ABSTRACT
Detection and quantification of dilute viral aerosols, as encountered outside animal housing facilities, requires methods that are able to detect small numbers of viruses in large volumes of air. This study compared the performance of two size-differentiating cascade impactors; an Andersen 8-stage (ACI; 28.3 L/min) and a high volume Tisch (TCI; 1,133 L/min) to assess sampling efficiency for detecting porcine reproductive and respiratory syndrome virus (PRRSV) and influenza A virus (IAV). Samples of particles sorted by aerodynamic diameter were analyzed by quantitative polymerase chain reaction (qPCR) and collection efficiency was assessed by particle size. Collection media (minimum essential medium [MEM] and beef extract [BE]), elution technique (active versus passive), and sampling times (10, 20, and 30 min) were variables assessed for the TCI sampler. Extraction efficiency was 35% higher with BE as compared to that of MEM (p = 0.0007); active extraction technique was 19% more efficient than the passive technique (p = 0.03); time of sampling did not significantly affect the amount of virus recovered. The ACI sampler was more efficient in detecting both viruses from small and medium sized airborne particles (≤3 μm) as compared to the TCI sampler (p < 0.001). The latter sampler, however, was more efficient at IAV detection from large airborne particles (>3 μm) (p = 0.0025) indicating the potential of this sampler in detecting the presence of small amounts of viruses in aerosols under field conditions.
© 2017 American Association for Aerosol Research
EDITOR:
Introduction
Viruses associated with airborne particles have the potential to be transmitted from infected livestock premises to naïve populations and propagate epidemics that cause substantial economic losses and impact the food supply (Stark Citation1999). Infectious diseases affecting swine are of particular importance since pork is the most consumed protein worldwide (FAO Citation2016). Foot and mouth disease virus (FMDV), porcine reproductive and respiratory syndrome virus (PRRSV), and influenza A virus (IAV) are examples of airborne pathogens that cause devastating losses due to their pathogenicity, ability to travel long distances and, for IAV, zoonotic risk (Gloster et al. Citation2010; Otake et al. Citation2010; Corzo et al. Citation2013).
To determine the relative contribution of the aerosol route to the overall transmission of viruses among swine herds, sensitive and precise methods for quantifying virus in air samples are needed. PRRSV, IAV, and H5N2 highly pathogenic avian influenza genetic material was found associated with particle sizes ranging between 0.3 and 10 µm in aerosols emitted by experimentally infected pigs (Alonso et al. Citation2015) and poultry under field conditions (Torremorell et al. Citation2016). Knowledge of the concentration of pathogens associated with airborne particles of different aerodynamic diameters at different distances from a point source should help predict the risk of airborne transmission events in the vicinity of infected herds. This is complicated by the complex dynamics of dilution of contaminated air plumes that exit the infected facilities (Cooper et al. Citation2014) and the ability of sampling methods to determine pathogen concentrations. High-volume size differentiating air samplers have been commonly used because they are able to sample large volumes of air and, in conjunction with quantitative polymerase chain reaction (PCR) techniques, detect low amounts of infectious agents relatively rapidly, which should aid in the face of an emergency response (Cooper et al. Citation2014). However, the sensitivity of high-volume air sampling equipment for detecting low virus concentrations has not been rigorously evaluated. In contrast, commercially available low-volume air samplers (i.e., liquid impingers, swirling aerosol collectors, and cascade impactors) have been commonly used to collect viral aerosols (Verreault et al. Citation2008) and they can be used as a reference to evaluate the efficiency and sensitivity of high-volume air samplers.
In this study, we first compared the recovery efficiency of PRRSV and IAV using different eluents, extraction techniques, and three types of collection substrates. We then investigated the recovery efficiency of different concentrations of nebulized viruses. Finally, and based on previous results, we compared the performance of a high-volume five-stage cascade impactor air sampler (TCI) versus a lower-volume but well-characterized eight-stage cascade impactor (ACI) (Appert et al. Citation2012; Zuo et al. Citation2013b; Ge et al. Citation2014) for detecting the two viruses.
Materials and methods
Virus propagation and titration
IAV/Swine/Iowa/00239/2004 H1N1 and PRRS MN 1-8-4 strains were propagated and titrated in Madin-Darby canine kidney (MDCK) and in a subline of the African monkey kidney cell line (MARC-145 cells), respectively (Mengeling et al. Citation1996; Brookes et al. Citation2010). The titers of stock virus suspensions were 1.13 × 105 TCID50/mL and 4.4 × 106 TCID50/mL for PRRSV and IAV, respectively. The virus stocks were aliquoted in small amounts and stored at −80°C until used. Both viruses, provided by the University of Minnesota Veterinary Diagnostic Laboratory, are known to be shed in aerosols by infected pigs (Cho et al. Citation2007; Corzo et al. Citation2014).
Virus elution from substrates
To determine virus elution efficiency from different filters/substrates, 300 µL of a virus suspension was applied as a single spot onto a piece of three types of glass fiber substrates from the two air samplers: (1) TCI substrate (slotted 15.2 cm × 17.8 cm, Model P/N TE-230-GF, New Star Environmental, Roswell, GA, USA); (2) final TCI substrate (Model G653, 20.3 cm × 25.4 cm, Whatman, GE Healthcare, UK); and (3) final ACI substrate (Model 934-AH, 80 mm, Whatman, GE Healthcare). Two elution techniques (active and passive) were evaluated along with two types of eluents (minimum essential medium [MEM] and 3% beef extract [BE] in 0.05 M glycine at pH 9.5). A total of 36 filters were evaluated (3 filter types × 2 elution techniques × 2 elution media × 3 replicates). The BE solution at alkaline pH was selected based on its ability to reduce virus absorption to surfaces as demonstrated in a previous study (Zuo et al. Citation2013a). MEM was also selected because of its ability to preserve swine viruses, specifically IAV, on surfaces and other materials (Tiwari et al. Citation2006; Thomas et al. Citation2008).
Prior to elution and after the application of virus suspension, the filters were placed in a biosafety cabinet to air dry (90 ± 15 min). For the passive technique, the filter was placed in a Petri dish followed by the addition of 20 mL of eluent solution. After 3 min, the filter was moved 20 times within the Petri dish using sterile forceps. The recovered eluate was frozen at −80°C until tested with reverse transcription-polymerase chain reaction (RT-PCR). For the active technique, the filter was cut in small pieces (each 1 cm2 square) using sterile scissors and forceps. The pieces were placed in a 50 mL plastic centrifuge tube containing 20 mL of an eluent solution followed by inversion of the tube 10 times to mix the contents. The mixing step was repeated again after 5 min. The tube was then vortexed followed by centrifugation at 2000 × g for 5 min. The eluate obtained with BE solution was adjusted to neutral pH. One ml of viral solution was kept as a positive control while MEM solution applied to the three types of filters was used as negative control.
Virus recovery efficiency
Based on the results of the above experiment, a sensitivity study was performed to determine the minimum amount of virus detectable by the active virus elution technique using alkaline solution of BE as an eluent. Three slotted glass fiber filter substrates were spiked with 300 µL on a single spot of three different concentrations of PRRSV and IAV (starting concentrations of 1.2 × 109 and 4 × 107 RNA copies/mL, respectively, see total virus spiked in ) and placed in a biosafety cabinet to air dry (90 ± 15 min). A total of 18 filters were analyzed (3 concentrations × 2 virus × 3 replicates).
Table 1. Mean RNA copies ± SD of porcine reproductive and respiratory syndrome virus (PRRSV) and influenza A virus (IAV) spiked and eluted from the filters as well as recovery efficiency (%) of the viruses as a function of virus concentration after the active technique was applied. Values are the mean of three replicates.
Comparison of air samplers
This experiment was carried out in an isolation room at the University of Minnesota BSL-2 animal research facility. The room had a total air space of 35.1 m3, was mechanically ventilated, and had a negative pressure of 0.11 inches of water. The setup of the air samplers and distribution of the aerosol was described previously (Alonso et al. Citation2016). Both incoming and outgoing air were filtered. Relative humidity and temperature were monitored continuously using a data logger (ThermaData Logger-Model HTD, ThermoWorks, Lindon, UT, USA). The virus suspensions were spiked with a fluorescent tracer dye (fluorescein sodium salt, Fluka, Buchs, Switzerland) and then aerosolized continuously at 20 psi using a 6-jet Collison-nebulizer (BGI Inc., Waltham, MA, USA). A series of air samples were obtained in order to compare the two air samplers: the ACI, an Andersen eight-stage nonviable cascade impactor with 80 mm aluminum impaction plates at a flow rate of 28.3 L/min (Thermo Scientific, Franklin, MA, USA), and the TCI, a high-volume Tisch cascade impactor (Model 230, Tisch Environmental, OH, USA) with slotted aluminum plates (15.2 cm × 17.8 cm) that were lined with four slotted collection substrates (glass fiber filters) at a flow rate of 1130 L/min. Both air samplers, equipped with final filters, were compared over different sampling times (10, 20, and 30 min). Each experiment was done in triplicate. For each sampling event, samples were collected sequentially first using the ACI and then the TCI. There was a 15 min interval between each sampling event to allow the aerosol to reach a steady-state. The top of both samplers was located at 70 cm from the floor. Sample collection started after 15 min of aerosol generation, which was the time needed to reach a steady-state.
The ACI sampler separates airborne particles into 8 size stages with aerodynamic diameter cut-points of 0.4, 0.7, 1.1, 2.1, 3.3, 4.7, 5.6, and 9.0 µm. Particles smaller than 0.4 µm are captured by a final glass fiber substrate filter. After each sampling period, the ACI was removed from the room, and virus from the eight plates was eluted by scraping the plate with a rubber spatula in 1.5 mL of MEM (Appert et al. Citation2012). All samples were transferred into 1.5 mL sterile plastic tubes, placed on ice and stored at −80°C until tested. Between replicates, all plates and stages were scrubbed and disinfected with alkyl dimethyl benzyl ammonium chloride soap (Lysol, Reckitt Benckiser) and finally rinsed and dried with paper towels. After disinfection and drying, a minimum of four collection plates and individual ACI stages were swabbed to serve as negative controls, and samples stored at −80°C.
The TCI sampler separates particles into four stages with aerodynamic particle diameter cut-points of 0.95, 1.5, 3.0, and 7.2 μm. Particles from these four stages are captured by slotted glass fiber collection substrates and particles smaller than 0.95 µm are captured by a final filter. After each sampling period, the sampler was taken to the laboratory where the collection substrates and filters were removed using sterile gloves. The filters were folded and placed into individually labeled Petri dishes and refrigerated at 4°C until processed for virus elution. Between replicates, all stages were disinfected with 70% alcohol, air dried, and finally rinsed and dried with paper towels. After disinfection and drying, all stages were swabbed, and samples stored at −80°C. For each replicate, there were eight stages assayed for the ACI, four stages for the TCI, and two backup filter samples.
Total airborne particles by particle size were counted using an optical particle counter (OPC) (AeroTrak 9306 Handheld Particle Sizer, TSI, St. Paul, MN, USA) for 10, 20, and 30 min during each sampling event of each of the air samplers.
Quantitative real-time RT-PCR
All air samples from the ACI, the TCI substrates, and the eluted samples originating from the spiked assessments were tested using a quantitative IAV and PRRSV reverse transcription polymerase chain reaction (RT-PCR) as previously described (Cho et al. Citation2006; Corzo et al. Citation2013). Based on transcript RNA, a standard curve with a quantitative linear range from 1 × 101 copies/µL to 1 × 1010 copies/µL was used in duplicate to determine the RNA copy number/mL. The copy number/mL results were consecutively converted to copies/m3 of air.
Fluorometric quantification
For the purpose of quantifying the amount of total particles and to understand the physical collection efficiency of both air samplers in the initial (before aerosolization) and final suspensions (after air sampling), a fluorescent dye was added to the nebulizer suspension as previously described (Agranovski et al. Citation2005; Appert et al. Citation2012; Ge et al. Citation2014). Fluorescein levels in the nebulizer suspension (before and after sampling), as well as those from substrates and impactor stage eluates were assessed using a spectrofluorometer (Model Synergy H1, BioTek, Winoosk, VT, USA). All samples were transferred in triplicate into 96-well black plates (Thermo Fisher Scientific, Roskilde, Denmark) and fluorescein intensity was measured at λ = 515 nm and at λ = 485 nm after the transition of the eluent to a high-energy electronic state or excitation. Results were expressed as fluorescein intensity per mL or volume of air (m3) depending on the type of sample. To quantify the fluorescein in the eluted samples, linear regression trend lines were used to calculate the calibration curves for the two types of media; slope (m) and intercept (c) from the linear regression equation were obtained (Microsoft EXCEL; Microsoft Corporation, Redmond, WA, USA). Unknown concentrations of fluorescein (y) were then calculated substituting the fluorescein intensity reading (x) in the equation:[1]
Data and statistical analysis
Data from the qRT-PCR results, replicate number, virus, type of air collector, time of sampling, and fluorescein tracer concentrations were entered into a spreadsheet (Microsoft EXCEL; Microsoft Corporation, Redmond, WA, USA) and organized for analysis. Means, standard deviations, and minimum and maximum values for quantitative variables, and frequency counts and percentages for qualitative variables were calculated for descriptive analysis. Negative PCR results in the stages of both air samplers were included in the analysis when one or more stages of the sampler had a Ct value within the positive or suspect range (Ct value <40). Negative results included in the analysis were assigned a value of 100 RNA copies/mL according to the limit of detection of the qRT-PCR technique.
To assess the recovery efficiency (Re) from the different filter substrates the quantity of virus present was calculated as[2] in which N eluate is the virus titer from the solution eluted from the filter and N loaded is the virus titer in the stock solution. To assess the distribution of particles aerosolized during the study, the number of total particles (n) counted by the OPC on a specific interval (x) was transformed to a volume concentration (v):
[3] in which d is the geometric midpoint diameter of x.
In regards to the efficiency comparison between both air samplers for different particle size ranges, a new variable called “size” was created. If the geometric mean point of a specific interval (square root of both ends of the interval product) was <1 µm, ≥1 and ≤ 3 µm, or > 3 µm then “size” was equal to “small,” “medium,” or “large,” respectively. Due to the number of variables in the study, a multivariate modeling analysis was performed. The variable “replicate” was considered as a random variable. Under these premises, the total quantity of virus (RNA copies/m3) adjusted by type of air sampler, size of particles, virus and time of sampling was assessed for significance using a mixed linear regression model in SAS 9.1 (SAS Institute, Cary, NC, USA). p-Values of the multiple comparisons were adjusted by Tukey–Kramer's test and considered significant at p < 0.05.
Results
Spiked test recovery efficiency results by extraction technique, type of substrates, and elution media
Results from the qPCR analysis demonstrated that “type of eluent media,” “type of substrates,” and “extraction technique” were significant variables in the model. Briefly, the BE eluent yielded a 35% higher extraction efficiency compared to MEM (p = 0.0007); the active extraction technique was 19% more efficient than the passive technique (p = 0.03); and, filter type ACI final filter yielded the highest extraction efficiency (73%), followed by the TCI filter (25%), and ultimately the TCI-backup filter (26%). No difference was observed between the two TCI types of filters (Table S1 in the online supplementary information).
Sensitivity of recovery efficiency by virus type
Results from the sensitivity analysis are shown in . The recovery efficiency using the active technique and BE as elution media decreased with the decrease in virus concentration. Virus recovery efficiency ranged between 0% and 22% and 12% and 22% for PRRSV and IAV, respectively, from the least to the most concentrated samples.
Comparison of air samplers
Comparison by viral load
Raw data of all sample points collected by the two air samplers during different sampling periods are represented in for both IAV () and PRRSV (). For the comparisons between air samplers based on the multivariable analysis, the variable “time” was removed from the final model due to lack of significance. For both IAV and PRRSV, the ACI sampler was more efficient than the TCI sampler (p < 0.001) at recovering virus from small and medium size particles when virus concentration in the air was high (). In contrast, the TCI sampler appeared to be more efficient at recovering virus from larger particles, although the difference was statistically significant only for IAV (p = 0.0025) but not PRRSV (p = 0.258).
Figure 1. Raw data of particle size distribution (concentration of log 10 RNA copies/m3 of air) of influenza A virus (IAV) and porcine reproductive and respiratory syndrome virus (PRRSV) detected at different sampling periods by the Andersen cascade impactor (ACI) and the high volume Tisch cascade impactor (TCI) from mechanically generated aerosols. Lowest particle size geometric values represent the data from the back up filters. The results of the black line correspond to the ACI and the gray line to the TCI.
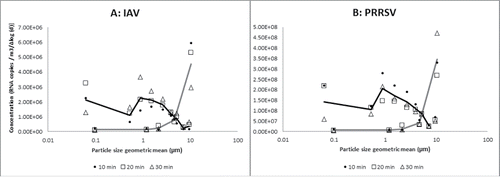
Figure 2. Particle size distribution (least-square means of log10 RNA copies/m3 of air and standard errors) of influenza A virus (IAV) and porcine reproductive and respiratory syndrome virus (PRRSV) detected by the Andersen cascade impactor (ACI) and the high volume Tisch cascade impactor (TCI) from mechanically generated aerosols. Results represent the mean values of three replicates.
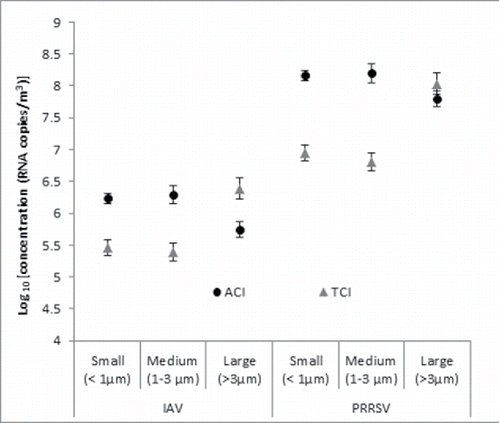
Comparison using fluorescein tracer
Results of fluorescein intensity for the different filters were expressed as µg/mL of air sample and then transformed to volume of air (m3) using the following calibration curves: y = 0.0072x + 0.0263 (R2 = 0.9623) and y = 0.0039x + 0.0188 (R2 = 0.9699) for DMEM and BE, respectively. The variable “time” was again removed from the final analysis due to lack of significance in the model. Overall, the ACI sampler was more efficient (p < 0.001) at recovering fluorescein associated with small and medium airborne particles at higher concentrations of fluorescein compared with the TCI sampler (). However, no difference between samplers was seen for recovery of fluorescein from larger airborne particles (p = 0.62).
Figure 3. Particle size distribution (least-square means of log10 RNA copies/m3 of air and standard errors) of fluorescein particles collected by the Andersen cascade impactor (ACI) and the high volume Tisch cascade impactor (TCI) from aerosols mechanically generated. Results represent the mean values of three replicates.
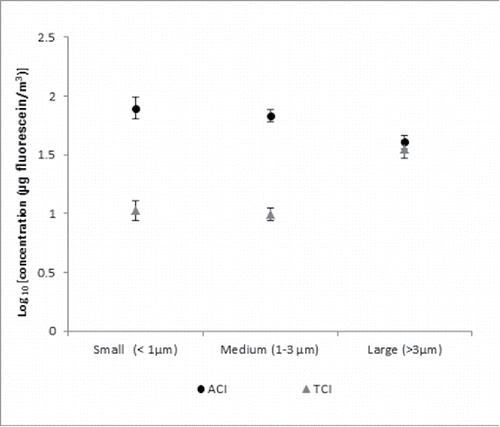
Distribution of airborne particles
An optical particle counter was used to measure the size distribution of total particles in the air at the same time when the ACI and TCI samplers were collecting air samples. Overall there were more total particles within the smallest sizes (0.5–3 µm) than the largest particles (>3 µm) (p < 0.05) during all air sampling events. The volume distribution of these particles is represented in for the ACI and the TCI, respectively. There were no statistically significant differences in the volume distribution of particles among different sampling times (10, 20, 30 min) when ACI and TCI samplers were operated throughout the study.
Figure 4. Volume distribution of total airborne particles (geometric mean diameter of volume of particles/m3) measured using an optical particle counter during sampling periods of 10, 20, or 30 min using the Andersen cascade impactor (ACI) (a) and the high volume Tisch cascade impactor (TCI) samplers (b). Results represent the mean values of three replicates.
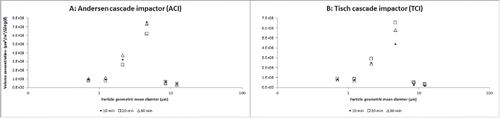
Discussion
Bioaerosol research is constrained by the limits of measurement tools to obtain accurate, reliable, and comparable exposure estimates for airborne microorganisms. Error in estimates of bioaerosols may originate at any step of the air sampling, collection, and processing protocol. Our specific interest was to advance understanding of methodologic factors that may influence observations of virus concentration in air, with a view to the particular challenge of sampling aerosols from outside animal housing facilities. Our data indicate that the higher airflow and collection principles of the high-volume TCI sampler overall did not improve the efficiency of detection of airborne viruses compared to the conventional ACI sampler. However, the TCI sampler was superior in the recovery of viruses associated with particles >3 μm (in the case of IAV) demonstrating a potential for its use in the field to predict the presence of viruses associated with larger particles.
The primary limitation to the use of filters for viral air sampling is the extraction of the sample from the filter. Based on a spike test, we compared the recovery efficiency of MEM and BE using two different extraction techniques. Difference in sampling efficiency between both collectors could be due to lower recovery efficiency after the aerosol test when compared to the spike test as demonstrated by other authors (Zuo et al. Citation2013a). The increase in recovery efficiency after increasing the concentration of virus solution for challenge of the substrates has been also demonstrated previously in a filter-based study comparing two large volume air samplers (EPA Citation2009). In our study, we used high concentrations of virus to compare the air sampler's efficiency, which not necessarily reflect rare events happening in the field due to lower concentrations of virus. Under those circumstances, the high flow rate air sampler may be more suitable than lower flow rate samplers. Further research on additional substrates and by sampling low concentration aerosols is indicated to improve virus recovery efficiency and detection capabilities under field conditions using large volume air samplers.
The methodology described in our study only allowed us to calculate recovery efficiency of virus based on physical penetration of RNA particles. Future studies should incorporate cell culture methods to measure live virus recovery (infectivity penetration) associated with different size ranges, considering the fact that both air samplers use different methods for collecting particles that may affect the infectivity of the virus. Infectivity penetration was calculated in a filtering face piece study to be of a magnitude of 2 orders lower than the physical penetration (Zuo et al. Citation2013a). Glass fiber substrate and high air flow could be responsible for structural damage and desiccation of the virus particles (Verreault et al. Citation2008), as well as the release of PCR interference particles during the TCI air sampling process (Blachere et al. Citation2007), which may have also impacted our results.
The impaction of particles as well as the bouncing of those particles (Dzubay et al. Citation1976) on aluminum plates as part of the ACI sampling process (Appert et al. Citation2012) could also be responsible for the differences in the recovery efficiency and distribution of virus particles between stages obtained between air samplers (especially for larger particles improving the results of smaller particles in the lower stages). Bouncing of particles from higher stages to lower stages is particularly significant for dry particles, although this process has also been described on filter substrates but not as strongly as from flat surfaces (Rao and Whitby Citation1978). In our study we did not assess the water content of our particles although we speculate that it is unlikely that the airborne particles became completely dry because of vapor pressure depression caused by dissolved materials in the fluids as shown by Nicas et al (Citation2005).
Fluorescein tracers have been previously applied to calculate physical and infectivity losses of viruses during the nebulization process in terms of fluorescein intensity as a reflection of the concentration of fluorescein in the virus solution (Appert et al. Citation2012; Ge et al. Citation2014). Since previous studies demonstrated no significant changes in the virus titer after nebulization and virus viability was not a part of the design of this study, the use of the fluorescein tracer was preferred as a confirmatory test to our molecular analysis based on concentration of viral RNA copies.
Results from this study demonstrate the difficulty of estimating the virus concentration in bioaerosols, specifically those from dilute aerosols outside of animal housing facilities, due to air sampling, collection, and processing challenges. Further research is needed to overcome these challenges in order to use these particle size differentiating air samplers under field conditions to better understand between-farm airborne disease transmission risks of important diseases of food animals and more specifically for the swine industry.
Conclusions
Preventing transmission of airborne pathogens is a priority for animal industries and for public health. Detection and quantification of dilute viral aerosols, as encountered outside animal housing facilities, requires methods that are able to detect small numbers of viruses in large volumes of air. We evaluated the recovery efficiency of a large volume particle size differentiating air sampler for detecting two important viruses affecting pigs, porcine reproductive and respiratory syndrome virus, and influenza A virus. We provide information on testing methods to conduct investigations under different settings, including field conditions. Under the conditions of this study the ACI sampler was more efficient than the TCI sampler at recovering virus smaller than 3 µm however the TCI sampler was more efficient at recovering virus from particles larger than 3 µm, being this difference significant only for one type of virus tested.
UAST_1249278_Supplementary_File.zip
Download Zip (14.7 KB)Acknowledgments
The authors would like to thank My Yang and Nhungoc Ti Luong for their assistance during the laboratory procedures and Dr. Tim Snider and Dr. Ana Alba for their technical support during this project.
Funding
This material was made possible, in part, by funding from the United States Department of Agriculture National Institute of Food and Agriculture (MIN-62-058) (Agricultural Experimental Station).
References
- Alonso, C., Raynor, P. C., Davies, P. R., and Torremorell, M. (2015). Concentration, Size Distribution, and Infectivity of Airborne Particles Carrying Swine Viruses. PLoS ONE, 10(8):e0135675.
- Alonso, C., Raynor, P. C., Davies, P. R., and Torremorell, M. (2016). Evaluation of an Electrostatic Particle Ionization Technology for Decreasing Airborne Pathogens in Pigs. Aerobiologia, 32(3):405–419.
- Agranovski, I. E., Safatov, A. S., Pyankov, O. V., Sergeev, A. A., Sergeev, A. N., and Grinshpun, S. A. (2005). Long-Term Sampling of Viable Airborne Viruses. Aerosol Sci. Technol., 9:912–918.
- Appert, J., Raynor, P. C., Abin, M., Chanderb, Y., Guarinob, H., Goyal, S. M., Zuo, Z., Gec, S., and Kuehn, T. H. (2012). Influence of Suspending Liquid, Impactor Type, and Substrate on Size-Selective Sampling of MS2 and Adenovirus Aerosols. Aerosol Sci. Technol., 46(3):249–257. doi:10.1080/02786826.2011.619224.
- Blachere, F. M., Lindsley, W. G., Slaven, J. E., Green, B. J., Anderson, S. E., Chen, B. T., and Beezhold, D. H. (2007). Bioaerosol Sampling for the Detection of Aerosolized Influenza Virus. Influenza Other Respir. Viruses, 1(3):113–120.
- Brookes, S., Choudhury, B., Matrosovich, M., Essen, S., Núñez, A., Clifford, D., Slomka, M., Kuntz Simon, G., Garcon, F., Nash, B., Hanna, A., Gardner, R., Quéguiner, S., Chiapponi, C., Bublot, M., Garcia, J., Foni, E., Loeffen, W., Larsen, L., Van Reeth, K., Banks, J., Irvine, R., Brown, I., and Brown, J. (2010). Replication, Pathogenesis and Transmission of Pandemic (H1N1) 2009 Virus in Non-Immune Pigs. PLoS ONE, 5(2):e9068.
- Cho, J., Dee, S., Deen, J., Guedes, A., Trincado, C., Fano, E., Jiang, Y., Faaberg, K., Collins, J., Murtaugh, M., and Joo, H. (2006). Evaluation of the Effects of Animal Age, Concurrent Bacterial Infection, and Pathogenicity of Porcine Reproductive and Respiratory Syndrome Virus on Virus Concentration in Pigs. Am. J. Vet. Res., 67(3):489–493.
- Cho, J., Deen, J., and Dee, S. (2007). Influence of Isolate Pathogenicity on the Aerosol Transmission of Porcine Reproductive and Respiratory Syndrome Virus. Can. J. Vet. Res., 71(1):23–27.
- Cooper, C., Slagley, J., Jr Lohaus, J., Escamilla, E., Bliss, C., Semler, D., Felker, D., Smith, D., and Ott, D. (2014). Comparison of High-Volume Air Sampling Equipment for Viral Aerosol Sampling During Emergency Response. J. Emerg. Manag., 12(2):161–170.
- Corzo, C. A., Culhane, M., Dee, S., Morrison, R. B., and Torremorell, M. (2013). Airborne Detection and Quantification of Swine Influenza a Virus in Air Samples Collected Inside, Outside and Downwind from Swine Barns. PLoS One, 8(8):e71444.
- Corzo, C. A., Allerson, M., Gramer, M., Morrison, R. B., and Torremorell, M. (2014). Detection of Airborne Influenza a Virus in Experimentally Infected Pigs with Maternally Derived Antibodies. Transbound. Emerg. Dis., 61(1):28–36.
- Dzubay, T. G., Hines, L. E., and Stevens, R. K. (1976). Particle Bounce Errors in Cascade Impactors. Atmos. Environ., 10:229–234.
- EPA. (2009). Aerosol Wind Tunnel Testing Task 2: Measurement of Spore Extraction Efficiency. National Homeland Security Research. Research Triangle Park, NC: RTI International.
- FAO. (2016). Agricultural and Consumer Protection Department. Animal Production and Health-Sources of Meat. Available at http://www.fao.org/ag/againfo/themes/en/meat/backgr_sources.html.
- Ge, S., Kuehn, T. H., Abin, M., Verma, H., Mor, S. K., Goyal, S. M., Appert, J., Raynor, P. C., and Zuo, Z. (2014). Airborne Virus Survivability During Long-Term Sampling using a Non-Viable Andersen Cascade Impactor in an Environmental Chamber. Aerosol Sci. Technol., 48:1360–1368.
- Gloster, J., Redington, A., Burgin, A., Sorensen, L., Sørensen, J., Turner, J., Dillon, R., Hullinger, M., Simpson, P., Astrup, M., Garner, P., Stewart, G., D'Amours, P., Sellers, R., and Paton, D. (2010). Airborne Spread of Foot-and-Mouth Disease–Model Intercomparison. Vet. J., 183(3):278–286.
- Mengeling, W. L., Vorwald, A. C., Lager, K. M., and Brockmeier, S. L. (1996). Diagnosis of Porcine Reproductive and Respiratory Syndrome using Infected Alveolar Macrophages Collected from Live Pigs. Vet. Microbiol., 49(1–2):105–115.
- Nicas, M., Nazaroff, W. W., and Hubbard, A. (2005). Toward Understanding the Risk of Secondary Airborne Infection: Emission of Respirable Pathogens. J. Occup. Environ. Hyg., 2:143–154.
- Otake, S., Dee, S., Corzo, C., Oliveira, S., and Deen, J. (2010). Long-Distance Airborne Transport of Infectious PRRSV and Mycoplasma Hyopneumoniae from a Swine Population Infected with Multiple Viral Variants. Vet. Microbiol., 145(3–4):198–208.
- Rao, A. K., and Whitby, K. T. (1978). Non-Idea Collection Characteristics of Inertial Impactors-II Cascade Impactors. J. Aerosol Sci., 9:87–100.
- Stark, K. D. C. (1999). The Role of Infectious Aerosols in Disease Transmission in Pigs. Vet. J., 158(3):164–181.
- Thomas, Y., Vogel, G., Wunderli, W., Suter, P., Witschi, M., Koch, D., Kaiser, L., and Tapparel, C. (2008). Survival of Influenza Virus on Banknotes. Appl. Environ. Microbiol., 74:3002–3007.
- Torremorell, M., Alonso, C., Davies, P. R., Raynor, P. C., Patanayak, D., Torchetti, M., and McCluskey, B. (2016). Investigation into the Airborne Dissemination of H5N2 Highly Pathogenic Avian Influenza Virus during the 2015 Spring Outbreaks in the Midwestern U.S. Avian Dis., 60:637–643.
- Tiwari, A., Patnayak, D. P., Chander, Y., Parsad, M., and Goyal, S. M. (2006). Survival of Two Avian Respiratory Viruses on Porous and Nonporous Surfaces. Avian Dis., 50:284–287.
- Verreault, D., Moineau, S., and Duchaine, C. (2008). Methods for Sampling of Airborne Viruses. Microbiol. Mol. Biol. Rev., 72(3):413–444.
- Zuo, Z., de Abin, M., Chander, Y., Kuehn, T., and Goyal, S. (2013a). Comparison of Spike and Aerosol Challenge Tests for the Recovery of Viable Influenza Virus from Non-Woven Fabrics. Influenza Other Respir. Viruses, 7(5):637–644.
- Zuo, Z. L., Kuehn, T. H., Verma, H., Kumar, S., Goyal, S. M., Appert, J., Raynor, P. C., Ge, S., and Pui, D. Y. H. (2013b). Association of Airborne Virus Infectivity and Survivability with its Carrier Particle Size. Aerosol Sci. Technol., 47(4):373–382. doi:10.1080/02786826.2012.754841.