ABSTRACT
Nascent soot particles with mobility diameters ≤10 nm were measured in an ethylene/air premixed flame to shed light on the challenges and potential artifacts affecting studies on soot inception by differential mobility analysis (DMA) techniques. The size distribution functions (SDFs) of particles with charge acquired either naturally or diffusively upon ion seeding were measured at several positions in the flame using rapid-dilution probing and a high-resolution DMA for different values of the ratio of dilution ratio to residence time (DR/Δt). The SDFs are roughly bimodal with a sub-3 nm mode and a larger one that appears either downstream in the flame or for low DR/Δts. Soot nuclei smaller than 3 nm preferentially acquire positive charge, which brings into question the assumption of steady-state charging probability of flame sampled soot nuclei in the bipolar diffusion neutralizer. The approximately polarity-symmetric lognormal SDF of larger particles is attributed to nuclei coagulation. Naturally charged particles increase in number when lowering DR/Δt, suggesting either their collisional charging by flame chemi-ions or particle nucleation by condensation of neutral molecules on ions or both. The critical conditions for suppressing particle coagulation and charge redistribution in the sampling system were not achieved under most conditions, despite the fact that values of DR/Δts were more favorable to such a suppression in the present experiment as compared to other studies in the literature. As a result, the identification of this “asymptotic” regime, which is critical to determine the parent SDFs and the charge state of nascent soot in the flame, is still elusive.
© 2016 American Association for Aerosol Research
EDITOR:
1. Introduction
Although much research has been devoted to soot in the past three decades, the topic of soot nucleation or inception, that is, the formation of the first soot nanoparticles, is still poorly understood, despite its critical role in the subsequent evolution of soot (Haynes and Wagner Citation1981; Bockhorn et al. Citation2009; D'Anna Citation2009; Wang Citation2011). Soot inception is considered to contribute little per se to the total soot loading and eventual emissions from the combustion processes, but the nuclei experience surface growth by acetylene addition, which is thought to be responsible for the bulk of the overall soot production (Frenklach and Wang Citation1991; Glassman and Yetter Citation2008; Bockhorn et al. Citation2009). The critical and still missing link to a fundamental understanding of soot formation in flames is precisely the soot inception step.
In an effort to clarify the mechanisms of the transition from the gas to the particle phase leading to soot formation in flames, many studies have reported measurements of ultrafine (D < 100 nm) size distribution functions (SDFs) of particles formed in incipiently sooting conditions by performing online differential mobility analysis (DMA) of sampled and quickly diluted flame products (Maricq et al. Citation2003; Zhao et al. Citation2003a,Citationb, Citation2005; Maricq Citation2004, Citation2005, Citation2006, Citation2007, Citation2008; Manzello Citation2007; Sgro et al. Citation2007, Citation2010, Citation2011; Abid et al. Citation2008, Citation2009a,Citationb; De Filippo et al. Citation2009; Echavarria et al. Citation2009, Citation2011a,Citationb; Camacho et al. Citation2013, Citation2015; Ghiassi et al. Citation2014). Two commercially available DMA systems were used for these studies with a maximum resolution of 10. The most commonly used system was a nanoDMA (TSI Inc., Shoreview, MN, USA). This instrument has a nominal lower detection limit of 2.5 nm to 3 nm, even though diffusive effects in the electrostatic classifier and the associated particle losses and broadening of the transfer function are already significant for sizes below 6 nm (Stolzenburg Citation1988; Flagan Citation1999, Citation2011, Citation2014; Jiang et al. Citation2011). The existence of carbonaceous particles with size below 3 nm was confirmed qualitatively using this instrument (Abid et al. Citation2009a). A few studies of the group in Naples (Sgro et al. Citation2007, Citation2010, Citation2011; De Filippo et al. Citation2009) extended the lower-dimensional range of DMA measurements below 3 nm by relying on a Tapcon Vienna DMA (Winklmayr et al. Citation1991; Rosell-Llompart et al. Citation1996; Fernandez de le Mora Citation2011; Jiang et al. Citation2011).
Inferences of the SDF of the entire particle population in these studies relied exclusively on the detection of one polarity of the charged particles (i.e., negative for the nanoDMA and positive for the Vienna DMA), and presumed the achievement of a steady-state charge probability in the diffusive charger to correct the measured signal (Fuchs Citation1963; Liu and Pui Citation1974; Adachi et al. Citation1985; Hoppel and Frick Citation1986, Citation1988, Citation1990; Wiedensohler et al. Citation1986; Wiedensohler Citation1988; Reischl et al Citation1996; Alonso et al. Citation1997, Citation1998, Citation2003; Stommel and Riebel Citation2007; Lopez-Yglesias and Flagan Citation2013; de La Verpilliere et al. Citation2015; Gopalakrishnan et al. Citation2015; Jiang et al. Citation2015; Tigges and Schmid Citation2015). To the best of the authors' knowledge this assumption has never been tested for sizes below 5 nm for carbonaceous particles directly sampled from a flame together with flame generated ions, even though it is known that the steady-state charge probability in this size range can be severely affected by changes in the ion population (Hoppel and Frick Citation1986, Citation1990; Gopalakrishnam and Hogan Citation2012; Steiner et al. Citation2014; Jiang et al. Citation2015; Tigges and Schmid Citation2015) and by particle morphology and electrical properties (Gopalakrishnam et al. Citation2013, Citation2015). Additionally, it has been known for a long time that soot is naturally partially charged in flames (Lawton and Weinberg Citation1969; Calcote Citation1981; Burtscher Citation1992; Calcote and Gill Citation1994) but only some studies considered the charge that particles naturally acquire in the flame (Maricq Citation2004, Citation2005, Citation2006, Citation2008; Sgro et al. Citation2010, Citation2011).
As part of a broader investigation of the gas-to-particle conversion in soot processes (Carbone and Gomez Citation2012, Citation2013; Figura and Gomez Citation2015; Carbone et al. Citation2015; Figura et al. Citation2015), we performed high-resolution measurements of carbonaceous nanoparticle SDFs (D < 10 nm) using a recently developed half-mini DMA (Fernandez de la Mora Citation2011a; Fernandez de le Mora and Kozlowski Citation2013). Our measurements were performed on a fuel-rich laminar premixed flame of ethylene and air that was identified as one of the target flames at the International Sooting Flame Workshop (2014; http://www.adelaide.edu.au/cet/isfworkshop/) and that had been already studied with a DMA (Maricq Citation2004, Citation2005; Sgro et al. Citation2007). We report our findings in a systematic investigation in which sampling line and charging procedures were varied. We identify challenges and artifacts in the measurement of nascent soot particles by DMA and also highlight those possibly originating in the data inversion algorithms. As discussed below, these artifacts may mislead the interpretation of measurements with particles smaller than 5 nm, but could even affect the correct SDF determination for larger particles in the inception region of the flame. One of the goals of the present work is to stimulate further DMA work on soot nucleation using high-resolution instruments to shed light on the highlighted problems and on soot nucleation itself. These problems are relevant in general to DMA measurements of nanoparticles nucleating at high temperatures.
2. Experimental methods
2.1. Burner and flame conditions
The flame of choice for this study is a laminar premixed ethylene/air flame with C/O ratio of 0.69 and a cold gas velocity of 5.87 mm/s. This flame was extensively studied in the context of soot formation (ISF workshop 2014; http://www.adelaide.edu.au/cet/isfworkshop/). The SDFs of soot generated in this flame were systematically measured, using the TSI nanoDMA system, at several heights above the burner (HAB) above 10 mm by Maricq (Citation2004, Citation2005). Sgro et al. (Citation2007) also examined this flame at HAB = 10 mm in an effort to extend the lower-dimensional limit of measurements below 3 nm. Whereas the target flame in those studies was stabilized over a water-cooled porous-plug McKenna burner, the present flame was stabilized with the same stoichiometry and cold-gas velocity on a 400 CPSI stainless steel honeycomb burner with an outlet diameter of 48 mm. Pure nitrogen with a velocity of 21 cm/s was flown through an outer annular region (OD 76 mm) of the same honeycomb to shield the flame from external disturbance. A 1.6-mm thick brass plate, 150 mm in diameter, was placed at a HAB = 40 mm to suppress further flame flickering and hot gas buoyant acceleration. An image of the flame recorded with a digital camera (Canon EOS D30) is shown in for illustrative purposes. Temperature, gas speciation, and soot volume fraction measurements confirmed that the present flame is not appreciably different from the one stabilized using the water-cooled burner.
2.2. High-resolution differential mobility analysis
The flame-formed aerosol was analyzed using a high-resolution half-mini DMA (Fernandez de la Mora Citation2011a; Fernandez de la Mora and Kozlowski Citation2013). The cylindrical DMA was equipped with a slightly conical “bullet” constituting the internal electrode of the electrostatic classifier whose nominal radius was Rin = 4 mm at the outlet slit. The nominal radius of the external cylindrical electrode, Rout, was 7 mm. The electrostatic classification nominal length, L, i.e., the distance between the electrostatic classifier inlet and outlet slits, was 20 mm. A schematic of the electrical and flow configuration of the DMA system is depicted in . The DMA was operated in a closed-loop configuration, recirculating the almost pure (impurities always below 0.1% mol, including the diluted sample) nitrogen in the sheath flow, Qsh. Operating in closed loop balanced the sample inlet and outlet flows, QDMA, and maximized the instrument resolution (Knutson and Whitby Citation1975; Stolzenburg Citation1988; Flagan Citation1999, Citation2011, Citation2014; Stolzenburg and McMurry Citation2008; Fernandez de la Mora Citation2011a). Recirculation was achieved using a brushless DC vacuum motor (model 116119–00, Ametek Inc., Wallingford, CT, USA) with interposition of a HEPA filter in the loop to remove any particle from the flow crossing the electrostatic classification section. The DMA flow to be analyzed, QDMA, was drawn through the sample inlet slit and was monitored and kept constant using a rotameter and a needle valve interposed between the outlet slit and a vacuum pump. All measurements were performed with a flow rate ratio, Qsh/QDMA, of 20 (Qsh = 100 slpm, QDMA = 5 slpm). The flow rate ratio equals the resolution of the instruments when particle diffusion in the classification section is negligible (Knutson and Whitby Citation1975; Stolzenburg Citation1988; Flagan Citation1999, Citation2011, Citation2014; Stolzenburg and McMurry Citation2008), i.e., for mobility diameters above 2 nm with the used configuration. A high-voltage power supply was used to apply a stepwise varying voltage, VDMA, ranging from 0 kV to ±5 kV between the electrodes of the electrostatic classifier. The external electrode, the DMA body, and the sampling system were electrically grounded.
At any given VDMA, the aerosol exiting the slit on the internal electrode of the DMA is almost monomobile, as detailed in the online supplementary information (SI), that is, monodisperse in terms of electrical mobility, ZDMA, and is charged with polarity opposite to that of the applied voltage:[1]
The first term on the right of Equation Equation(1)[1] represents the electrical mobility that would be filtered by a perfectly cylindrical classification section (Rin = 4 mm; Rout = 7 mm; L = 20 mm). The correction factor, Ccone, accounting for the conical shape of the inner electrode, was quantified to Ccone = 1.203 upon calibration of Qsh and ZDMA. Calibrations relied on a reference positive displacement flowmeter to measure Qsh, and the tetraheptyl ammonium ion THA+ electrosprayed from a 100-μM ethanol solution of its bromide salt (99.9%, Sigma-Aldrich), with known inverse electrical mobility and mobility diameter (1/ZTHA+ = 1.030 V s cm−2, DTHA+ = 1.47 nm) (Ude and Fernandez de la Mora Citation2005), to identify the THA+ peak voltage VDMA = VTHA+. Measurements of VTHA+ were performed before and after each flame experiment to infer the actual flowrates and check for stability.
The mobility diameter, D, of particles classified at each VDMA was inferred by assuming that particles carry a single elementary charge and that, according to the Stokes Millikan law in the free-molecular regime approximation (Hinds Citation1999; Friedlander Citation2000), D is inversely proportional to the square root of ZDMA:[2]
The unity elementary charged state is expected to dominate for charged particles smaller than 10 nm (Fuchs Citation1963; Hoppel and Frick Citation1986, Citation1988, Citation1990; Wiedensohler et al. Citation1986; Wiedensohler Citation1988; Reischl et al Citation1996; Stommel and Riebel Citation2007), which allows a straightforward conversion of electrical mobility to D. The largest value of D with the present DMA configuration is 10.84 nm. Even if one considers calibration accuracy and daily variations in the atmospheric conditions, D is underestimated by using Equation Equation(2)[2] instead of the full Stokes Millikan law, by less than 2%; the smaller the diameter, the smaller the underestimate. There is one additional effect unaccounted for when showing the results in terms of mobility diameter. D is an overestimate of the particle mass equivalent sizes (Fernandez de la Mora et al. Citation2003; Larriba et al. Citation2011; Steiner et al. Citation2014) since it includes an effective size, D0, of the transport gas molecules accounting for polarization/ion induced dipole effects (Tammet Citation1995; Fernandez Garcia and Fernandez de la Mora Citation2013; Larriba and Hogan Citation2013). For measurements in nitrogen, D0 is just a few tens of nanometer, which implies that the correction of D would be appreciable only in the 1-nm size range. For the reported datasets, D was not corrected for this effect since the actual values of D0 depend on the unknown specific properties of the measured particles (Fernandez Garcia and Fernandez de la Mora Citation2013; Larriba and Hogan Citation2013). As a representative example of the accuracy of D in the smallest dimensional range as well as of the capability of measuring small molecular clusters, the mobility diameter of pyrene (C16H10) was estimated from its diffusivity in nitrogen at 298 K to be 1.04 nm whereas its mass equivalent diameter is 0.80 nm.
The classified monodisperse charged aerosol leaving the electrostatic classifier outlet slit was conveyed to a Faraday cup (FC) electrometer before crossing the rotameter, the needle valve, and the pump. The FC converted the electrical current IFC carried by the monodisperse charged aerosol flow to a voltage, VFC, through a 1011 Ω resistance (Burtscher Citation1992). VDMA and the corresponding properly amplified VFC were controlled/measured using a digital data acquisition system (Data Translation Inc.) and a LabView (National Instruments Corporation) program.
2.3. Aerosol dilution sampling and charging system
Nascent soot particles were sampled from the flame at several HABs up to 12.5 mm in incremental steps of 2.5 mm, using a set of stainless steel rapid dilution probes (6.35 mm OD, 305 mm length, and 0.125 mm wall thickness). Similar probes were designed to minimize aerosol evolution in the path from the sampling orifice to the analysis system (Kasper et al. Citation1997) and have been used in studies on soot and other combustion generated particles (Zhao et al. Citation2003a,Citationb; Maricq Citation2004; Manzello Citation2007; Sgro et al. Citation2007, Citation2010, Citation2011; Abid et al. Citation2008, Citation2009a,Citationb; De Filippo et al. Citation2009; Echavarria et al. Citation2009, Citation2011a,Citationb; Carbone Citation2010; Carbone et al. Citation2010, Citation2011; Camacho et al. Citation2013, Citation2015; Ghiassi et al. Citation2014). The aerosol was drawn through an orifice drilled through the probe wall and facing the center of the burner outlet. A small and constant under-pressure at ≈ −600 Pa Gauge was established in the probe by a rotary-vane vacuum pump and a back-pressure vacuum regulator, as depicted in . The aerosol was quickly diluted in a crossflow configuration with 30 slpm of pure nitrogen and the diluted aerosol was conveyed to the DMA inlet port using additional stainless steel tubing.
Figure 3. Schematic of the dilution sampling and charging system. From top to bottom: (A) traditional aerosol charging using a radioactive bipolar charger; (B) aerosol charging through the use of an ion-enriched dilution nitrogen flow generated upstream of the probe by a radioactive bipolar charger; (C) aerosol naturally getting charged upon flame sampling.
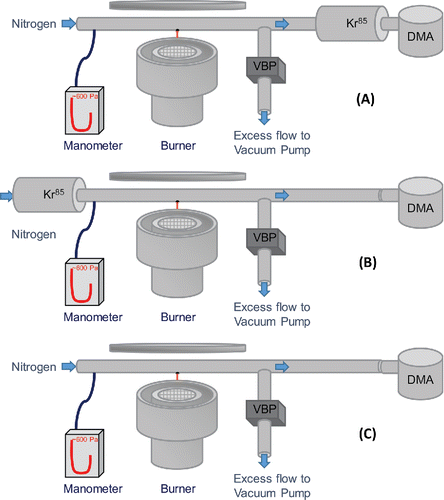
Even though the dilution ratio (DR), that is, the ratio of the standard volumetric flow rate of nitrogen to the sampled one, can be adjusted by changing the under-pressure in the probe, as in most studies of this type, and/or the dilution nitrogen flow rate, we chose to adjust it by using a set of probes differing only in orifice diameter, while keeping everything else unchanged (Carbone Citation2010; Carbone et al. Citation2010, Citation2011). The steadiness of the under-pressure and of the dilution flow in the probe ensured constant velocity through the orifice (Abid et al. Citation2008; Carbone Citation2010; Carbone et al. Citation2010; Camacho et al. Citation2015) and through the probe itself, simplifying the result interpretation. A constant sampling velocity fixes sampling artifacts related to the probe intrusiveness. Additionally, for a given flow pattern, the steadiness of the dilution flowrate makes the percentage of particles surviving diffusive losses through the sampling and charging system a decaying function of the residence time (Wang 2003) or, equivalently, of the tube length (Hinds Citation1999; Kumar et al. Citation2008; Ghaffarpasand et al. Citation2012). We achieved DRs of approximately 6.2 103, 1.8 103, 6.6 102, 3.2 102, and 1.6 102 by selecting orifice diameters of 0.08 mm, 0.15 mm, 0.25 mm, 0.36 mm, and 0.5 mm, respectively. DRs were calibrated by gas chromatography measurements of flame products microsampled at several HAB directly from the flame and from the sampling probe with a technique detailed elsewhere (Carbone and Gomez Citation2012, Citation2013; Carbone et al. Citation2015). Calibrated values were in close agreement with those obtained by performing three-dimensional modeling of the probe-induced flow perturbation. On the basis of the size of the orifice and of the value of the under-pressure in the sampling probe, the DR values in the present study certainly encompass the range used in past studies with DMA analysis of nascent soot. However, we note that the values reported in the literature are not all consistent among themselves or with our GC calibrations (Zhao et al. Citation2003; Abid et al. Citation2008; Camacho et al Citation2015).
The residence time of the aerosol was determined by the flow pattern that was partially dictated by the need to supply charged particles to the DMA system. One of the novelties of the present study is that three flow configurations were used, two of them to measure purposely charged particles and one to measure particles whose charge was naturally acquired either in the flame or, possibly, in the sampling system itself, as schematically shown in . In all cases, the diluted aerosol initially flowed through the stainless steel tube probe for a residence time of 9 ms, before being split into the QDMA drawn into the DMA inlet port and an excess flow that was exhausted through the pump. After being conveyed to the DMA inlet port, the aerosol spent an additional 32 ms to reach the DMA inlet slit.
In the first traditional flow configuration (A), QDMA crossed a bipolar diffusion charger based on Kr85 (model 3077, TSI Inc., Shoreview, MN, USA; November 1987, activity of 0.344 mCi), within which the aerosol spent 1200 ms colliding with ions generated by radioactive decay and diffusively acquiring a charge before entering the DMA inlet port. In the other two flow configurations (B and C) the aerosol spent only 67 ms in the stainless steel tube (6.35 mm OD, 197 mm length, and 0.254 mm wall thickness) used to convey QDMA to the DMA inlet port. Using the short residence time flow configuration, measurements were performed either by (B) seeding bipolar ions in the dilution nitrogen flow to diffusively charge the aerosol or by (C) relying on the charge that particles acquired naturally. Ions seeding was obtained by installing the radioactive charger upstream of the probe that is 155 mm (equivalent to 10 ms) before the sampling orifice. The dilution nitrogen spent 200 ms in the charger where free bipolar ions were generated, before entering the sampling probe. Particles acquired charge by colliding with the ions in their path from the orifice to the DMA inlet slit. The applied DRs and the total aerosol residence time, Δt, from the sampling orifice to the DMA inlet slit are listed in
Table 1. Conditions of the sampling and charging system flow configurations.
3. Data analysis
3.1. Inversion of the DMA signal
The raw DMA measurement is a current versus voltage curve IFC (VDMA). This information can be converted into the number SDF, dN/dlnD, of charged particles present at the DMA inlet slit on the external electrode, by performing an approximation of the DMA transfer function (Stolzenburg Citation1988; Stolzenburg and McMurry Citation2008; Flagan Citation2014) that is valid for D larger than 2 nm with the used DMA configuration, and considering that the analyzed particles carry a single elementary charge, ±e. Thus, as detailed in the SI,[3]
The first term on the RHS is the number of singly charged particles per unit volume in the classified aerosol reaching the FC; the second term accounts for the DMA flow limited resolution; the third term accounts for particle diffusion losses through the penetration efficiency PFC, in the path from the DMA exit slit to the FC. The latter is computed for a laminar flow (Re ≈ 2200) in a 0.25-m long tube (Hinds Citation1999; Kumar et al. Citation2008).
3.2. Inferring SDFs in the flame
Since the particle number concentration in the sampling system ideally decreases proportionally to DR, the measured SDF should be the same at each sampling position for constant values of the ratio DR/Δt (reported in ) because of the similar effectiveness in quenching particle coagulation. The measurements should also give the SDF of particles effectively present in the flame if DR/Δt is larger than a critical value necessary to completely quench the aerosol evolution in the sampling and charging system:[4] with
[5]
On the RHS of Equation Equation(5)[5] the SDF obtained by the DMA data is corrected for the parameters accounting for the sampling and charging procedures. Among these parameters, the first term accounts for the applied dilution and the second for the change in gas density due to the temperature difference between the flame, Tflame ≈ 1680 K, and the diluted sample, TDMA ≈ 300 K. The third and the fourth terms consider diffusion losses in the path from the sampling orifice to the DMA inlet slit and the charging probability, ηCharg, that is the fraction of particles being charged, at the DMA inlet slit. The penetration efficiency in the sampling and charging system, Psamp, was estimated by using standard correlations (Kumar et al. Citation2008) and considering the laminar flow (Re < 500) within the sampling orifice, the turbulent flow (Re ≈ 7000) within the probe tube itself, and the laminar flow (Re < 1500) eventually crossing the radioactive charger (A flow configuration) to reach the DMA inlet slit. The diameter dependence for the total penetration efficiency, i.e., Psamp.PFC, is shown in for all orifice sizes.
Figure 4. Parameters used in the data inversion as a function of particle size: (a) total penetration efficiency, Psamp PFC, for different sampling orifice diameters as detailed in the legend; (b) Wiedensohler (Citation1988) approximation, ηWied, of the steady-state diffusion charging probability compared to the equilibrium charge probabilities at the probe and flame temperatures, respectively (see legend); (c, d) transient charging correction factor (symbols) parametrically calculated for the A and B flow configuration as detailed in the online SI, for two values of the particle number concentration—the dashed lines represent the constant values used for data inversion.
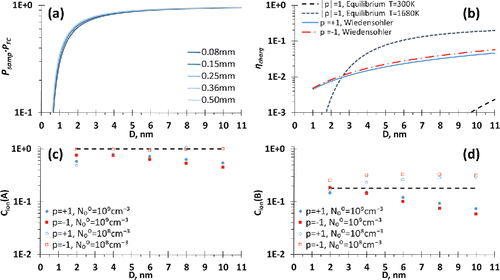
3.3. Estimation of the charging probability
In most DMA applications based on bipolar diffusion charging, ηCharg is estimated by assuming steady state for the diffusional charging mechanisms. This charge probability is affected by the values of the electrical mobility and molecular masses of the ions (Hoppel and Frick Citation1986) but is generally approximated by a simple function of D, ηWied, introduced by Wiedensohler (Citation1988) and often used for data inversion. shows ηWied for particles carrying either a positive or a negative single elementary charge. For illustrative purposes, also shows the polarity symmetric equilibrium charge probabilities that would be asymptotically achieved in the absence of a nonequilibrium ion source (e.g., radioactive decay or chemi-ionization) at the prevailing temperatures.
The reasonable accuracy of ηWied in reproducing ηCharg achieved at ambient temperature in radioactive bipolar chargers by an initially neutral and monodisperse aerosol with sizes down to 2 nm is supported by several experimental and theoretical studies (Reischl et al Citation1996; Gopalakrishnan et al. Citation2013, Citation2015; Lopez-Yglesias and Flagan Citation2013; de La Verpilliere et al. Citation2015; Jiang et al. Citation2015; Tigges and Schmid Citation2015). This is the case for almost monodisperse particles with a relatively low number concentration if the activity of the radioactive source and the residence time of the aerosol in the neutralizer are sufficient for the achievement of this steady state (Jiang et al. Citation2015). However, the smallest nanoparticles can even disappear faster than they acquire charge because of coagulation in the charger (Alonso et al. Citation1998). As a result, there are conflicting requirements on the residence time. The B flow configuration was designed purposely to overcome this problem, by sacrificing the ability to achieve the steady-state charging probability. Steady state may not be achieved even with the A flow configuration because of the expected high number concentration of the smallest soot nuclei. When steady-state bipolar diffusion charging is not achieved ηCharg differs from the estimated ηWied because of a diameter- and polarity-dependent transient charging/recombination correction factor, Cion:[6]
From a theoretical point of view, the exact determination of Cion requires the solution of the population balance equations for coagulating particles (charged and uncharged) and ions starting from their initial SDFs and charge status (Alonso et al. Citation1998). This poses a problem since the initial conditions are precisely what we are trying to determine. We pursued instead a parametric estimation of Cion following the approach of Hoppel and Frick (Citation1990; de La Verpilliere et al. Citation2015), details of which are provided in the SI. The parametrically modeled values of Cion at the DMA inlet slit are shown in for the A and B flow configurations, respectively. For simplicity, in the ensuing data analysis we assumed Cion(A) = 1 and Cion(B) = 0.18, as shown by dashed lines in the figures. This simplification is accurate within a factor of 2 with respect to the parametrically modeled values. Estimating the charge probability of naturally charged particles, i.e., Cion(C), from the measurement results and discussing its variability with DR are additional objectives of this study. The analysis of the sensitivity of Cion to variations in the assumed charging kinetic parameters is beyond the scope of this article, but is partially embedded in since those are the only parameters of the model being a function of D, as detailed in the online SI.
4. Results and discussion
4.1. Particles charged diffusively in a bipolar radioactive charger
shows the SDFs measured with the A configuration (), with the particles diffusively charged right upstream of the DMA inlet port as they crossed the radioactive bipolar charger. Data are presented at several HABs showing the SDFs inferred from measuring particles carrying either positive (left column) or negative (right column) charges at the DMA inlet slit. The data are reported for three different DR/Δts, as listed in
Figure 5. Total particle SDFs inferred by measuring diffusionally charged particles carrying either positive (left column) or negative (right column) charge acquired after crossing the radioactive bipolar charger (configuration A in ). Measurements were performed at different HABs with DR/Δt varying by a factor of 6. Dotted lines represent the “background” SDFs of gaseous ions detected in the presence of only nitrogen flow.

Soot SDFs are contrasted with those of neutralizer ions shown as dotted lines. The latter were measured in particle-free nitrogen and were generated in the charger by ionization of gaseous impurities. These flameless ions provide a repeatable background signal that is dilution independent and whose SDF artificially increases with DR because of the dilution correction in Equation Equation(5)[5] . The total number concentration of ions detected at the DMA inlet slit (n+ ≈ n– = 2.2 ± 0.2 106 cm−3) corresponds to n+ ≈ n– = 4.0 ± 0.8 106 cm−3 once one accounts for diffusional losses (but not recombination) in the path from the outlet of the neutralizer to the DMA inlet slit. These values are in agreement with those obtained by solving the ion population balance model (not including diffusional losses) for the A configuration and
(see the SI). The ion background cannot be directly subtracted from the particle SDFs because neutralizer ions are progressively depleted at increasing aerosol concentrations by charge transfer to the particles. The negatively charged neutralizer ions in nitrogen are smaller than the positively charged ones, their SDF extending to D < 1 nm. The measured SDFs at HAB = 0 mm, i.e., in the unreacted C2H4/air mixture, are virtually indistinguishable from those obtained by sampling pure nitrogen.
At HAB = 2.5 mm the slight differences between the two sets of curves cannot be appreciated in the logarithmic scale of , so these data are not included in the figure. The difference from the background signal is generally less appreciable with increasing dilution since the number concentration of charged particles reaching the DMA inlet slit generally decreases whereas the number concentration of the neutralizer ions in nitrogen remains constant. The particle SDFs in are roughly bimodal with a sub-3 nm mode and a larger one that was detected only for HAB ≥ 10 mm. At all dilutions, and especially for the positive polarity, the concentration of the sub 3-nm mode visibly exceeds the background already at HAB = 5 mm, and persist at all sampling positions up to HAB = 12.5 mm. At this height, soot with size above 5 nm has already formed, as suggested also by the relatively fast clogging of the probe orifice and by the luminosity observable in . The SDFs at the larger mode seems to approach the shape of a lognormal distribution further downstream in the flame, indicating that coagulation is the mechanism for its formation. The presence of small particles even at the downstream positions suggests also that particle nucleation persists in the presence of coagulation.
The SDFs of all particles inferred from measurements in positive and negative polarities are almost indistinguishable for D > 3 nm. For smaller D they deviate increasingly from each other, with a significantly higher (up to six times larger) number concentrations of positively charged particles. This difference indicates that flame-sampled soot particles smaller than 3 nm do not achieve the charge probability estimated by Wiedensohler (Citation1988), because they preferentially acquire a positive charge. This observation does not necessarily mean that steady state is not reached, but may be due to variations in the kinetic ionization and recombination constants associated with the concurrent sampling of flame ions and to the properties and the initial charge state of the nuclei (e.g., large aromatic molecules are more prone to form cations than anions). Clearly, one should directly measure diffusional charging probability in both polarities, while previous studies examined only particles of one polarity with the same DMA system (Sgro et al. Citation2007, Citation2010, Citation2011; Abid et al. Citation2009a; De Filippo et al. Citation2009) and presumed the achievement of ηWied in the diffusional charger (Wiedensohler Citation1988; Reischl et al. Citation1996; Alonso et al. Citation1997) to infer the SDFs and determine the number concentration of particles smaller than 3 nm in the flame.
At all sampling positions, increasing dilution causes a narrowing of the SDFs, a mode-shift toward smaller sizes, and an increase in number concentration. This trend is evidently due to a reduction in coagulation in the sampling system, even though we already exceeded the DR/Δt ratios reported in prior flame studies in which particle coagulation in the probe was allegedly suppressed. For the measured number concentrations of particles, using a rigid body collision kernel approximation (Hinds Citation1999), we estimated that the coagulation half-life of monodisperse 2-nm particles such as those detected at HAB ≥ 7.5 mm is on the order of 800 ms and 4 ms at the probe and flame conditions, respectively. These times are an upper limit estimation of the real characteristic coagulation times since they neglect the existence of larger particles with a larger collisional cross-section and of possible interactions among particles. Nonetheless they are shorter than the residence time in the sampling and charging system and correspond to an HAB displacement in the flame burnt gases of 1.2 mm that is about a half of the distance between two adjacent sampling positions. It is noteworthy that the SDFs in measured at HAB = 10 mm for DR/Δt = 1.5 ms−1 are virtually identical to that reported by Maricq (Citation2004) for the same flame at HAB = 10 mm. The PSDs in measured at HAB = 12.5 for DR/Δt ≥ 0.53 ms−1 are instead already shifted toward smaller sizes as compared to those reported by Maricq (Citation2004) for the same flame at HAB = 12 mm. This finding suggests that the low DR/Δt asymptote is not easily achieved, nor is this deficiency readily diagnosed with DMAs not designed to analyze particles smaller than 3 nm. Note, in particular, that quenching of coagulation brings the size of a large number of particles below 3 nm, where they cannot be effectively quantified by most DMAs because of losses and diffusional broadening of the DMA transfer function (Stolzenburg Citation1988; Stolzenburg and McMurry Citation2008; Jiang Citation2011).
4.2. Particles charged diffusively by an ion-seeded dilution flow
The use of a short residence time flow configuration (B in ) for diluting and charging the particles was explored in the hope of achieving the critical value of DR/Δt needed to quench particle coagulation in the sampling system. This approach allows for a drastic reduction of the residence time of the aerosol in the path from the orifice to the DMA inlet slit (108 ms vs. 1241 ms). The disadvantage of the absence of ion replenishment during the charging process and of a shorter time (108 ms vs. 1232 ms) for the achievement of ηWied as compared to the traditional configuration (A) is inconsequential since such charge status was not achieved for D < 3 nm even in the A configuration, as shown in comparing results with opposite polarity.
As noticeable in , the background SDFs due to the ions seeded with the radioactive charger and measured by sampling pure nitrogen have an almost identical shape to the ones measured with the traditional diffusive charging configuration (A). However, their total number concentrations at the DMA inlet slit are significantly lower (n+ ≈ n– = 5.2 ± 1.4 105 cm−3) because of recombination and diffusional losses. They correspond to n+ ≈ n– = 1.9 ± 0.6 106 cm−3 once one accounts for diffusional losses in the path from the outlet of the neutralizer to the DMA inlet slit. Those values are in agreement with the ones obtained by modeling the ion population balances (not including diffusional losses) for the B configuration and (see online SI).
Figure 6. Total particle SDFs inferred by measuring diffusionally charged particles carrying either a positive (left column) or a negative (right column) charge acquired after being diluted with an ion-seeded nitrogen flow (configuration B in ). Measurements were performed at different HABs with DR/Δt varying by a factor of 10. Dotted lines represent the “background” SDFs of gaseous ions detected in the presence of only nitrogen flow.

The SDFs at all the sampling positions resulting by assuming the constant value Cion(B) = 0.18 are shown in and they are consistent with those in . Specifically, the SDFs obtained for DR/Δt = 6.1 ms−1 are almost identical at HAB = 12.5 mm to the ones in measured for DR/Δt = 1.5 ms−1, especially for D ≥ 6 nm, that is, the range where the parametric charging model predicts the largest variability of Cion(B) in . This consistency gives further confidence in the relative accuracy of the estimated constant value of Cion(B). The SDFs of measured at HAB ≤ 10 mm are instead slightly shifted toward smaller sizes as compared to those in , because of a more effective quenching of coagulation. The measured SDFs at HAB = 0 mm (unreacted C2H4 mixture) and at HAB = 2.5 mm are not shown in since they are indistinguishable in the logscale plot from those obtained by sampling pure nitrogen.
As in , at all sampling positions increasing DR/Δt causes a more effective suppression of particle coagulation. In view of the increased number concentrations of the smallest particles detected with the B flow configuration, the estimated coagulation rates can increase up to one order of magnitude compared to the estimates performed with the results obtained in the traditional A flow configuration. Whereas the latter suggested the imminent approach of asymptotic conditions with increasing dilution, it appears that coagulation is potentially effective on timescales shorter than 100 ms in the probe and well below 1 ms (ΔHAB smaller than 0.3 mm) in the flame. Neither the rapidity of coagulation nor the challenges to properly quench coagulation would have been appreciated without performing these nontraditional measurements. Note that not even achieving a DR/Δt of 57.6 ms−1 (at least ten times larger than that of traditional configurations) demonstrated the suppression of particle coagulation in the sampling line. Only at HAB = 12.5 mm and exclusively for diameters larger than 6 nm the measured SDF is nearly independent of the applied dilutions for DR/Δt larger than 6.1 ms−1 (1.5 ms−1, considering also data in ). This evidence confirms a likely bias in previously published SDFs in the soot inception zone of the flames (i.e., HAB < 15 mm for the considered flame), at least for sizes below 10 nm, because coagulation of particles smaller than 3 nm affect the SDF shape even in a larger dimensional range.
When the highest dilution is used in the low residence time flow configuration (B), the SDFs appear to be monomodal at the lower sampling positions up to HAB = 10 mm, with the peak number concentration located at D < 2 nm. The second mode has a lognormal distribution. It becomes more predominant and shifts to increasingly larger sizes by either lowering the dilution at each sampling position or sampling further downstream in the flame (larger HAB) for a fixed DR. All these features confirm that the second mode is due to coagulation of soot nuclei from the first mode.
Soot nuclei smaller than 3 nm appear to acquire preferentially a positive charge also in the unconventional B configuration, so that a direct measurement of the charging probability of soot nuclei is mandatory for determining their absolute number concentration in the flame. The number concentration inferred by measurements of positively charged particles exceeds that of the negatively charged ones by a factor larger than 3 for D between 1 nm and 3 nm. In contrast, the SDFs in for particles with opposite polarities are almost identical for D > 3 nm, as already noted in the discussion of
4.3. Naturally charged particles
Particles naturally have a charging probability in the flame either because they directly nucleate through molecular ion reactions, as hypothesized by Calcote (Citation1981, Citation1994), or because they are diffusively and thermally ionized after nucleating via neutral molecular reaction mechanisms (Frenklach and Wang Citation1991, 1994; D'Anna Citation2009; Wang Citation2011). Diffusion charging certainly occurs in the flame since chemi-ions are formed with concentrations as high as 109–1012 cm−3 in the flame front (Lawton and Weinberg Citation1969; Calcote Citation1981). If the characteristic time for diffusion charging in the flame is comparable to that for soot nucleation, observation of the flame charging probability in the size range below 3 nm would give insight in the soot inception stages.
Measurements of particles that naturally acquire a charge when sampled from the flame were performed using the short residence time flow configuration after removing the radioactive neutralizer (configuration C in ). In this configuration, the measured SDF at the DMA inlet slit may differ considerably from the one that charged particles originally had in the flame at the sampling position because diffusion charging continues at low temperature in the sampling system through the concurrent flame ion sampling. Going back to , we showed the Boltzmann equilibrium charge probability that would be achieved for an infinite time and in the absence of not-equilibrium ion sources at the flame and at the probe temperatures, respectively. Experimentally freezing the original flame charge state of particles in the flame is especially difficult because of the high concentrations of the concurrently sampled flame ions, calling for even higher DR/Δt values than in configurations A and B. To complicate things further, the lower signal associated to the lower concentration of naturally charged particles limits the use of extremely high dilution and DMA resolutions.
The measured SDFs of naturally charged flame-sampled particles are shown in . Data were processed with Equation Equation(5)[5] by neglecting the ηCharg correction to account only for charged particles. In the set of measurements with the C flow configuration, four sampling dilutions were used to match some of the DR/Δt ratios used in the A and B configurations ( and and ). Measurements performed in particle-free nitrogen and at HAB = 0 mm showed that the signals did not exceed the noise level.
Figure 7. SDFs of particles that are naturally charged when sampled from the flame and carrying either a positive (left column) or a negative (right column) charge (configuration C in ). Measurements were performed at different HABs with DR/Δt varying by a factor of 10.
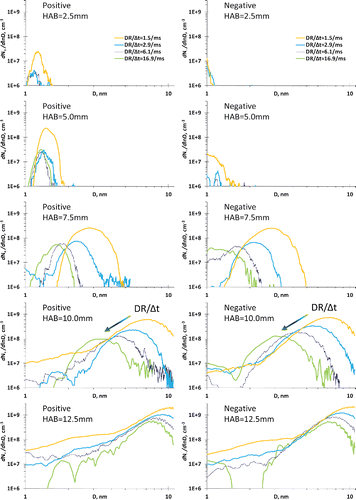
Results show that naturally and positively charged particles/clusters with mobility diameters around 1.2–1.4 nm appear in the flame already at HAB = 2.5, whereas the signal measured for negative polarity is appreciable only at the sub-nanometer sizes associated with gaseous ions. The peaks are consistent with the signal in excess of the background values observed at these sampling positions using the A and B configurations. The asymmetry in polarity for naturally charged particles is also consistent with results in and . Indeed, a greater presence of positively charged particles between 1 nm and 3 nm clearly persists at least up to HAB = 5 mm. The consistency of the SDF peak positions in – suggests that the specific charging mechanisms of soot nuclei are not severely affecting their sizes. The SDFs of naturally charged particles larger than 3 nm detected downstream in the flame have instead an almost polarity symmetric charge state and approach a lognormal shape, implying coagulation from smaller nuclei with the consequent charge equilibration (Maricq Citation2008). The data indicate that a critical value of DR/Δt was reached to quench the evolution of naturally charged particles and to infer the actual SDF of charged particles in the flame only at HAB ≤ 5 mm (for DR/Δtcritical≥ 2.9), where they have the lowest number concentrations (below 105 cm−3 in the probe after dilution).
The most interesting and surprising aspect of the data in is the variation of the measured SDFs of naturally charged particles as the sampling dilution is lowered below the critical value. The lowering in dilution involves the anticipated increase in particle size and the polarity symmetrization of the particle SDFs that are evident at HAB = 7.5 mm, because of enhanced coagulation following the higher concentration levels in the sampling system. There is a surprising increase in their number concentration that can go up to approximately 4 107 cm−3 in the probe (corresponding to 1 109 cm−3 in the flame). This behavior is observed at all sampling positions, applies to both polarities, and is not vitiated by measurements uncertainties. This finding cannot be explained by coagulation effects in the sampling system, since coagulation decreases the total particle number concentrations, as well as that of charged particles by charge recombination. Furthermore, it cannot be straightforwardly rationalized as a consequence of the diffusion charging effects in the sampling line if the intuitive view, suggested by the equilibrium charging probabilities in , that the fraction of charged particles is larger in the flame than in the sampling line is true.
There are two nonmutually exclusive hypotheses that may rationalize this behavior—the first consistent with a neutral soot nucleation pathway, and the other partially involving ion-mediated soot nucleation. One may assume, perhaps counterintuitively, that the particle charging probability at high temperature in the flame is lower than the one at low temperature in the sampling system, so that diffusional charging in the probe by flame sampled ions is enhanced with dilution lowering below the critical value. If this were the case, initially neutral particles would be diffusively charged by collisions with extremely short lived and small ions whose charge would not otherwise be able to reach the DMA inlet slit. Such an anomalous low charging probability of particles in the flame, requiring diffusional charging rates much slower than the soot nucleation rate, would need to be experimentally tested and/or theoretically rationalized. An alternative possible explanation for the increasing naturally charged particle concentration with decreasing dilution is the direct nucleation of charged particles in the sampling line, which is ever more effective with the lowering of dilution, much the same way as a heterogeneous condensation process of neutral molecules over small ions (Winkler et al. Citation2008) whose charge would not otherwise be able to reach the DMA inlet slit because of their extremely short lifetime. Results indicate that such a nucleation mechanism would initially occur on positive ions at HAB ≤ 5 mm and would still be active far downstream in the flame (i.e., at least up to HAB = 12.5 mm). This second hypothesized mechanism, if active also in the flame, may eventually explain the condensation of otherwise volatile species at flame temperature (Elvati and Violi Citation2013). Additionally, it would not require the assumption of a peculiar charge probability of particles in the flame. We highlight that the two, possibly concurrent, hypothesized mechanisms for the number density increase in one case require molecular ions colliding with neutral nuclei and in the other case neutral molecules colliding and condensing on ionic nuclei before small ions are lost by recombination and/or wall losses. The presence of free electron in the flame may rationalize the initial charge asymmetry in particle nucleation since electron collisions with molecules are in most cases followed by fragmentation. Additional work is needed to test these hypotheses.
To shed some light on the natural charging mechanisms, we compared measurement in with those shown in and for the same DR/Δts, specifically DR/Δt = 1.5 ms−1 from and DR/Δt of 6.1 ms−1 and 16.9 ms−1 from . Considering that in Equation Equation(5)[5] the SDFs of all particles (neutral and charged) is fixed at a given sampling position, the ratios of the two SDF measurement sets is equal to the charging probability achieved in the C configuration, ηCharg(C), that is, Cion(C) upon normalization over ηWied. In determining the SDF ratios, datasets were truncated at D below 1.4 nm for positively charged particle and below 1.2 nm for negatively charged ones, to avoid unquantifiable uncertainties associated with the nitrogen background signal, as previously discussed. For this reason, data at HAB below 5 mm are not presented. The resulting SDF of Cion(C) are affected by the assumption of Cion(A) = 1 for data at DR/Δt = 1.5 ms−1 and of Cion(B) = 0.18 for the other two datasets, as discussed earlier. The charging probability in the flame is equal to Cion(C) ηWied when DR/Δt is large enough to achieve the asymptotic regime freezing both charge and aerosol evolutions in the sampling system.
The SDFs of Cion(C) are shown in with the data presented for decreasing values of DR/Δt to highlight variations in the charging mechanisms that are enhanced in the probe at lower dilutions. The critical dilution to determine the charge status in the flame was not achieved at HAB ≥ 7.5 mm. As limiting cases, also shows the ratio of the equilibrium charge distribution at the flame and probe temperatures over ηWied. If one assumes that the flame generated ions have the sizes of the SDF at HAB ≤ 5 mm in (sizes close to that of the ions generated in the radioactive chargers) the unity value, CWied in , approximates also the steady-state charging probability at ambient temperature due to flame-generated ions. It is not possible to perform a parametric simulation of Cion(C) because the total number concentration of flame ions cannot be determined at each HAB since they quickly disappear in the probe as their charge recombine and/or is transferred to larger particles.
Figure 8. Ratio (Cion in Equation Equation(6)[6] ) of the natural charging probability obtained in the C configuration of over the Wiendsholer (1988) approximation, ηWied, of the steady-state charge probability. Cion is estimated by the ratio of the SDFs in divided by the ones at the same DR/Δt in (for DR/Δt = 1.5 ms−1) and in (for DR/Δts equal to 6.1 ms−1 and 34 ms−1). The ratios of by the equilibrium charge distributions, Cequil, at the flame and probe temperatures and of ηWied itself, CWied = 1, are also shown as asymptotic cases.
![Figure 8. Ratio (Cion in Equation Equation(6)[6] ) of the natural charging probability obtained in the C configuration of Figure 3 over the Wiendsholer (1988) approximation, ηWied, of the steady-state charge probability. Cion is estimated by the ratio of the SDFs in Figure 7 divided by the ones at the same DR/Δt in Figure 5 (for DR/Δt = 1.5 ms−1) and in Figure 6 (for DR/Δts equal to 6.1 ms−1 and 34 ms−1). The ratios of by the equilibrium charge distributions, Cequil, at the flame and probe temperatures and of ηWied itself, CWied = 1, are also shown as asymptotic cases.](/cms/asset/401ffa9e-d211-4df8-a7c2-f50d27240749/uast_a_1179715_f0008_oc.gif)
At the highest DR/Δt of 16.9 ms−1 the SDF of Cion(C) gives the best available experimental approximation of the charge probability in the flame. In this case, if we consider the three HABs, Cion(C) grows gradually from approximately 10−2 for D = 2 nm to approximately 10−1 for D = 10.84 nm as the values of the natural charging probability moves from values close to equilibrium at the flame temperature to the one of equilibrium at the probe temperature. This finding suggests that for this condition the smallest particles may get their charge directly in the flame, whereas the larger ones readjust their charge state as they are sampled. Further increase in DR/Δt would be necessary to corroborate this hypothesis. As DR/Δt is lowered to 6.1 ms−1 the SDFs of Cion(C) varies because of a noticeable increase in the values for D < 3 nm and for D > 5 nm. Such a localized increase may be caused by continuous ion-induced nucleation in the smallest size range and more effective diffusional charging in the larger size range. It also brings out a dip in the SDFs of Cion(C). At the lowest DR/Δt = 1.5 ms−1 the size-selective increase of Cion(C) is so marked that the dip in most cases becomes a well visible minimum whose position moves toward larger sizes, as the average size of detected particles increases downstream in the flame. At each HAB the largest detected particles approximately reach the steady-state charge state, i.e., Cion(C) = 1, supporting the concept that the increase of Cion(C) for the largest particles as DR/Δt is lowered, maybe caused by diffusional charging.
In closing, a reexamination of all the data shown in – shows that the behavior of SDFs when lowering DR/Δt is similar to that observed by moving downstream in the flame, suggesting a similarity between the mechanisms active in the probe and in the flame.
5. Conclusions
Nascent soot particles were measured at several positions in a moderately sooting premixed flame, to investigate measurement and data inversion artifacts possibly affecting DMA results in the size range (≤10 nm) where the transition from gas to particle phase occurs. High-resolution DMA measurements were performed using rapid-dilution probes. They yielded particle SDFs with charge acquired either naturally or diffusively by exposure to either a bi-polar radioactive charger or by dilution with a nitrogen flow seeded with ions. Results were obtained for different dilution ratios and residence times in the sampling and charging system, yielding the conclusions listed below.
The critical value of the ratio of dilution over residence time for suppressing particle coagulation was not achieved under most conditions, despite efforts to minimize the residence time in the sampling line. The value of this ratio in the present study largely exceeds values used in similar flame studies in which this goal had been purportedly reached. As a result, the possible identification of this “asymptotic” regime, which is critical to determine the parent SDFs and charge probability of particle in the soot inception region of the flame, undoubtedly requires further work with high-resolution DMAs and nonconventional configurations of the sampling and charging system.
Soot nuclei smaller than 3 nm preferentially acquire a positive charge so that a direct measurement of their charging probability is mandatory for determining with accuracy their absolute number concentration in the flame.
For D larger than 3 nm, the SDFs obtained from measurements in opposite polarities are almost indistinguishable and have a lognormal shape, implying that particles with size between 3 nm and 11 nm are generated by coagulation of the smaller nuclei. Coagulation of nuclei must be suppressed to correctly measure also particles larger than 3 nm, in the soot inception zone of the flame.
Measurements of naturally charged particles evidence an unexpected increase in their number concentrations as dilution is lowered. Hypothesized culprits for this surprising finding, subject to future study, include: (a) the possibility that the particle charging probability is higher at room temperature than in the flame, allowing diffusive charging in the probe of initially neutral particles by flame sampled ions; and/or (b) the possibility that some of the observed nuclei are formed by heterogeneous condensation of rapidly cooled flame vapors on flame ions (ion-induced nucleation), with an initial prevalence of the positive polarity.
The behavior of the SDFs when lowering DR/Δt is similar to that observed by moving downstream in the flame, suggesting similarities between the mechanisms active in the sampling/charging system and at high temperature in the flame.
Nomenclature
Ccone | = | mobility correction factor due to the conical shape of the DMA inner electrode |
Cion | = | transient charging correction factor of the steady-state charging probability |
D | = | mobility diameter |
D0 | = | effective size of the transport gas molecules to correct the mobility diameter |
DR | = | standard volumetric dilution ratio |
DTHA+ | = | mobility diameter of the tetraheptyl ammonium ion monomer |
e | = | elementary charge |
HAB | = | height above the burner |
IFC | = | current measured in the Faraday cup electrometer |
L | = | nominal length of the DMA classification section between inlet and outlet slit |
n+ | = | number concentration of positive ions |
n- | = | number concentration of negative ions |
N | = | total particle number concentration |
Np | = | number concentration of particle carrying p elementary charges |
= | diffusional penetration efficiency from the DMA outlet slit to the Faraday cup | |
= | diffusional penetration efficiency from the sampling orifice to the DMA inlet slit | |
P(Z, Z′) | = | transfer function of the DMA classification section |
QDMA | = | flowrate of the aerosol crossing the DMA classification section |
Qsh | = | sheath flowrate crossing the DMA closed loop and classification section |
Rin | = | nominal radius of the DMA internal electrode at the DMA outlet slit |
Rout | = | nominal radius of the DMA external electrode |
TDMA | = | aerosol temperature at the DMA inlet slit |
Tflame | = | flame temperature |
VDMA | = | voltage applied to the DMA classification section electrodes |
VFC | = | voltage measured over the electrical resistance of the Faraday cup electrometer |
VTHA+ | = | electrostatic classifier voltage to detect the tetraheptyl ammonium ion monomer |
ZTHA+ | = | electrical mobility of the tetraheptyl ammonium ion monomer |
α | = | ion recombination rate coefficient |
q | = | ion production rate in the radioactive source |
Δt | = | residence time of the aerosol from the sampling orifice to the DMA inlet slit |
ηCharg | = | charging probability |
ηWied | = | approximation of the steady-state diffusional charging probability |
= | attachment coefficient of positive ions to particles carrying p elementary charges | |
= | attachment coefficient of negative ions to particles carrying p elementary charges |
UAST_1179715_Supplementary_File.zip
Download Zip (22.9 KB)Acknowledgments
The authors gratefully acknowledge Professor Juan Fernandez de la Mora for much advice and fruitful discussions, in addition to lending the Half-Mini DMA system, Professor Marshal Long for the loan of the honeycomb burner, and Mr. Nicholas Bernardo for machining some components of the experimental system. One of the authors (FC) acknowledges many discussions with the late Professor Ninni D'Alessio that stimulated much of his thinking in nascent soot.
Funding
Financial support for this work was provided by the USA National Science Foundation (Grant #CBET-1233318, Dr. Ruey-Hung Chen, Program Director) and the U.S. Army Research Office (Grant #W911NF-14-1-0264, Dr. Ralph A. Anthenien, Program Director).
References
- Abid, A. D., Heinz, N., Tolmachoff, E. D., Phares, D. J., Campbell, C. S., and Wang, H. (2008). On Evolution of Particle Size Distribution Functions of Incipient Soot in Premixed Ethylene–Oxygen–Argon Flames. Combust. Flame, 154:775–788.
- Abid, A. D., Camacho, J., Sheen, D. A., and Wang, H. (2009b). Quantitative Measurement of Soot Particle Size Distribution in Premixed Flames–The Burner-Stabilized Stagnation Flame Approach. Combust. Flame, 156:1862–1870.
- Abid, A. D., Tolmachoff, E. D., Phares, D. J., Wang, H., Liu, Y., and Laskin, A. (2009a). Size Distribution and Morphology of Nascent Soot in Premixed Ethylene Flames with and without Benzene Doping. Proc. Combust. Inst., 32:681–688.
- Adachi, M., Kousaka, Y., and Okuyama, K. (1985). Unipolar and Bipolar Diffusion Charging of Ultrafine Aerosol Particles. J. Aerosol Sci., 16:109–123.
- Alonso, M., and Alguacil, F. J. (2003). The Effect of Ion and Particle Losses in a Diffusion Charger on Reaching a Stationary Charge Distribution. J. Aerosol Sci., 34:1647–1664.
- Alonso, M., Hashimoto, N., Kousaka, Y., Higuchi, M., and Nomura, T. (1998). Transient Bipolar Charging of a Coagulating Nanometer Aerosol. J. Aerosol Sci., 29:263–270.
- Alonso, M., Kousaka, Y., Nomura, T., Hashimoto, N., and Hashimoto, T. (1997). Bipolar Charging and Neutralization of Nanometer-Sized Aerosol Particles. J. Aerosol Sci., 28:1479–1490.
- Bockhorn, H., D'Anna, A., Sarofim, A. F., and Wang, H. eds. (2009). Combustion Generated Fine Carbonaceous Particles. KIT Scientific, Karlsruhe.
- Burtscher, H. (1992). Measurement and Characteristics of Combustion Aerosols with Special Consideration of Photoelectric Charging and Charging by Flame Ions. J. Aerosol Sci., 23:549–595.
- Calcote, H. F. (1981). Mechanisms of Soot Nucleation in Flames-Critical Review. Combust. Flame, 42:215–242.
- Calcote, H. F., and Gill R. J. (1994). Comparison of the Ionic Mechanism of Soot Formation with a Free Radical Mechanism, in Soot Formation in Combustion-Mechanism and Models. Bockhorn, H. ed, Springer Verlag, Berlin, pp. 471–484.
- Camacho, J., Lieb, S., and Wang. H. (2013). Evolution of Size Distribution of Nascent Soot in n- and i-Butanol Flames. Proc. Combust. Inst., 34:1853–1860.
- Camacho, J., Liu, C., Gu, C., Lin, H., Huang, Z., Tang, Q., You, X., Saggese, C., Li, Y., Jung, H., Deng, L., Wlokas, I., and Wang H. (2015). Combust. Flame, 162:3810–3822.
- Carbone, F. (2010). Physicochemical Characterization of Combustion Generated Inorganic Nanoparticles, Ph.D. Dissertation. Fredericiana Editrice Universitaria, Naples, IT.
- Carbone, F., Beretta, F., and D'Anna, A. (2010). Size Distribution Functions of Ultrafine Ashes from Pulverized Coal Combustion. Combust. Sci. Tech., 182:668–682.
- Carbone, F., Beretta, F., and D'Anna, A. (2011). A Flat Premixed Flame Reactor to Study Nano-Ash Formation during High Temperature Pulverized Coal Combustion and Oxygen Firing. Fuel, 90:369–375.
- Carbone, F., Cattaneo, F., and Gomez, A. (2015). Structure of Incipiently Sooting Partially Premixed Ethylene Counterflow Flames. Combust. Flame, 162:4138–4148.
- Carbone, F., and Gomez, A. (2012). The Structure of Toluene-Doped Counterflow Gaseous Diffusion Flames. Combust. Flame, 159:3040–3055.
- Carbone, F., and Gomez, A. (2013). Chemical Effects of 1,2,4-Trimethyl Benzene Addition in Counterflow Gaseous Diffusion Flames. Proc. Combust. Inst., 34:1025–1033.
- D'Anna, A. (2009). Combustion-Formed Nanoparticles. Proc. Combust. Inst., 32:593–613.
- De Filippo, A., Sgro, L. A., Lanzuolo, G., and D'Alessio, A. (2009). Probe Measurements and Numerical Model Predictions of Evolving Size Distributions in Premixed Flames. Combust. Flame, 156:1744–1754.
- de La Verpilliere, J. L., Swanson, J. J., and Boies, A. M. (2015). Unsteady Bipolar Diffusion Charging in Aerosol Neutralisers: A Non-Dimensional Approach to Predict Charge Distribution Equilibrium Behavior. J. Aerosol Sci., 86:55–68.
- Echavarria, C. A., Jaramillo, I. C., Sarofim, A. F., and Lighty, J. S. (2011b). Studies of Soot Oxidation and Fragmentation in a Two-Stage Burner under Fuel-Lean and Fuel-Rich Conditions. Proc. Combust. Inst., 33:659–666.
- Echavarria, C. A., Sarofim, A. F., Lighty, J. S., and D'Anna, A. (2009). Modeling and Measurements of Size Distributions in Premixed Ethylene and Benzene Flames. Proc. Combust. Inst., 32:705–711.
- Echavarria, C. A., Sarofim, A. F., Lighty, J. S., and D'Anna, A. (2011a). Sooting Behaviors of n-Butanol and n-Dodecane Blends. Combust. Flame, 158:98–104.
- Elvati, P., and Violi, A. (2013). Thermodynamics of Poly-Aromatic Hydrocarbon Clustering and the Effects of Substituted Aliphatic Chains. Proc. Combust. Inst., 34:1837–1843.
- Fang, J., Wang, Y., Attoui, M., Chadha, T. S., Ray, J. R., Wang, W. N., Jun, Y. S., and Biswas, P. (2014). Measurement of Sub-2 nm Clusters of Pristine and Composite Metal Oxides during Nanomaterial Synthesis in Flame Aerosol Reactors. Anal. Chem., 86:7523–7529.
- Fernandez de la Mora, J. (2011a). Electrical Classification and Condensation Detection of Sub-3-nm Aerosols, in Aerosol Measurement: Principles, Techniques, and Applications, 3rd ed., P. Kulkarni, P. A. Baron, and K. Willeke, eds., John Wiley, Hoboken, NJ, pp. 697–722.
- Fernandez de la Mora, J. (2011b). Ionization of Vapor Molecules by an Electrospray Cloud. Int. J. Mass Spec., 300:182–193.
- Fernandez de la Mora, J., de Juan, L., Liedtke, K., and Schmidt-Ott, A. (2003). Mass and Size Determination of Nanometer Particles by Means of Mobility Analysis and Focused Impaction. J. Aerosol Sci., 34:79–98.
- Fernandez de la Mora, J., and Kozlowski, J. (2013). Hand-Held Differential Mobility Analyzers of High Resolution for 1–30 nm Particles: Design and Fabrication Considerations. J. Aerosol Sci., 57:45–53.
- Fernandez Garcia, J., and Fernández de la Mora, J. (2013). Measuring the Effect of Ion-Induced Drift-Gas Polarization on the Electrical Mobilities of Multiply-Charged Ionic Liquid Nanodrops in Air. J. Am. Soc. Mass Spec., 24:1872–1889.
- Figura, L., Carbone, F., and Gomez, A. (2015). Challenges and Artifacts of Probing High-Pressure Counterflow Laminar Diffusion Flames. Proc. Combust. Inst., 35:1871–1878.
- Figura, L., and Gomez, A. (2015). Structure of Incipiently Sooting Ethylene–Nitrogen Counterflow Diffusion Flames at High Pressures. Combust. Flame., 161:1587–1603.
- Flagan, R. C. (1999). On Differential Mobility Analyzer Resolution. Aerosol Sci. Tech., 30:556–570.
- Flagan, R. C. (2011). Electrical Mobility Methods for Submicrometer Particle Characterization, in Aerosol Measurement: Principles, Techniques, and Applications, 3rd ed., P. Kulkarni, P. A. Baron, and K. Willeke, K. , eds., John Wiley, Hoboken, NJ, pp. 339–364.
- Flagan, R. C. (2014). Continuous-Flow Differential Mobility Analysis of Nanoparticles and Biomolecules. Annu. Rev. Chem. Biomol. Eng., 5:255–279.
- Frenklach, M., and Wang, H. (1991). Detailed Modeling of Soot Particle Nucleation and Growth. Proc. Combust. Inst., 23:1559–1566.
- Friedlander, S. K. (2000). Smoke, Dust, and Haze: Fundamentals of Aerosol Dynamics. Oxford University Press, New York.
- Fuchs, N. A. (1963). On the Stationary Charge Distribution on Aerosol Particles in a Bipolar Ionic Atmosphere. Geofis. Pura Appl., 56:185–193.
- Ghaffarpasand, O., Drewnick, F., Hosseiniebalam, F., Gallavardin, S., Fachinger, J., Hassanzadeh, S., and Borrmann, S. (2012). Penetration Efficiency of Nanometer-Sized Aerosol Particles in Tubes under Turbulent Flow Conditions. J. Aerosol Sci., 50:11–25.
- Ghiassi, H., Toth, P., and Lighty, J. S. (2014). Sooting Behaviors of n-Butanol and n-Dodecane Blends. Combust. Flame, 161:671–679.
- Glassman, I., and Yetter, R. (2008). Combustion. 4th ed. Academic Press, Burlington, MA.
- Gopalakrishnan, R., and Hogan, C. J. (2012). Coulomb-Influenced Collisions in Aerosols and Dusty Plasmas. Phys. Rev. E., 85:026410.
- Gopalakrishnan, R., McMurry, P. H., and Hogan, Jr., C. J. (2015). The Bipolar Diffusion Charging of Nanoparticles: A Review and Development of Approaches for Non-Spherical Particles. Aerosol Sci. Tech., 49:1181–1194.
- Gopalakrishnan, R., Meredith, M. J., Larriba-Andaluz, C., and Hogan, Jr. C. J. (2013). Brownian Dynamics Determination of the Bipolar Steady-State Charge Distribution on Spheres and Non-Spheres in the Transition Regime. J. Aerosol Sci., 63:126–145.
- Haynes, B. S., and Wagner, H. G. G. (1981). Soot Formation. Prog. Energy Combust. Sci., 7:229–273.
- Hinds, W. C. (1999). Aerosol Technology—Properties, Behavior and Measurement of Airborne Particles. John Wiley, Hoboken, NJ.
- Hoppel, W., and Frick, G. (1990). The Nonequilibrium Character of the Aerosol Charge-Distributions Produced by Neutralizers. Aerosol Sci. Technol., 12(3):471–496.
- Hoppel, W. A., and Frick, G. M. (1986). Ion-Aerosol Attachment Coefficients and the Steady-State Charge Distribution on Aerosols in a Bipolar Ion Environment. Aerosol Sci. Tech., 5:1–21.
- Hoppel, W. A., and Frick, G. M. (1988). Aerosol Charge Distributions Produced By Radioactive Ionizers. Naval Research Laboratory, NRL Report 9108. Naval Research Laboratory, Washington, DC.
- Jiang, J., Attoui, M., Heim, M., Brunelli, N. A., McMurry, P. H., Kasper, G., Flagan, R. C., Giapis, K., and Mouret, G. (2011). Transfer Functions and Penetrations of Five Differential Mobility Analyzers for Sub-2 nm Particle Classification. Aerosol Sci. Technol., 45:480–492.
- Jiang, J., Kim, C., Wang, X., Stolzenburg, M. R., Kaufman, S. L., Qi, C., Sem, G. J., Sakurai, H., Hama, N., and McMurry, P. H. (2015). Aerosol Charge Fractions Downstream of Six Bipolar Chargers: Effects of Ion Source, Source Activity, and Flowrate. Aerosol Sci. Technol., 48:1207–1216.
- Kasper, M., Siegmann, K., and Sattler, K. (1997). Evaluation of an In Situ Sampling Probe for Its Accuracy in Determining Particle Size Distributions from Flames. J. Aerosol Sci., 28:1569–1578.
- Knutson, E. O., and Whitby, K. T. (1975). Aerosol Classification by Electric Mobility: Apparatus, Theory and Applications. J. Aerosol Sci., 6:443–451.
- Kumar, P., Fennell, P., Symonds, J., and Britter, R. (2008). Treatment of Losses of Ultrafine Aerosol Particles in Long Sampling Tubes during Ambient Measurements. Atmosph. Env., 42:8819–8826.
- Larriba, C., and Hogan, C. J. (2013). Ion Mobilities in Diatomic Gases: Measurement vs. Prediction with Non-Specular Scattering Models. J. Phys. Chem. A., 117:3887–3901.
- Larriba, C., Hogan, C. J., Attoui, M., Borrajo, R., Fernandez Garcia, J., and Fernandez de la Mora, J. (2011). The Mobility-Volume Relationship Below 3.0 nm Examined by Tandem Mobility-Mass Measurement. Aerosol Sci. Technol., 45:453–467.
- Lawton, J., and Weinberg, F. J. (1969). Electrical Aspects of Combustion, Oxford University Press, New York.
- Liu, B. Y. H., and Pui, D. Y. H. (1974). Electrical Neutralization of Aerosols. J. Aerosol Sci., 5:465–472.
- Lopez-Yglesias, X., and Flagan, R. C. (2013). Ion–Aerosol Flux Coefficients and the Steady-State Charge Distribution of Aerosols in a Bipolar Ion Environment. Aerosol Sci. Technol., 47:688–704.
- Manzello, S. L., Lenhert, D. B., Yozgatligil, A., Donovan, M. T., Mulholland, G. W., Zachariah, M. R., and Tsang, W. (2007). Soot Particle Size Distributions in a Well-Stirred Reactor/Plug Flow Reactor. Proc. Combust. Inst., 31:675–683.
- Maricq, M. M. (2004). Size and Charge of Soot Particles in Rich Premixed Ethylene Flames. Combust. Flame, 137:340–350.
- Maricq, M. M. (2005). The Dynamics of Electrically Charged Soot Particles in a Premixed Ethylene Flame. Combust. Flame, 141:406–416.
- Maricq, M. M. (2006). A Comparison of Soot Size and Charge Distributions from Ethane, Ethylene, Acetylene, and Benzene/Ethylene Premixed Flames. Combust. Flame, 144:730–743.
- Maricq, M. M. (2007). Coagulation Dynamics of Fractal-Like Soot Aggregates. J. Aerosol Sci., 38(2007):141–156.
- Maricq, M. M. (2008). Thermal Equilibration of Soot Charge Distributions by Coagulation. J. Aerosol Sci., 39:141–149.
- Maricq, M. M., Harris, S. J., and Szente, J. J. (2003). Soot Size Distributions in Rich Premixed Ethylene Flames. Combust. Flame, 132:328–342.
- Reischl, G. P., Mäkelä, J. M., Karch, R., and Necid, J. (1996). Bipolar Charging of Ultrafine Particles in the Size Range Below 10 nm. J. Aerosol Sci., 27:931–949.
- Rosell-Llompart, J., Loscertales, I. G., Bingham, D., and de la Mora, J. F. (1996). Sizing Nanoparticles and Ions with a Short Differential Mobility Analyzer. J. Aerosol Sci., 27:695–719.
- Sgro, L. A., D'Anna, A., and Minutolo, P. (2010). Charge Distribution of Incipient Flame-Generated Particles. Aerosol Sci. Technol., 44:651–662.
- Sgro, L. A., D'Anna, A., and Minutolo, P. (2011). Charge Fraction Distribution of Nucleation Mode Particles: New Insight on the Particle Formation Mechanism. Combust. Flame, 158:1418–1425.
- Sgro, L. A., De Filippo, A., Lanzuolo, G., and D'Alessio, A. (2007). Characterization of Nanoparticles of Organic Carbon (NOC) Produced in Rich Premixed Flames by Differential Mobility Analysis. Proc. Combust. Inst., 31:631–638.
- Steiner, G., Jokinen, T., Junninen, H., Sipilä, M., Petäjä, T., Worsnop, D., Reischl, G. P., and Kulmala, M. (2014). High-Resolution Mobility and Mass Spectrometry of Negative Ions Produced in a 241 Am Aerosol Charger. Aerosol Sci. Technol., 48:261–270.
- Stolzenburg, M. R. (1988). An Ultrafine Aerosol Size Distribution Measuring System, Ph.D. Thesis, University of Minnesota.
- Stolzenburg, M. R., and McMurry, P. H. (2008). Equations Governing Single and Tandem DMA Configurations and a New Lognormal Approximation to the Transfer Function. Aerosol Sci. Technol., 42:421–432.
- Stommel, Y. G., and Riebel, U. (2007). Comment on the Calculation of the Steady-State Charge Distribution on Aerosols < 100 nm by Three Body Trapping Method in a Bipolar Ion Environment. Aerosol Sci. Technol., 41:840–847.
- Tammet, H. (1995). Size and Mobility of Nanometer Particles, Clusters, and Ions. J. Aerosol Sci., 26:459–475.
- Tigges, L., and Schmid, H. J. (2015). On the Bipolar Charge Distribution Used for Mobility Particle Sizing: Theoretical Considerations. J. Aerosol Sci., 88:119–134.
- Ude, S., and Fernandez de la Mora, J. (2005). Molecular Monodisperse Mobility and Mass Standards from Electrosprays of Tetra-Alkyl Ammonium Halides. J. Aerosol Sci., 36:1224–1237.
- Wang, H. (2011). Formation of Nascent Soot and Other Condensed-Phase Materials in Flames. Proc. Combust. Inst., 33:41–67.
- Wang, Y., Fang, J., Attoui, M., Chadha, T. S., Wang, W. N., and Biswas, P. (2014). Application of Half Mini DMA for Sub 2 nm Particle Size Distribution Measurement in an Electrospray and a Flame Aerosol Reactor. J. Aerosol Sci., 71:52–64.
- Wiedensohler, A. (1988). An Approximation of the Bipolar Charge Distribution for Particles in the Submicron Size Range. J. Aerosol Sci., 3:387–389.
- Wiedensohler, A., Lutkemeier, E., Feldpausch, M., and Helsper, C. (1986). Investigation of the Bipolar Charge-Distribution at Various Gas Conditions. J. Aerosol Sci., 17(3):413–416.
- Winkler, P. M., Steiner, G., Vrtala, A., Vehkamäki, H., Noppel, M., Lehtinen, K. E. J., Reischl, G. P., Wagner, P. E., and Kulmala, M. (2008). Heterogeneous Nucleation Experiments Bridging the Scale from Molecular Ion Clusters to Nanoparticles. Science, 319:1374–1377.
- Winklmayr, W., Reischl, G. P., ·Lindner, A. O., and Bemer, A. (1991). A New Electromobility Spectrometer for the Measurement of Aerosol Size Distributions in the Size Range from 1 to 1000 nm. J. Aerosol Sci., 22:289–296.
- Zhao, B., Yang, Z., Wang, J., Johnston, M. V., and Wang, H. (2003a). Measurement and Numerical Simulation of Soot Particle Size Distribution Functions in a Laminar Premixed Ethylene-Oxygen-Argon Flame. Aerosol Sci. Technol., 37:611–620.
- Zhao, B., Yang, Z., Wang, J., Johnston, M. V., and Wang, H. (2003b). Analysis of Soot Nanoparticles in a Laminar Premixed Ethylene Flame by Scanning Mobility Particle Sizer. Aerosol Sci. Technol., 37:611–620.
- Zhao, B., Yang, Z., Wang, J., Johnston, M. V., and Wang, H. (2005). Particle Size Distribution Function of Incipient Soot in Laminar Premixed Ethylene Flames: Effect of Flame Temperature. Proc. Combust. Inst., 30:1441–1448.