged over three consecutive measurements and the highlighted regions show their standard deviation.](/cms/asset/431676ba-f4f9-4023-90aa-9a08d6716b32/uast_a_1168922_f0005_oc.gif)
In addition, at HAB ≤ 30 cm, sampling by the HiaT samplers leads to larger Sauter mean dm than the ST sampler for both flames, regardless of hole orientation (see Table S1 in the SI). For example, the difference in the Sauter mean dm measured at HAB = 10 cm and for hot flame conditions between the HiaT of Ø 1 mm and the ST sampler in upstream orientation is 55%. For hot flames and HAB > 30 cm, however, that difference is less than 13% as sampling is carried out when new particle formation in the flame has been completed. For cold flame conditions the maximum dm difference (40%) is observed between the 1 mm HiaT and the ST samplers at HAB = 10 cm and sidestream hole orientation. Even though the sampling system design does not practically change the shape of the mobility SDs, it affects the mean particle size due to the different particle residence time in ST and HiaT samplers rather than any sampling variation.
4.3. Effect of sampler design on particle characteristics
shows the Sauter mean PP, dva (filled symbols), and agglomerate mobility diameter, dm (open symbols), for (a) cold and (b) hot flames at HAB = 10–60 cm. The particles were sampled using a HiaT of hole Ø 4 mm (circles) and an ST sampler (triangles) in upstream orientation. The dva obtained by Equation (4) is compared to STEM-obtained dva (squares) as well as to off-line XRD (diamonds) and BET (down triangles) measurements (filter placed at 70 cm above the burner).
Figure 6. Sauter mean primary particle, dva (filled symbols), and agglomerate mobility, dm (open symbols), diameters for (a) cold and (b) hot flames at HAB = 10–60 cm. The dva obtained by sampling (Equation (4)) with an HiaT sampler of Ø 4 mm (circles [red]), and an ST sampler (triangles [blue]), at upstream hole orientation is in close agreement with the corresponding STEM-obtained dva (squares) for HAB = 10–60 cm and XRD (diamonds) and BET (down triangles) measurements. However, the HiaT sampler results in larger dva for HAB ≥ 40 cm and for hot flame conditions compared to the ST sampler and consistently larger dm at most HAB, especially for the hot flame.
![Figure 6. Sauter mean primary particle, dva (filled symbols), and agglomerate mobility, dm (open symbols), diameters for (a) cold and (b) hot flames at HAB = 10–60 cm. The dva obtained by sampling (Equation (4)) with an HiaT sampler of Ø 4 mm (circles [red]), and an ST sampler (triangles [blue]), at upstream hole orientation is in close agreement with the corresponding STEM-obtained dva (squares) for HAB = 10–60 cm and XRD (diamonds) and BET (down triangles) measurements. However, the HiaT sampler results in larger dva for HAB ≥ 40 cm and for hot flame conditions compared to the ST sampler and consistently larger dm at most HAB, especially for the hot flame.](/cms/asset/ad8d335c-51a0-4235-8e15-bec6dbe1407e/uast_a_1168922_f0006_oc.gif)
For the hot flame (), the final PP dva (HAB ≥ 40 cm) is in excellent agreement with the off-line dva measurements. So Equation (4) accurately predicts dva when cluster–cluster agglomeration prevails based only on online measurements. At HAB = 10 cm of the hot flame (), however, new particle formation takes place resulting in spherical particles or droplets (dva ≈ dm) so dva is erroneously larger than the corresponding dm as Equation (4) does not apply. Furthermore, at HAB = 10 cm, the ST sampler results in smaller dm than the HiaT one as aerosol spends about 40% less time in the ST sampler, preventing deposition and coagulation in the sampling lines more effectively than in the HiaT sampler. This is also observed in (HAB = 10 cm) where the ST-obtained SDs are shifted to smaller sizes compared to the HiaT-obtained ones. STEM images and analysis at the above conditions reveal the formation of compact, spherical-like particles as the difference in their dva and dpa is too small. For the hot flame, both sampler designs reveal about the same degree of agglomeration, h (Tsantilis and Pratsinis Citation2004), when dm is used as collision diameter (: at HAB = 40 cm, h = dm/dva ≈ 3.6), consistent with Gröhn et al. (Citation2014a: figure 6) that h ≈ 3.02 at HAB = 10 cm for ZrO2 particles made at similar conditions.
At cold flame conditions (), dva by Equation (4) is in excellent agreement with the STEM-obtained one at all HAB shown here. In addition, the cold flame results in smaller dm and dva than the hot one, qualitatively consistent with STEM images (), due to decreased particle concentration and process temperature. Here, however, more aggregated particles than in the hot flame are formed as indicated by the decreased number-based geometric standard deviation of the mobility diameter, σg,m (see Figure S7 in the SI). Agglomerates made in the cold flame are less polydisperse than in the hot flame as the increased O2 flow rate intensifies turbulence and mixing and narrows down the particle residence time distribution leading to more uniform particle morphology (Camenzind et al. Citation2008). This trend is consistent with Maricq et al. (Citation2003) who reported broader SDs for higher fuel equivalence ratio that corresponds to the hot flame conditions here.
In the cold flame (), the stronger turbulence intensity leads to enhanced flame strain rates and, hence, bigger Damköhler numbers and short reaction times. There, precursor oxidation had been completed at all HAB while the relatively low temperature and high cooling rate imposed by the high strain rate facilitate the rapid quenching of coalescence. At the cold flames, the higher dispersion gas flow rate (compared to the hot flames) decreases the particle residence time at high temperature and accelerates reactant mixing. As a result, nearly perfect agreement is obtained between the two sampler designs for both agglomerate (dm) and primary particle diameters (dva). However, for both flames, the particle residence time range is 1–100 ms at HAB = 10–60 cm (see Figure S14 in the SI).
shows the number of PPs per agglomerate, nva, as function of dm/dSSA at HAB (a) 60 and (b) 10 cm for the cold (open symbols) and hot (filled symbols) flames, using a Ø 4 mm HiaT (circles) and an ST (triangles) sampler. The mass and mobility diameter measured by DMA-APM-CPC and SMPS, respectively, are normalized with the SSA-obtained PP diameter, dSSA (Gröhn et al. Citation2014a). Data from both samplers at HAB = 60 cm () for cold and hot flames reveal largely fractal-like particles of asymptotic Dfm = 2.15–2.39. At these conditions, large (nva > 10) agglomerates have been formed that have attained their asymptotic fractal-like structure (Dfm = 2.2), consistent with Sorensen (Citation2011) and DEM simulations (). The kfm is nearly 1 by the HiaT sampler and 1.20 ± 0.35 by the ST sampler, consistent with the DEM-derived asymptotic kfm = 1.18 ± 0.05.
Figure 7. Average number of primary particles per agglomerate, nva, as function of the normalized mobility diameter, dm/dSSA, at HAB (a) 60 and (b) 10 cm for the hot (filled symbols) and cold flames (open symbols) as sampled using a HiaT sampler with Ø 4 mm (circles) and a ST sampler (triangles). The mass mobility exponent, Dfm, and prefactor, kfm, are obtained from the slope in the log nva vs. log (dm/dSSA) plot. The measurements with the ST sampler reveal more open structures compared to the HiaT sampler except for the hot flame at HAB = 10 cm. At this height, collected particles are either spherical (hot flame) or have formed clusters of few primary particles (cold flame) but have not attained their asymptotic fractal-like structure of Dfm ≈ 2.2 (Figure 2a). At HAB = 60 cm, however, larger fractal-like aggregates/agglomerates are formed having Dfm = 2.15–2.39.
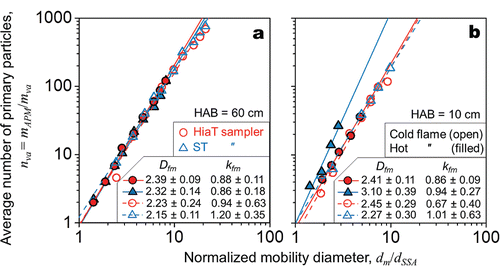
At HAB = 10 cm (), however, particles are either spherical (hot flame) or clusters consisting of a few primary particles (cold flame). It is worth noting that in the cold flame (open symbols) both ST and HiaT samplers reveal fractal-like particles of nearly asymptotic structure. In the hot flame (filled symbols), the ST sampler reveals rather compact particles (Dfm ≈ 3) consistent with thermophoretic sampling (). In contrast, the HiaT sampler reveals particles approaching their asymptotic fractal-like structure (Dfm = 2.41 ± 0.11 and kfm = 0.86 ± 0.09). It is quite likely that the longer particle residence time in this compared to the ST sampler led to some sampler-induced agglomeration and growth of nearly fractal-like structures.
shows the Dfm evolution for the hot (filled triangles) and cold (open triangles) spray flames as function of the relative particle density, measured using HiaT of hole Ø 4 mm (down triangles) and ST (up triangles) samplers along with Equation (6) (solid line) and Equation (3) (dotted line) of Stein et al. (Citation2013). Reasonably good agreement (within experimental error) is obtained between the two sampler designs for the cold flame and the DEM-derived Dfm (Equation (6): solid line), which corresponds to agglomeration of monodisperse PPs in the absence of partial sintering or coalescence. Particles in the cold flame form soft-agglomerates (see also ) with mostly ρeff/ρb < 0.6 that collapse on the theoretical line of unsintered agglomerates (Equation (6)). The hot flame, however, leads to the formation of hard-agglomerates or compact, nearly spherical (ρeff/ρb > 0.3) particles (larger Dfm compared to the cold flame) as also observed by STEM images ().
Figure 8. Mass-mobility exponent, Dfm, for the hot (filled triangles) and cold (open triangles) spray flames as function of the relative density, measured using a HiaT of Ø 4 mm (down triangles) and ST (up triangles) samplers. The results are in agreement with the DEM-derived Dfm (solid line) and experimental data for Ag (Kim et al. Citation2009: circles), ZrO2 (Eggersdorfer et al. Citation2012a: diamonds), and Cu (Stein et al. Citation2013: squares) summarized by a linear fit (Stein et al. Citation2013: equation (6), dotted line). The hot flame leads to the formation of slightly more compact structures compared to the cold one, consistent with Figure 3. When the relative density ranges from 0.1 to 0.5, the DMA/APM-obtained Dfm approaches the asymptotic value of 2.2, consistent with Maricq and Xu (Citation2004) who found that Dfm = 2.2–2.35 for soot particles.
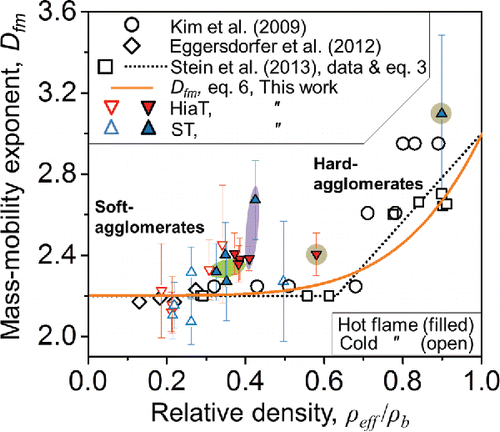
At HAB = 10 cm and hot flame conditions (gray-shaded regions: ρeff/ρb ≈ 0.6–0.9), the ST sampler reveals the formation of spherical particles (Dfm ≈ 3.1), consistent with microscopy (), even though the HiaT sampler measures considerably lower Dfm ≈ 2.4 due to longer residence times in the latter that allow some sampler-induced coagulation-agglomeration. As HAB increases, the agreement between the two samplers is improved, as shown also for SDs (). So at HAB = 30 cm (purple-shaded region: ρeff/ρb ≈ 0.4–0.45), the ST and HiaT samplers reveal more aggregated structures (hard-agglomerates) compared to HAB = 10 cm, with Dfm = 2.7 and 2.4, respectively. At HAB = 60 cm (green-shaded region: ρeff/ρb ≈ 0.3–0.4) where cluster–cluster agglomeration dominates (soft-agglomerate formation), both samplers practically result in identical agglomerate structures (Dfm ≈ 2.3). In the hot flame, when HAB > 30 cm (ρeff/ ρb < 0.4), the DMA-APM-CPC-obtained Dfm approaches the asymptotic value of 2.2 (by both samplers) as the DLCA particle growth mechanism prevails at both flames (particles in the near-continuum regime), consistent with experimental data of soot particles that have Dfm = 2.2–2.35 (Maricq and Xu Citation2004). The DEM-derived Dfm is in agreement with experiments for ZrO2 (Eggersdorfer et al. Citation2012a: diamonds) and Cu (Stein et al. Citation2013: squares) agglomerates while it differs from that of Ag particles (Kim et al. Citation2009: inverse triangles) at large relative densities corresponding to highly sintered hard-agglomerates. Both experimental measurements and Equation (6) are consistent with the experimentally derived correlation (equation (3) of Stein et al. (2013: dotted line).
5. Conclusions
The effect of sampler configuration on particle characteristics during sampling of highly concentrated aerosols at high temperature is investigated. Two samplers, an ST and an HiaT, at three orientations (upstream, downstream, and sidestream) are examined during measurement of flame-made ZrO2 mobility size distributions, structure, and geometric standard deviation for fuel-rich (hot) and fuel-lean (cold) spray flames at 10–60 cm HAB. Furthermore, particle morphology and structure are quantified by the mass-mobility exponent, Dfm, and prefactor, kfm, that are related to the cluster transport properties. Both Dfm and kfm are independent of primary particle size, consistent with Df and kn (Goudeli et al. Citation2015). The Dfm evolution from spherical to fractal-like agglomerates in the absence of sintering, coalescence, or surface growth is quantified as a function of the number of primary particles per agglomerate and relative particle density.
Sampling design does not significantly affect the measured aerosol size distribution; however, it slightly affects the mean particle characteristics: HiaT samplers in downstream hole orientation result in slightly larger (up to 15%) mean mobility diameters than in upstream or sidestream orientation (see Table S1 in the SI). More specifically, these differences become important when gas-to-particle conversion takes place during sampling, typically at the lowest HAB of fuel-rich flames. The present ST sampler leads to smaller mobility diameters than the HiaT sampler due to its 40% shorter residence time and more intense turbulence that suppress further coagulation in the sampling lines, consistent with counting of particle images. At higher HAB when gas-to-particle conversion has ended, all sampler configurations and orientations yield practically the same particle size distributions and structures. So particle characteristics could be affected by sampler design when chemical reactions are still ongoing. Online mobility measurements result in considerably narrower mobility size distributions than the projected area-equivalent diameter- and diameter of gyration-based distributions obtained by microscopy, consistent with literature (Goudeli et al. Citation2015).
With increasing number of constituent primary particles, nva, the average Dfm evolves through a smooth transition from 3 to its asymptotic value of 2.2 (at nva = 10–40) while the corresponding kfm increases slightly from 1 to 1.18, regardless of the primary particle radius, as shown by DEM simulations, consistent with the literature (Goudeli et al. Citation2015). Experimental results obtained by real-time measurements in the literature (Kim et al. Citation2009; Eggersdorfer et al. Citation2012a; Stein et al. Citation2013) are in good agreement to the above Dfm evolution for low relative densities (fractal-like particles). These relationships can be used in process design optimization for aerosol synthesis of materials as well as climate dynamics and meteorological models.
Nomenclature
BET | = | nitrogen adsorption |
d | = | particle diameter, m |
Da | = | projected area exponent |
DEM | = | discrete element modeling |
Df | = | mass fractal dimension |
Dfm | = | mass-mobility exponent |
h | = | degree of agglomeration |
HAB | = | height above the burner |
HiaT | = | hole-in-a-tube sampler |
ka | = | projected area prefactor |
kn | = | pre-exponential prefactor |
kfm | = | mass-mobility prefactor |
Kn | = | gas Knudsen number |
m | = | mass, kg |
nva | = | primary particle number per agglomerate |
r | = | particle radius, m |
Re | = | Reynolds number |
SD | = | size distribution |
SSA | = | specific surface area, m2/kg |
ST | = | straight-tube sampler |
STEM | = | scanning transmission electron microscopy |
T | = | temperature, K |
Greek letters
λ | = | gas mean free path, m |
ρ | = | particle density, kg m−3 |
σg,m | = | number-based geometric standard deviation of mobility distribution |
υ | = | particle/agglomerate volume, m3 |
Subscripts
0 | = | initial |
air | = | air properties |
APM | = | aerosol particle mass analyzer |
b | = | bulk |
eff | = | effective |
g | = | geometric averaging |
m | = | mobility |
p | = | primary particle |
pa | = | projected area |
va | = | volume equivalent |
160311_SI_clean_copy3.docx
Download MS Word (1.5 MB)Acknowledgments
Electron microscopy investigations were performed by Dr. Frank Krumeich at EMEZ (Electron Microscopy Center of the ETH Zürich).
Funding
This research was funded by the Swiss National Science Foundation (grant no. 200021_149144) and the European Research Council under the European Union's Seventh Framework Programme (FP7/2007-2013, ERC grant agreement no. 247283).
References
- Ahonen, P. P., Joutsensaari, J., Richard, O., Tapper, U., Brown, D. P., Jokiniemi, J. K., and Kauppinen, E. I. (2001). Mobility Size Development and the Crystallization Path during Aerosol Decomposition Synthesis of TiO2 Particles. J. Aerosol Sci., 32:615–630.
- Brasil, A. M., Farias, T. L., and Carvalho, M. G. (1999). A Recipe for Image Characterization of Fractal-Like Aggregates. J. Aerosol Sci., 30:1379–1389.
- Camenzind, A., Schulz, H., Teleki, A., Beaucage, G., Narayanan, T., and Pratsinis, S. E. (2008). Nanostructure Evolution: From Aggregated to Spherical SiO2 Particles Made in Diffusion Flames. Eur. J. Inorg. Chem., 2008:911–918.
- De Filippo, A., Sgro, L. A., Lanzuolo, G., and D’Alessio (2009). Probe Measurements and Numerical Model Predictions of Evolving Size Distributions in Premixed Flames. Combust. Flame, 156:1744–1754.
- Dobbins, R. A., and Megaridis, C. M. (1987). Morphology of Flame-Generated Soot as Determined by Thermophoretic Sampling. Langmuir, 3:254–259.
- Eggersdorfer, M. L., Gröhn, A. J., Sorensen, C. M., McMurry, P. H., and Pratsinis, S. E. (2012a). Mass-Mobility Characterization of Flame-Made ZrO2 Aerosols: Primary Particle Diameter and Extent of Aggregation. J. Colloid Interf. Sci., 387:12–23.
- Eggersdorfer, M. L., Kadau, D., Herrmann, H. J., and Pratsinis, S. E. (2012b). Aggregate Morphology Evolution by Sintering: Number and Diameter of Primary Particles. J. Aerosol Sci., 46:7–19.
- Eggersdorfer, M. L., and Pratsinis, S. E. (2013). Restructuring of Aggregates and Their Primary Particle Size Distribution during Sintering. AIChE J., 59:1118–1126.
- Forrest, S. R., and Witten, T. A. Jr. (1979). Long-Range Correlations in Smoke-Particle Aggregates. J. Phys. A: Math. Gen., 12:L109–L117.
- Gormley, P. G., and Kennedy, M. (1949). Diffusion of Stream Flowing through a Cylindrical Tube. P. Roy. Irish Acad. A, 52A:163–169.
- Goudeli, E., Eggersdorfer, M. L., and Pratsinis, S. E. (2015). Coagulation—Agglomeration of Fractal-Like Particles: Structure and Self-Preserving Size Distribution. Langmuir, 31:1320–1327.
- Gröhn, A. J., Eggersdorfer, M. L., Pratsinis, S. E., and Wegner, K. (2014a). On-Line Monitoring of Primary and Agglomerate Particle Dynamics. J. Aerosol Sci., 73:1–13.
- Gröhn, A. J., Pratsinis, S. E., Sánchez-Ferrer, A., Mezzenga, R., and Wegner, K. (2014b). Scale-Up of Nanoparticle Synthesis by Flame Spray Pyrolysis. J. Ind. Eng. Chem., 53:10734–10742.
- Gröhn, A. J., Pratsinis, S. E., and Wegner, K. (2012). Fluid Particle Dynamics during Combustion Spray Aerosol Synthesis of ZrO2. Chem. Eng. J., 191:491–502.
- Haywood, J., and Boucher, O. (2000). Estimates of the Direct and Indirect Radiative Forcing Due to Tropospheric Aerosols: A Review. Rev. Geophys., 38:513–543.
- Heine, M. C., and Pratsinis, S. E. (2005). Droplet and Particle Dynamics during Flame Spray Synthesis of Nanoparticles. Ind. Eng. Chem. Res., 44:6222–6232.
- Heine, M. C., and Pratsinis, S. E. (2007). Brownian Coagulation at High Concentration. Langmuir, 23:9882–9890.
- Jullien, R., Kolb, M., and Botet, R. (1984). Aggregation by Kinetic Clustering of Clusters in Dimension d > 2. J. Phys. – Paris, 45:L–211–L-216.
- Kasper, M., Siegmann, K., and Sattler, K. (1997). Evaluation of an In Situ Sampling Probe for Its Accuracy in Determining Particle Size Distributions from Flames. J. Aerosol Sci., 28:1569–1578.
- Kim, S. C., Wang, J., Emery, M. S., Shin, W. G., Mulholland, G. W., and Pui, D. Y. H. (2009). Structural Property Effect of Nanoparticle Agglomerates on Particle Penetration through Fibrous Filter. Aerosol Sci. Technol., 43:344–355.
- Kuga, Y., Okauchi, K., Takeda, D., Ohira, Y., and Koji, A. (2001). Classification Performance of a Low Pressure Differential Mobility Analyzer for Nanometer-Sized Particles. J. Nanopart. Res., 3:175–183.
- Mädler, L., Kammler, H. K., Mueller, R., and Pratsinis, S. E. (2002). Controlled Synthesis of Nanostructured Particles by Flame Spray Pyrolysis. J. Aerosol Sci., 33:369–389.
- Maricq, M. M. (2004). Size and Charge of Soot Particles in Rich Premixed Ethylene Flames. Combust. Flame, 137:340–350.
- Maricq, M. M., Harris, S. J., and Szente, J. J. (2003). Soot Size Distributions in Rich Premixed Ethylene Flames. Combust. Flame, 132:328–342.
- Maricq, M. M., and Xu, N. (2004). The Effective Density and Fractal Dimension of Soot Particles from Premixed Flames and Motor Vehicle Exhaust. J. Aerosol Sci., 35:1251–1274.
- Mueller, R., Jossen, R., Kammler, H. K., and Pratsinis, S. E. (2004a). Growth of Zirconia Particles Made by Flame Spray Pyrolysis. AIChE J., 50:3085–3094.
- Mueller, R., Jossen, R., Pratsinis, S. E., Watson, M., and Akhtar, M. K. (2004b). Zirconia Nanoparticles Made in Spray Flames at High Production Rates. J. Am. Ceram. Soc., 87:197–202.
- Oberdörster, G., Gelein, R. M., Ferin, J., and Weiss, B. (1995). Association of Particulate Air Pollution and Accute Mortality: Involvement of Ultrafine Particles?. Inhal. Toxicol., 7:111–124.
- Park, K., Cao, F., Kittelson, D. B., and McMurry, P. H. (2003). Relationships to Particle Mass and Mobility for Diesel Exhaust Particles. Environ. Sci. Technol., 37:577–583.
- Pierce, F., Sorensen, C. M., and Chakrabarti, A. (2006). Computer Simulation of Diffusion-Limited Cluster–Cluster Aggregation with an Epstein Drag Force. Phys. Rev. E, 74:021411–021418.
- Ristimäki, J., Virtanen, A., Marjamäki, M., Rostedt, A., and Keskinen, J. (2002). On-Line Measurement of Size Distribution and Effective Density of Submicron Aerosol Particles. J. Aerosol Sci., 18:1541–1557.
- Rogak, S. N., Flagan, R. C., and Nguyen, H. V. (1993). The Mobility and Structure of Aerosol Agglomerates. Aerosol Sci. Technol., 18:25–47.
- Sorensen, C. M. (2011). The Mobility of Fractal-Like Aggregates: A Review. Aerosol Sci. Technol., 45:765–779.
- Stein, M., Kiesler, D., and Kruis, F. E. (2013). Adjustment and Online Determination of Primary Particle Size in Transferred Arc Synthesis of Copper Nanoparticles. Aerosol Sci. Technol., 47:1276–1284.
- Strobel, R., and Pratsinis, S. E. (2007). Flame Aerosol Synthesis of Smart Nanostructured Materials. J. Mater. Chem., 17:4743–4756.
- Tsantilis, S., and Pratsinis, S. E. (2004). Soft- and Hard-Agglomerate Aerosols Made at High Temperatures. Langmuir, 20:5933–5939.
- Ulrich, G. D., Milnes, B. A., and Subramanian, N. A. (1976). Particle Growth in Flames. II: Experimental Results for Silica Particles. Combust. Sci. Technol., 14:243–249.
- Whitney, E. D. (1994). Ceramic Cutting Tools: Materials, Development and Performance. William Andrew Publishing, Park Ridge, NJ.
- Wu, M. K., and Friedlander, S. K. (1993). Note on the Power Law Equation of Fractal-Like Aerosol Agglomerates. J. Colloid Interf. Sci., 159:246–248.
- Zhao, B., Yang, J., Wang, J., Johnston, M. V., and Wang, H. (2003). Analysis of Soot Nanoparticles in a Laminar Premixed Ethylene Flame by Scanning Mobility Particle Sizer. Aerosol Sci. Technol., 37:611–620.