ABSTRACT
A method of long-lasting TiO2 nanoparticle generation was tested for use in follow-up studies of the health impacts of nanoparticles on laboratory animals during several weeks' long exposure experiments. Nanoparticles were synthesized in an externally heated tube reactor by pyrolysis and oxidation of titanium tetraisopropoxide. Particle production was studied under varying reactor temperature, reactor flow rate, and precursor vapor pressure. A total of 264 h of particle generation were performed in four experimental campaigns using one batch of precursor without an observable decrease of particle production. As a result, particle production with number concentrations high above 1.0 × 107 #/cm3 and with primary particle sizes well below 50 nm could be achieved in most of the investigated experimental conditions. Maximum of particle mass concentration reached the value 9500 μg/m3, which corresponds with emission rate 29 μg/min. The dependence of nanoparticle production and characteristics on experimental conditions was evaluated and the most suitable parameters for exposure experiments were specified. A comparison of the results with data from the literature shows that the material of the reactor plays a significant role in the process of particle formation.
Copyright © 2016 American Association for Aerosol Research
EDITOR:
1. Introduction
Nanoparticles (NPs) have attracted considerable attention because they show a quantum size effect, for which the physical and chemical properties are strongly dependent on the particle size (Nakaso et al. Citation2003). This change of properties can also affect their toxicity, therefore, they cannot be treated the same way as bulk material. Some materials can become toxic if they are inhaled in the form of nanoparticles. When inhaled, nanoparticles can be deposited in the lungs, with the potential to move to other organs, such as the brain, liver, spleen, etc. In spite of that, studies of allocation of inhaled NPs are still rather rare (Večeřa et al. Citation2011). Recently, an inhalation chamber for the study of the allocation of nanoparticles in organs of laboratory animals was constructed in the Institute of Analytical Chemistry of the Czech Academy of Sciences (Večeřa et al. Citation2011) and some results of inhalation experiments have already been reported (Večeřa et al. Citation2012). For these experiments, it is necessary to continuously generate nanoparticles of the appropriate number concentration and size distribution for the duration of several weeks. Methods for long-term generation of nanoparticles Pb/PbOx (Moravec et al. Citation2015) have been presented recently. The aim of this study was to test the method for long-term generation of TiO2 NPs suitable for subsequent inhalation experiments.
Titania NPs are desired material due to their unique properties and large variety of applications. They are commonly used as pigments and cosmetics, catalyst (Rekoske and Barteau Citation1997) or catalyst supports (Bankmann et al. Citation1992), inorganic membranes (Gu and Oyama Citation2009), photocatalysts for waste water treatments (Ollis et al. Citation1991), gas sensors (Ferroni et al. Citation1996), dye-sensitized solar cells (Grätzel Citation2003), and the surface coatings of paper (Mäkelä et al. Citation2011). Recently, titania NPs were used for exposure experiments (Koivisto et al. Citation2011). The National Institute for Occupational Safety and Health (NIOSH) recommends an airborne exposure limit of 2.4 mg/m3 for respirable pigment-TiO2 and 0.3 mg/m3 for nano-TiO2 as time weighted average concentrations for up to a 10 h per day during a 40-h work week over a whole life time (Koivisto et al. Citation2012). The estimated global production of TiO2 was 4 million metric tons and further increase as well as an increase of a portion on nano-TiO2 is forecasted (Robichaud et al. Citation2009).
Generation of titania NPs in the gas phase has been investigated for a long time. Most frequently, TiO2 NPs were prepared by the gas-to-particle method, either in a flame (Pratsinis et al. Citation1996) or in an externally heated tube reactor (Jang Citation1997; Nakaso et al. Citation2003) by oxidation (Pratsinis et al. Citation1996) or hydrolysis (Jang Citation1997) of TiCl4, or by pyrolysis (Nakaso et al. Citation2003), oxidation (Choi and Park Citation2006), or hydrolysis (Kirkbir and Komiyama Citation1988) of metal organic precursors. Less often, titania NPs were prepared by spray pyrolysis (droplet-to-particle method) using solutions of metalorganic precursors (Ahonen et al. Citation2001; Wang et al. Citation2005). After evaluating these methods with respect to their application in inhalation experiments and based on our earlier experience with synthesis of titania NPs in a moderate temperature glass reactor (Moravec et al. Citation2001), we decided to test the generation of TiO2 NPs by pyrolysis and oxidation of titanium tetraisopropoxide (TTIP). Oxidation was examined with the intention of decreasing the cost of long-term experiments by replacing the part of nitrogen carrier gas with compressed air.
2. Experimental
NPs were synthesized by thermal decomposition of titanium tetraisopropoxide in an inert atmosphere (pyrolysis) or in the mixture of nitrogen and air with a typical oxygen concentration of 12 vol. % (oxidation). The overall stoichiometry of the pyrolysis reaction can be written as follows (Komiyama et al. Citation1984):[1] and for oxidation (Kim et al. Citation1999):
[2]
Vapor pressure of TTIP in the reactor was calculated from the equation (Okuyama et al. Citation1986):[3]
Experiments were performed using the apparatus shown in . Particles were synthesized in an externally heated tube flow reactor with an i.d. of 25 mm and a 1 m-long heated zone. Total length of the work tube, made from impervious aluminous porcelain (IAP), was 1.5 m. The purification unit, used for removing impurities from the carrier gas, consisted of a deoxidizing column and two drying columns. The deoxidizing column (Equation1[1] ) was filled with 5 × 5 mm pellets of Cu catalyst R3-11 (BASF). The first drying column (Equation2
[2] ) was filled with the Dusimo S5 Å molecular sieve and the second with magnesium perchlorate 99% (Fluka, product No. 63102). Two streams of deoxygenated (Equation1
[1] ), dry (Equation2
[2] ), particle-free nitrogen (F) were fed into the saturator (S). The TTIP saturator consists of two chambers. In the first, a stream of nitrogen was saturated by TTIP vapors; in the second the saturated carrier gas was diluted by another stream of N2 to prevent condensation of TTIP in the tubing between saturator outlet and reactor inlet. At the inlet section of the reactor, the TTIP saturated stream was further diluted by a stream of either pure nitrogen (pyrolysis) or filtered and dried air (oxidation). The mixture of gas and particles leaving the reactor was cooled in a diluter by mixing it with an additional stream of air. Flow rates of individual gas streams were controlled with electronic mass flow controllers (Aalborg GFCS), and the temperatures of the saturator and the reactor were controlled with a thermostat and electronic controller Technologic THP 94, respectively.
Figure 1. The scheme of the apparatus: (1) deoxygenator, (2) dryer, (CV) control valve, (D) diluter, (F) filter, (MFC) electronic mass flow controller, (N) aerosol neutralizer Am241, (P) pressure reducing valve, (S) saturator, (SF) sampling filter, and (VP) vacuum pump.
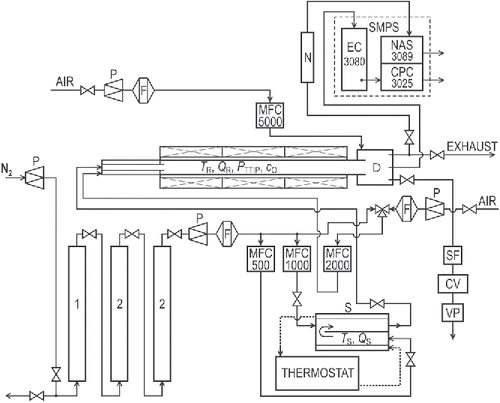
The particle production was monitored by a scanning mobility particle sizer (SMPS, TSI model 3936L25), consisting of electrostatic classifier (TSI model 3080) with long differential mobility analyzer (TSI model 3081) and condensation particle counter (CPC; TSI model 3025). CPC was operated in high flow mode with aerosol flow rate 800 cm3/min and particle density in Aerosol Instrument Manager software was set to default value 1.2 g/cm3. Samples for particle characterization were deposited onto transmission electron microscopy (TEM) grids using a nanometer aerosol sampler (NAS, TSI model 3089) and on cellulose, quartz, Zefluor, and Sterlitech Ag filters. Filters were weighed on scales Sartorius M5P-000V001 with readability 1 μg. Particle characteristics were studied by transmission electron microscopy (TEM, JEOL 2000FX, samples on TEM grids), scanning electron microscopy (SEM, TESCAN INDUSEM, Ag filters), selected area electron diffraction (SAED, TEM grids), energy dispersive spectroscopy (EDS, Bruker Quantax, Ag filters), inductively coupled plasma–optical emission spectrometry (ICP-MS ELEMENT 2, ThermoFisher Scientific, cellulose filters), elemental carbon (EC) and organic carbon (OC) analyzer (EC/OC, Model 4, Sunset Laboratory, quartz filters), X-ray diffraction (XRD, Bruker D8 Discover diffractometer, Ag filters), and X-ray photoelectron spectrometry (XPS, ADES-400, Ag filters, VG Scientific Limited, Sussex, UK). Some further details of the particle characterization are given in the online supplemental information (SI).
3. Results and discussions
3.1. Particle production
Four experimental campaigns, with duration of 33, 31, 99, and 101 h (264 h in total), were performed with one batch of precursor. The NPs production and characteristics were studied in terms of dependence on TTIP vapor pressure (PTTIP; 1.6–2.8 Pa, controlled both by saturator temperature, TS, and by saturator flow rate, QS), reactor temperature (TR; 500–900°C), reactor flow rate (QR; 800–1400 cm3/min), and oxygen concentration (cO; 0 or approximately 12 vol. %). An example of the dependence of particle production on time and various experimental conditions during the 99 h-long experiment (3rd experimental campaign) is shown in . Particle production is represented by number (Nt), surface (St), mass (Mt), total concentrations, geometric mean diameter (GMD), as well as number (N), surface (S), and mass (M) particle size distributions. In this figure, the data are plotted as they were collected by SMPS, including start-up period and technological events as replacement of nitrogen gas cylinder (scan 316, during which SMPS sampled an air from the laboratory). Analogical graphs of NPs production during experimental campaigns 1, 2, and 4 are plotted in Figures S1a–c (see the SI). Experiments have shown that particle size distribution can be controlled by TR, PTTIP, and QR. Typically, in the range of investigated experimental conditions, with increasing TR and QR the number concentration increases and the geometric mean diameter decreases, while the precursor vapor pressure has the opposite effect. With increasing precursor concentration, the size distribution shifts toward larger particle sizes (mass concentration increases), but number concentration decreases. As a result, surface and mass concentrations show maximum values at moderate TR from 600°C to 750°C and they can be increased by an increase of PTTIP, see Figure S1b in the SI. Stability of NPs production can be seen, e.g., in over a time period from 81 to 93 h. It is plotted in more detail in the form of number, surface, and mass particle size distributions in . Average values of Nt, St, and Mt concentrations during this period are 1.49 × 107 #/cm3, 2.79 × 1011 nm2/cm3, and 5.74 × 103 μg/m3, respectively. Stability of four other steady-state periods in the terms of total number and mass concentrations is plotted in Figure S2a, and in terms of median diameter and GSD in Figure S2b (see the SI).
Figure 2. The dependence of Nt, GMD, St, Mt, and number, mass and surface particle size distributions on time and experimental conditions (TR, QR, PTTIP, cO) during 3rd experimental campaign. Vertical gray bars highlight sampling periods, F denotes Ag filter, FC cellulose filter, and FQ quartz filter.
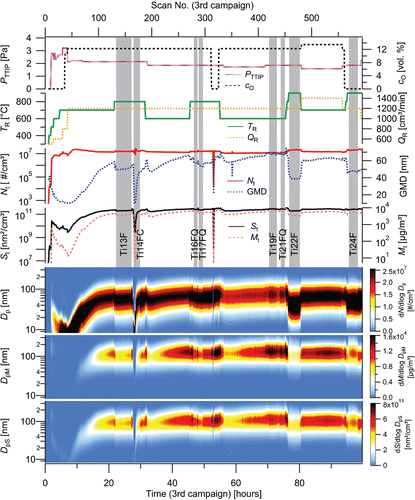
Figure 3. Stability of NPs production in the form of number, surface, and mass particle size distributions during 12 h long steady-state period, TR = 700°C, QR = 1400 cm3/min, PTTIP = 1.59 Pa, cO = 13.5 vol. %, 3rd experimental campaign.
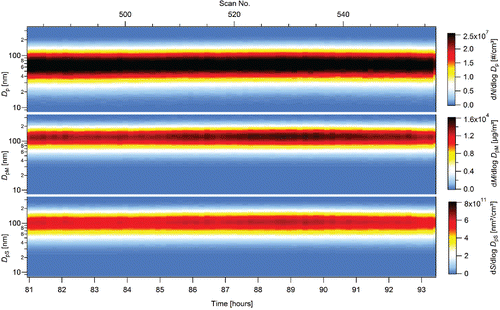
Besides variation of Nt and GMD with process parameters (TR, QR, PTTIP, and cO), one can also observe a start-up period at the beginning of particle generation process. When the reactor temperature reached 700°C (scan No. 43 in ), the particle size distribution was mostly below measuring range of SMPS (Nt = 8.02 × 106 #/cm3, GMD = 10 nm). As time went on, the GMD increased up to 56 nm at scan No. 123, which was when the particle production reached the steady state. On the other hand, Nt reached a maximum at scan No. 58 (2.35 × 107 #/cm3), and after which it gradually decreased until reaching a steady-state value 1.39 ×107 #/cm3. Due to increasing GMD, the total mass production of NPs gradually increased throughout the whole start-up period. It took approximately 13 h to achieve a steady state of particle production at given experimental conditions (TR = 700°C, QR = 1200 cm3/min, PTTIP = 2.14 Pa). Particle size distributions of scans No. 43, 58, 83, 113, and 123 are plotted in .
Figure 4. A development of the particle size distribution during start-up period, 3rd experimental campaign, TR = 700°C, QR = 1200 cm3/min, PTTIP = 2.14 Pa, cO = 12 vol. %.
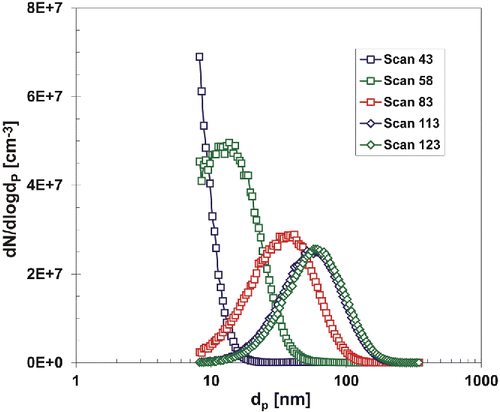
Start-up or induction period was already reported, e.g., by Kirkbir and Komiyama (Citation1987) or Komiyama et al. (Citation1984) and mentioned also by Koivisto et al. (Citation2011). This gradual increase of NP production was attributed to the catalytic effect of TiO2 deposit on the reactor wall (surface reaction). The difference is that the length of induction period in their experiments was shorter (120 min) than ours, and that in the reactor coated by TiO2 the induction period was as short as 10 min. We did not observe this shortening of the induction period in our experiments. It seems that TiO2 deposit was more or less deactivated during several day long breaks between our experimental campaigns. The shorter start-up period in the above-mentioned studies might be caused by different reactor material used: ceramic in our study and glass or quartz in theirs.
3.2. NPs characterization
3.2.1. Morphology
A SEM image of NPs synthesized at TR = 700°C by oxidation is shown in Figure S3 (see the SI). It is obvious that the sample consists of NPs without large submicron sized particles. SEM images of the other samples showed similar morphology and confirmed the homogeneity of the samples over large sample areas.
The results from TEM images are summarized in and TEM images of individual samples can be seen in Figures S4a–g (see the SI). The typical size of primary particles increases from 6–11 nm at TR = 500°C to 12–20 nm at TR = 900°C. However, the maximum NPs size, i.e., the largest NPs at given experimental conditions, had a minimum at TR = 800°C, . Although NPs synthesized at lower TR have smaller typical sizes, they are more polydisperse than those synthesized at higher TR, see . also shows that some particles synthesized at TR = 900°C were facetted. The lowest dispersity of the particle size was observed in NPs synthesized at TR = 800°C (). NPs synthesized by pyrolysis seem to be slightly larger than those formed by oxidation at the same TR, QR, and PTTIP (compare samples Ti15G vs. Ti18G and Ti23G vs. Ti25G in , , and Figures S4f and S4g (see the SI), but the differences are rather small.
Table 1. Morphological properties and SAED characteristics of NPs synthesized at various experimental conditions.
Figure 5. TEM images and electron diffraction patterns of the samples of NPs synthesized at 500 (Ti33G) and 900°C (Ti23G), respectively.
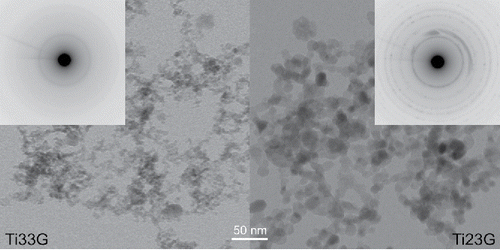
Figure 6. TEM images and electron diffraction patterns of the samples of NPs synthesized at 800°C by pyrolysis (Ti18G) and oxidation (Ti15G), respectively.
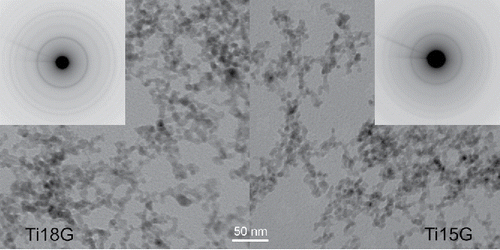
In contrast with our previous research in the glass reactor (Moravec et al. Citation2001), where particles with bimodal size distribution and some portion of submicron sized particles were produced, in the present study, the synthesized NPs had a monomodal size distribution with a low dispersity, which can be seen in , , and Figures S4a–g (see the SI). In the present study, higher TR and QR (lower residence time) were applied, but we can still make a direct comparison of morphology of particles produced at TR = 500°C. The difference is significant: no particles larger than 50 nm were produced in the IAP reactor and a bimodal size distribution with quite a few submicron sized particles (100–1000 nm) was generated in the glass reactor (Figure S5, see the SI).
Morphologies of particles prepared by other authors vary significantly, which is most probably due to different experimental conditions and arrangements of the apparatuses. Quite large and polydisperse particles were prepared by Kirkbir and Komiyama (Citation1987), who reported decreasing GMD from 300 to 65 nm with increasing TR from 300°C to 700°C, as well as Choi and Park (Citation2006), who reported the same trend with slightly lower values in the same range of TR. Both studies were performed in a quartz tube reactor, but in the work of Kirkbir and Komiyama (Citation1987) glass rings were inserted inside the quartz tube. Production of submicron sized and quite polydisperse particles in a glass reactor was reported also by Komiyama et al. (Citation1984). On the other hand, an increasing GMD from 19 to 37 nm with increasing TR from 400°C to 600°C was reported by Okuyama et al. (Citation1986), but material of the reactor was not specified. Formation of NPs, consisting of crystallites in a size range from 10 to 60 nm, in a stainless steel reactor at 500°C was reported by Koivisto et al. (Citation2011). Very small NPs, with mean sizes ranging between 6 and 16 nm in the temperature range from 500°C to 1200°C, were synthesized by Nakaso et al. (Citation2003) in a quartz reactor. The primary particles exhibited a minimum size of 6 nm at 700°C.
It seems that besides catalytic effect of the surface of TiO2 particles, the material of the reactor itself also played a role. Bradley (Citation1989) reported a mechanism of thermal decomposition of zirconium tert-butoxide (ZTBO) in an all-glass system at 200–250°C. Decomposition was initiated by water molecules adsorbed on the glass surface. Tertiary alcohol was formed as an intermediate product and dehydration of the alcohol was catalyzed by glass surface and led to the formation of olefin and water. As a result, two molecules of water are produced from one molecule of metal alkoxide. Therefore, it appears that glass surface accelerates the process of particle formation during the start-up period and that in the glass reactor the particles are produced by two reaction mechanism: NPs by homogenous reaction in the reactor volume and submicron sized particles by heterogenous reaction on the reactor wall. The release of water molecules by surface reactions, either on a glass surface or TiO2 deposit, accelerates gas phase decomposition (hydrolysis) of the precursor and the formation of new primary particles. From the comparison of our two studies (ceramic and glass reactors) and the literature, it seems obvious that these catalytic processes have different impacts on the process of particle formation. The length of the start-up period is quite short in a glass reactor and formation of widely spread from nano to submicron sized particles occurs (Komiyama et al. Citation1984; Kirkbir and Komiyama Citation1987; Moravec et al. Citation2001), while in a ceramic/stainless steel reactor the start-up period is much longer (this report) and formation of submicron sized particles is not observed, this report and, e.g., Koivisto et al. (Citation2011) and Okuyama et al. (Citation1986).
3.2.2. Crystallinity
In the samples generated at TR ≤ 800°C XRD analysis identified tetragonal anatase (PDF ICDD 86-1156) in addition to cubic silver (87-0717) and cubic chlorargyrite (AgCl: 31–1238) from the Sterlitech Ag filters. An example is shown in for particles generated at TR = 600°C. With increasing TR, peaks attributed to anatase become higher and narrower. At TR = 900°C, traces of rutile diffraction pattern (01–1292) were detected in addition to anatase (). Mean crystallite size, calculated from the Scherer equation using integral breadth, were 6.8 nm at TR = 600°C, and 12.8 nm at TR = 900°C. XRD pattern of the sample of NPs after annealing at temperature 800°C for 2 h is shown in Figure S6 (see the SI).
Figure 7. XRD pattern and SEM image (inset) of the sample Ti19F, TR = 600°C, QR = 1200 cm3/min, PTTIP = 1.9 Pa, cO = 12 vol %, A denotes anatase, C chlorargyrire, and S silver.
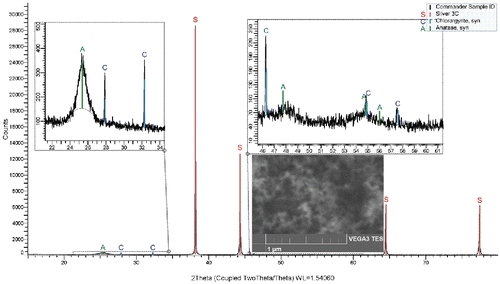
Figure 8. XRD pattern and SEM image (inset) of the sample Ti24F, TR = 900°C, QR = 1200 cm3/min, PTTIP = 1.9 Pa, cO = 0, A denotes anatase, C chlorargyrire, R rutile, and S silver.
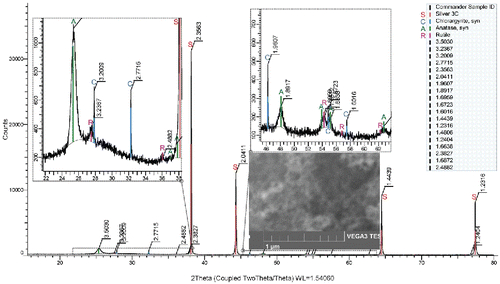
Results obtained by XRD method were confirmed by SAED. Number and intensity of rings in electron diffraction patterns increased with TR, which can be seen in , and . All rings belong to the anatase electron diffraction pattern. However, at TR = 900°C (), there were also some visible dots corresponding with rutile electron diffraction pattern. As in the case of morphology, we did not observe any substantial difference in the crystallinity of the samples synthesized by pyrolysis and oxidation. Results reported by other authors (Okuyama et al. Citation1986; Kirkbir and Komiyama Citation1987; Choi and Park Citation2006) were quite similar. Generally, at TR ≤ 400°C, NPs were amorphous, at TR ranging from 400°C to 800°C the NPs were anatase and above 800°C the NPs were anatase and/or rutile. Results reported by Choi and Park (Citation2006) differ from ours, as they recorded the formation of more crystalline NPs by oxidation than by thermal decomposition in an inert atmosphere.
3.2.3. Composition
EDS spectra confirmed presence of Ti and O in the samples deposited on Sterlitech Ag filters, as well as occurrence of some other elements. An illustration of EDS spectrum of the sample Ti9 can be seen in an inset of Figure S3 (see the SI). Concentration of Ti in the samples on the cellulose filters was analyzed by the ICP-MS method; the recalculated content of TiO2 varied between 74 and 85 weight %. EC/OC analyses of the samples deposited on quartz fiber filters showed that samples were free of EC and the content of OC was typically between 3 and 6 weight %, extreme values were 1.7% and 11%. XPS analyses were performed from the surface layers cca 3 and 6 nm using detection angles 60 and 0 degrees and confirmed presence of Ti in bonds Ti-O (TiO2) and C in bonds C-C, C-H, C-O, and C˭O. The content of C in the surface layer of particles is almost the same in the samples as prepared and in the samples that have undergone annealing. This indicates that surface of NPs is contaminated mostly by components from the atmosphere: CO2, volatile organic compounds, and also humidity.
3.3. Discussion
A comparison of TEM images with SMPS data indicates that SMPS detects agglomerates of NPs, because GMD is several times larger than the mean size of primary particles. This implies that SMPS results overestimate the volume and underestimate the surface concentrations of NPs. Estimates of mass concentrations depend on the selected NPs density. Even though the anatase bulk density is 3.78 g/cm3, in our experiments we set NPs density in the Aerosol Instrument Manager software to 1.2 g/cm3, due to expected porosity of NPs and NPs agglomerates. We can calculate mass concentrations from the filter measurements, but only approximately and moreover only for some experimental conditions. We know the precise values of mass of the samples, but we can only estimate the flow rate through the filter during sampling period. Typically, the flow rate of the aerosol at the diluter output was 3000 cm3/min and aerosol flow rate to SMPS 800 cm3/min, therefore initial sampling flow rate was set as close as possible to 2200 cm3/min. Due to increasing pressure drop and decreasing sampling flow rate during sampling period, we estimated the average sampling flow rate to 2000 cm3/min. From Figure S7 (see the SI), we can see that the ratio of filter mass concentration to SMPS mass concentration (MFilters/MSMPS) increases from 40% at TR = 500°C to 100% at TR = 900°C. The downward deviations of MFilters/MSMPS from this trend at TR = 800°C can be obviously attributed to the smallest NPs size and probably more agglomerated particles at this TR.
Typical mass concentrations detected by SMPS for NPs density 1.2 g/cm3 ranged from 3000 to 5700 μg/m3, maximum value 9500 μg/m3 was achieved for TR = 750°C, QR = 1200 cm3/min, PTTIP = 2.47 Pa, cO = 12.25 vol. %. At a flow rate 3000 cm3/min these values represent emission rates 9–17 μg/min, maximum value was 29 μg/min. Mass concentrations from filter measurements were lower, typically 2000–3000 μg/m3, extreme values were 5170 and 7400 μg/m3. These values correspond with emission rates 6–9, 16, and 22 μg/min, respectively.
Exposure experiments are being performed in the Institute of Analytical Chemistry of the CAS using almost identical NPs generator and exposure chamber described by Večeřa et al. (Citation2011). A stream of generated aerosol (3 LPM) is further diluted by a stream of air (20 LPM) and introduced to exposure chamber with four boxes, each for 10 laboratory mice (T is maintained at 20–21°C, RH at 50%–60%). After this dilution, Mt drops from 3000 to 450 (typical) and from 7400 to 1100 μg/m3 (maximum). These values are above the limit of NIOSH 0.3 mg/m3 for nano-TiO2 and for up to 10 h per day during a 40-h work week. For surface area concentration, which is the biologically most effective dose metric for acute nanoparticle toxicity in lung (Schmid and Stoeger Citation2016), we have (underestimated) values from SMPS measurements. The average value 2.79 × 1011 nm2/cm3 from steady-state period in drops after dilution in an exposure chamber to the value 4.2 × 1010 nm2/cm3.
4. Conclusions
A method of TiO2 NPs generation by pyrolysis and oxidation of TTIP in an externally heated tube reactor is suitable for long-lasting inhalation experiments with laboratory animals. Using purified nitrogen as a carrier gas, NPs production with Nt above 1.0 × 107 #/cm3 and with primary particle size less than 50 nm was performed for 264 h in four experimental campaigns without noticeable decrease of NPs production. Production of NPs and their characteristics can be controlled by TR, QR, and PTTIP, while the influence of reaction atmosphere was practically negligible. Mass concentration of NPs can be kept within the range from 3000 to 9500 μg/m3, which corresponds with emission rates 9–29 μg/min, and surface area concentration from 1.8 × 1011 to 4.0 × 1011 nm2/cm3.
Synthesized NPs contain approximately 80 weight % of TiO2, they are free of EC and typically contain between 3 and 6 weight % of OC. NPs are partially crystalline and a portion of tetragonal anatase and mean crystallite size increases with TR.
Start-up period of NPs generation in an IAP reactor is several times longer than that observed in our previous study with glass reactor or as it was reported in the literature for experiments with glass reactor and deserves further investigation, but it was not the topic of this project, as its impact on long-lasting exposure experiments was not essential.
The most suitable parameters of NPs synthesis for long-lasting exposure experiments seem to be as follows: TR = 700°C–750°C, QR = 1200–1400 cm3/min, PTTIP ≥ 1.85 Pa (TS ≥ 40°C, Qs = 80 cm3/min), cO∼12 vol. %. At these parameters, number, surface, and mass concentrations are sufficiently high, primary particle size is small and consumption of nitrogen from gas cylinders is reduced by replacing part of it with compressed air. When necessary, the NPs production can be increased by an increase of TS. The results of this study provide a good basis for follow-up inhalation experiments.
UAST_1224803_SupplementalFile.zip
Download Zip (3.9 MB)Acknowledgments
TEM analyses were performed by Dr. Bohumil Smola, Faculty of Mathematics and Physics, Charles University, XPS analyses by Dr. Josef Zemek, Institute of Physics of the CAS, v.v.i., ICP-MS analyses by Dr. Jan Rohovec, Geological Institute of the CAS, v.v.i., and XRD analyses by Mgr. Anna Kallistová, Geological Institute of the CAS, v.v.i.
Funding
This work was supported by the Czech Science Foundation under Grant P503/12/G147.
References
- Ahonen, P. P., Tapper, U., Kauppinen, E. I., Joubert, J.-C., and Deschanvres, J.-L. (2001). Aerosol Synthesis of Ti-O Powders via in-Droplet Hydrolysis of Titanium Alkoxide. Mater. Sci. Eng. A, 315:113–121.
- Bankmann, M., Brand, R., Engler, B. H., and Ohmer, J. (1992). Forming of High Surface Area TiO2 Catalyst Supports. Catal. Today, 14:225–242.
- Bradley, D. C. (1989). Metal Alkoxides as Precursors for Electronic and Ceramic Materials. Chem. Rev., 89:1317–1322.
- Choi, J. G., and Park, K. J. (2006). Effect of Reaction Atmosphere on Particle Morphology of TiO2 Produced by Thermal Decomposition of Titanium Tetraisopropoxide. J. Nanoparticle Res., 8:269–278.
- Ferroni, M., Guidi, V., Martinelli, G., Faglia, G., Nelli, P., and Sberveglieri, G. (1996). Characterization of a Nanosized TiO2 Gas Sensor. NanoStruct. Mater., 7:709–718.
- Grätzel, M. (2003). Dye-Sensitized Solar Cells. J. Photochem. Photobiol. C: Photochem. Rev., 4:145–153.
- Gu, Y., and Oyama, S. T. (2009). Permeation Properties and Hydrothermal Stability of Silica-Titania Membranes Supported on Porous Alumina Substrates. J. Membr. Sci., 345:267–275.
- Jang, H. D. (1997). Effects of H2O on the Particle Size in the Vapor-Phase Synthesis of TiO2. AIChE J., 43:2704–2709.
- Kim, S. Y., Yu, J. H., and Lee, J. S. (1999). The Characteristics of Nanosized TiO2 Powders Synthesized by Chemical Vapor Condensation. NanoStruct. Mater., 12:471–474.
- Kirkbir, F., and Komiyama, H. (1987). Formation and Growth Mechanism of Porous, Amorphous, and Fine Particles Prepared by Chemical Vapor Deposition. Titania from Titanium Tetraisopropoxide. Can. J. Chem. Eng., 65:759–766.
- Kirkbir, F., and Komiyama, H. (1988). Low Temperature Synthesis of TiO2 by Vapor-Phase Hydrolysis of Titanium Tetraisopropoxide. Chem. Lett., 17:791–794.
- Koivisto, A. J., Lyyränen, J., Auvinen, A., Vanhala, E., Hämeri, K., Tuomi, T., and Jokiniemi, J. (2012). Industrial Worker Exposure to Airborne Particles during the Packing of Pigment and Nanoscale Titanium Dioxide. Inhal. Toxicol., 24:839–849.
- Koivisto, A. J., Mäkinen, M., Rossi, E. M., Lindberg, H. K., Miettinen, M., Falck, G. C.-M., Norppa, H., Alenius, H., Korpi, A., Riikonen, J., Vanhala, E., Vippola, M., Pasanen, P., Lehto, V.-P., Savolainen, K., Jokiniemi, J., and Hämeri, K. (2011). Aerosol Characterization and Lung Deposition of Synthesized TiO2 Nanoparticles for Murine Inhalation Studies. J. Nanopart. Res., 13:2949–2961.
- Komiyama, H., Kanai, T., and Inoue, H. (1984). Preparation of Porous, Amorphous, and Ultrafine TiO2 Particles by Chemical Vapor Deposition. Chem. Lett., 13:1283–1286.
- Mäkelä, J. M., Aromaa, M., Teisala, H., Tuominen, M., Stepinen, M., Saarinen, J. J., Toivakka, M., and Kuusipalo, J. (2011). Nanoparticle Deposition from Liquid Flame Spray onto Moving Roll-to-Roll Paperboard Material. Aerosol Sci. Technol., 45:827–837.
- Moravec, P., Smolík, J., and Levdansky, V. V. (2001). Preparation of TiO2 Fine Particles by Thermal Decomposition of Titanium Tetraisopropoxide Vapor. J. Mater. Sci. Lett., 20:2033–2037.
- Moravec, P., Smolik, J., Ondráček, J., Vodička, P., and Fajgar, R. (2015). Lead and/or Lead Oxide Nanoparticle Generation for Inhalation Experiments. Aerosol Sci. Technol., 49:655–665.
- Nakaso, K., Okuyama, K., Shimada, M., and Pratsinis, S. E. (2003). Effect of Reaction Temperature on CVD-Made TiO2 Primary Particle Diameter. Chem. Eng. Sci., 58:3327–3335.
- Okuyama, K., Kousaka, K., Tohge, K., Yamamoto, S., Wu, J. J., Flagan, R. C., and Seinfeld, J. H. (1986). Production of Metal Oxide Aerosol Particles by Thermal Decomposition of Metal Alkoxide Vapors. AIChE J., 32:2010–2019.
- Ollis, D. F., Pelizzetti, E., and Serpone, N. (1991). Photocatalysed Destruction of Water Contaminants. Environ. Sci. Technol., 25:1522–1529.
- Pratsinis, S. E., Zhu, W., and Vemury, S. (1996). The Role of Gas Mixing in Flame Synthesis of Titania Powders. Powder Technol., 86:87–93.
- Rekoske, J. E., and Barteau, M. A. (1997). Isothermal Reduction Kinetics of Titanium Dioxide-Based Materials. J. Chem. Phys. B, 101:1113–1124.
- Robichaud, C. O., Uyar, A. E., Darby, M. R., Zucker, L. G., and Wiesner, M. R. (2009). Estimates of Upper Bounds and Trends in Nano-TiO2 Production as a Basis for Exposure Assessment. Environ. Sci. Technol., 43:4227–4233.
- Schmid, O., and Stoeger, T. (2016). Surface Area is the Biologically Most Effective Dose Metric for Acute Nanoparticle Toxicity in the Lung. J. Aerosol Sci., 99:133–143. http://dx.doi.org/10.1016/j.jaerosci.2015.12.006i
- Večeřa, Z., Mikuška, P., Moravec, P., and Smolík, J. (2011). Unique Exposure System for the Whole Body Inhalation Experiments with Small Animals, in 3rd International Conference NANOCON 2011, September 21–23, Brno, Czech Republic, pp. 652–654.
- Večeřa, Z., Mikuška, P., Moravec, P., and Smolík, J. (2012). The First Experience with New Exposure System for the Whole Body Inhalation Experiments with Small Animals, in 4th International Conference NANOCON 2012, October 23–25, Brno, Czech Republic, pp. 697–699.
- Wang, W.-N., Lenggoro, I. W., Terashi, Y., Kim, T. O., and Okuyama, K. (2005). One-Step Synthesis of Titanium Oxide Nanoparticles by Spray Pyrolysis of Organic Precursors. Mater. Sci. Eng. B, 123:194–202.