ABSTRACT
Energetic materials generating biocidal combustion products to disable airborne pathogenic microorganisms (including bio-threat agents) were designed as compounds of halogens and metals with high heats of oxidation. Thermally stable Al-based powders containing iodine and chlorine were prepared using ball-milling at room and cryogenic temperatures. Such powders can replace pure aluminum in metallized energetic formulations. Their stability and halogen release were quantified using thermo-gravimetric analysis. Ignition temperatures were determined by coating prepared powders onto an electrically heated filament. All prepared composites had lower ignition temperatures and longer combustion times compared to pure Al. In separate experiments, combustion products generated by injecting the prepared powders into an air-acetylene flame were mixed with a well-characterized bioaerosol. Inactivation of viable bioaerosol particles exposed to the heated combustion products for a short period of time (estimated to be 0.33 s) was quantified. The combustion products of materials investigated in this study effectively inactivated the aerosolized spores of two tested surrogates of Bacillus anthracis (B. atrophaeus and B. thuringiensis var kurstaki). A ternary composite with 20 wt% of iodine, 40 wt% of aluminum and 40 wt% of boron was found to be most attractive based on both its stability and efficiency in inactivating the aerosolized spores. The inactivation achieved was primarily attributed to chemical stresses as the thermal effect could not solely produce the high measured levels of inactivation. The findings point to a possible synergy of the thermal and chemical spore inactivation mechanisms.
© 2017 American Association for Aerosol Research
EDITOR:
Introduction
Anthrax caused by Bacillus anthracis (BA) endospores is a potential global threat in the event it is used as bioweapon (D'Amelio et al. Citation2015). Substantial efforts have recently been directed towards the development of new agent defeat weapon systems (Nelson Citation2003), which are intended to be used against stockpiles of BA spores and similar biological agents. An explosive charge may disperse stored BA spores, some of which might escape the fireball and potentially contaminate large areas (Caldicott and Edwards Citation2002; Kerwat et al. Citation2010). It is therefore desired for such an explosive to contain reactive materials that generate biocidal combustion products capable of inactivating dispersed BA spores and other aerosolized biological weapon agents (Clark and Pantoya Citation2010; Farley and Pantoya Citation2010; Feng et al. Citation2013, Grinshpun et al. Citation2012, Citation2010b; Sullivan et al. Citation2011, Citation2013; Zhang et al. Citation2010a; Zhou et al. Citation2015). Among many types of reactive materials under development, powders based on metal fuels and incorporating a halogen may be most easily implemented in existing energetic formulations as drop-in replacements for currently used aluminum powders. Several such halogen-bearing materials employing aluminum as a metal fuel have recently been prepared using mechanical alloying, including binary powders of Al·I2 (Zhang et al. Citation2010a) and Al·CHI3 (Zhang et al. Citation2012), and ternary Al·B·I2(Aly et al. Citation2014; Abraham et al. Citation2015). Along with these materials, a facility and experimental protocols were developed specifically to compare the biocidal effectiveness of their respective combustion products (Grinshpun et al. Citation2010b). This methodology relies on mixing a biological aerosol containing a BA surrogate with products of a hydrocarbon flame seeded with the reactive material powder particles. The bioaerosol is exposed to the combustion products for a limited, well-specified time before the spores are collected for the culture-based analysis aiming at assessing their post-exposure viability, which is compared to the initial one. For meaningful comparisons, the operating conditions are selected to achieve a well-quantified effect for each of the reactive materials. For the effect to be quantifiable, at least some measurable fraction of the spores exposed to the combustion products must survive.
In laboratory studies, it is common to use biowarfare simulants of BA, which are not pathogenic (Greenberg et al. Citation2010; Sinclair et al. Citation2012). Conventionally used surrogates are Bacillus atrophaeus (or Bacillus globigii var niger), commonly abbreviated as BG (Gibbons et al. Citation2011), and Bacillus thuringiensis var kurstaki, usually abbreviated as Bt(k) (Tufts et al. Citation2014). The inactivation of aerosolized spores of both BA surrogates by products released during combustion of several reactive materials has been studied previously using the same methodology as employed here (Aly et al. Citation2014; Grinshpun et al. Citation2012; Adhikari et al. Citation2016). In parallel, combustion dynamics of the prepared reactive material powders have been characterized, focusing on the ignition temperatures and burn times of composite, halogen containing particles (Adhikari et al. Citation2016; Aly et al. Citation2014; Zhang et al. Citation2010a; Abraham et al. Citation2013; Wang et al. Citation2016a). Reliable data on both the combustion dynamics and biocidal properties of new reactive materials are critical in supporting research on development of new explosive formulations and on modeling their energetic performance and effectiveness against biological agents (Henderson et al. Citation2015). This work extends the previous efforts dealing with the development of aluminum-based fuel additives generating biocidal combustion products (Abraham et al. Citation2013; Grinshpun et al. Citation2012; Zhang et al. 2012a, Citation2010a; Abraham et al. Citation2014). New materials are prepared and characterized using previously established methods and techniques. Results obtained with the new materials are presented and compared with those reported earlier. A comprehensive description is provided for the combustion dynamics and biocidal effectiveness of the combustion products of aluminum-based halogen-bearing fuel additives.
Materials and methods
Materials
Starting materials used to prepare different composite materials were aluminum powder, −325 mesh size (<45 µm), 99.5% pure (Atlantic Equipment Engineers, Upper Saddle River, NJ, USA), amorphous boron powder (<1 µm), 93-96% pure (SB Boron, Bellwood, IL, USA), iodoform powder, 99% pure, niobium chloride, 99% pure, and titanium iodide, 99.9% pure (all from Alfa Aesar, Ward Hill, MA, USA), and iodine chips (approximately 1–5 mm in size), 99% pure (Sigma Aldrich, Allentown, PA, USA).
Composite powders were prepared by ball milling. Ternary Al·B·I2 composites were prepared using a shaker mill (8000 series by SPEX Certiprep, Metuchen, NJ, USA) with two 50 mL flat-ended steel vials cooled by room-temperature air jets; details of the powder preparation are available elsewhere (Aly et al. Citation2014). Each vial was loaded with 5 g of the starting powder blend and 10-mm diameter case-hardened carbon steel balls. The ball to powder mass ratio (BPR) was fixed at 10. The vials were loaded and sealed inside an argon-filled glovebox. Materials with varied Al/B ratio but with a fixed concentration of iodine at 20 wt. % were prepared and evaluated. It was noted that keeping the prepared Al·B·I2 powders in an argon-filled glovebox for as little as seven days makes the powders more stable, i.e., powders were retaining their weight better upon heating, as discussed below. Respectively, some powders were held in the argon-filled glovebox for one month prior to further evaluation; such powders are referred to as “argon aged.”
Multiple composite materials, including Al·I2 (Abraham et al. Citation2014), Al·CHI3 (Zhang et al. 2012a), Al·TiI4 and Al·NbCl5 were prepared by cryogenic milling using liquid nitrogen at −196°C as a cooling agent. A model 01HD attritor mill (Union Process, Akron, OH, USA), was used. Liquid nitrogen was flown at approximately 2 CFM (0.94 L/s) within an insulated cooling jacket around the 750-mL stainless steel vial. The powder mass was 50 g; the BPR was 36; 10 mm-diameter case-hardened carbon steel balls were used. An impeller rotated at 400 rpm and the milling time was 24 h.
An additional, room temperature milling step using the 8000 series shaker mill was used to coat both Al·TiI4 and Al·NbCl5 powders with 5 wt. % of Teflon™ (Teflon™ PTFE 7A, Dupont). The powders were milled for 30 min using 3 mm-diameter case-hardened carbon steel balls with a BPR of 5. Typically, softer materials, such as PTFE, coat harder particles, such as metal-based composites employed here. The effectiveness of this treatment was determined by the powder stability measurements as described below. A quantitative microscopic assessment of the coating was not attempted.
Three prepared materials, specifically Al·B·I2, Al·I2 and Al·CHI3, contained a fixed amount of 20 wt.% of iodine. The net iodine content was 0.79 mmol/g. The powders of Al·TiI4 and Al·NbCl5 contained 44 and 26 wt. % of TiI4 and NbCl5, respectively. The corresponding halogen contents were 1.58 mmol/g of iodine and 2.46 mmol/g of chlorine.
Experimental design and methods
The stability of halogens encapsulation into the Al-matrix at low temperatures typical of storage and handling of these materials and the release of these halogens upon further heating were investigated utilizing thermo-gravimetric (TG) analysis. The samples were heated in argon to 1000°C using a model Q5000IR TG analyzer (TA Instruments, New Castle, DE, USA). Sample masses ranging from 10 to 25 mg were loaded into the instrument in an alumina crucible. Both, the balance and furnace were purged with 100 mL/min of argon at 30°C for at least 500 min before starting the experiment. High gas flow rates and long flushing times were used to purge oxygen from the furnace, which could not be evacuated. During the measurements gas flow rates were reduced to 20 and 50 mL/min for the balance and furnace, respectively. Measurements were performed at a fixed heating rate of 10 K/min for all powders.
Ignition of the powders was characterized in air using a heated filament experiment described in detail elsewhere (Shoshin et al. Citation2006; Ward et al. Citation2006). A slurry of each powder in hexane was made to prepare a thin, 10-mm long coating on 0.5 mm diameter nickel-chromium alloy wire with a length of 45 mm. The coated wire was heated by a DC current. Varied applied voltage and adjustable resistors connected in series with the wire were used to vary the heating rates in a range of 103–105 K/s. The temperature of the filament was measured using a high-speed infrared pyrometer (DP1581, Omega Engineering, Inc., Stamford, CT, USA) focused on an uncoated filament surface adjacent to the powder coating. The emission from the powder coating was visualized using a high-speed video camera (MotionPro 500, DEL Imaging Systems, Cheshire, CT, USA), operated at 500 fps. Prior to ignition, the coated fraction of the filament surface was darker than the surface of the clean nickel-chromium filament. The ignition instant was registered when the powder coating became brighter than the clean filament.
In two separate experiments, powders were injected in a premixed air-acetylene flame using a custom-designed gas burner described in detail elsewhere (Ward et al. Citation2006; Corcoran et al. Citation2015). In one experiment, which was performed to characterize the particle combustion, the burner was operated in room air. Particle optical emission was monitored using a photomultiplier tube (PMT) equipped with a 700-nm interference filter. The wavelength was selected to avoid characteristic molecular emission associated with AlO and typically occurring in aluminum combustion. The recorded optical emission pulses were processed to determine their durations, interpreted as burn times. Particle size distributions of the composite powders were determined separately by dynamic light scattering using a Coulter LS230 analyzer. Durations of the emission pulses produced by burning powder particles were then correlated with the size distributions to determine burn times as a function of the particle size. In the other experiment, aimed at quantifying the biocidal properties of the prepared materials, the same burner was mounted inside an enclosed gas flow system, as illustrated in and described in more detail elsewhere (Grinshpun et al. Citation2012; Aly et al. Citation2014). The same reactive material powders were injected into the flame, thus generating the same combustion products above the burner. An air flow carrying spore aerosol generated using a six-jet Collison nebulizer (BGI Inc., Waltham, MA, USA) operating at a flow rate of 6 L min−1 and diluted with a HEPA-filtered dry air flow of 30 L min−1 was passed through the exposure chamber above the burner. Based on the flowrates used, the exposure time of spores to the combustion products is estimated to be close to 0.33 s (Aly et al. Citation2014). This is comparable to the time of decay for fireballs produced by various types of conventional munitions (Orson et al. Citation2003). After being exposed to the combustion products and cooled down in a 300-mm long cooling system, the bioaerosol was sampled into three identical gelatin filter cassettes (25-mm dia) (SKC Inc. Eighty Four, PA, USA) and analyzed using the culture-based method. Similar test was conducted with no burner operating (control). This design allowed determining the effect of combustion products generated by different fuels on the spore viability by comparing the combustion-exposed and non-exposed (control) values of culturable concentrations. The ratio of control-to-exposed colony forming unit (CFU) counts is the Inactivation Factor (IF) conventionally used to quantify the viability loss. In the experiment producing combustion exposed spore samples, the burner could be mounted at different vertical positions (not shown in ). Moving the burner higher up (or closer to the horizontal tube carrying the bioaerosol) resulted in a higher temperature in the mixing volume. Experiments were performed with two burner positions, described by the distance from the tip of the burner to the axis of the horizontal tube carrying the bioaerosol flow. These two distances, 325 and 415 mm, are referred to as “high” and “low” positions of the burner, respectively. These positions were selected to mix the bioaerosol with combustion products at temperatures above and below the iodine boiling point of 184.3°C. Average temperatures in the mixing volume corresponding to the high and low positions of the burner were about 259 and 170°C, respectively (Aly et al. Citation2014). Note that the feed rate of reactive powders into the flame was sufficiently low to not noticeably affect the heat release of the air-acetylene flame and thus to not disturb the temperature field above the burner. Thus, nearly the same temperatures in the bio-aerosol mixing volume were obtained with all materials in experiments employing two burner positions described above.
Figure 1. Experimental setup for testing biocidal effect of combustion products of different materials on the aerosolized spores.
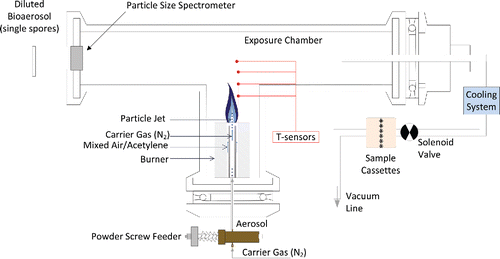
Preparation of microbial suspensions
Freeze-dried BG spores were acquired from the US Army Edgewood Laboratories (Aberdeen Proving Ground, MD, USA). Freeze-dried spores of B. thuringiensis serovar kurstaki, Bt(k), strain SA-11 (product # SA-11 SDTC; technical grade concentrate developed for US Army and Air Force) were acquired from Certis USA Inc. (Columbia, MD, USA). Both types of spores are well-characterized as they have been extensively used as simulants of bio-warfare agents (Faille et al. Citation1999; Beuchat et al. Citation2005; Rice et al. Citation2005; Helfinstine et al. Citation2005; Luna et al. Citation2008). The originally purchased Bt(k) had approximately 65% protein crystals (referred to as insecticidal crystal proteins), which were removed as a part of the purification procedure since the toxic insecticidal proteins could influence the spore culturability. To achieve an adequate (>98%) purification level, freeze-dried spores were suspended in 50% EtOH (pH 9.0), washed 2-3 times by using centrifugation until the supernatant was clear, and re-suspended in cold acetone for about 12 hours in a refrigerator. The suspension was subsequently filtered, dried, and crushed into dry powder in a mortar and pestle. As part of preparation of the suspension for aerosolization, the BG and purified Bt(k) powders were suspended in sterile filtered deionized water, vortexed to remove clumps (verified by microscopy), centrifuged at approximately 6,300×g for 7 min, and washed again twice. The concentration of viable spores in the suspension was 107–1010 CFU/mL. The latter was determined after making serial dilutions and cultivating aliquots on tryptic soy agar (TSA, BD, Franklin Lakes, NJ, USA) at 30°C for BG and 37°C for Bt(k) for 24 h.
Analysis of samples and data analysis
The spores collected on the filters (both exposed and control) were extracted by intermittent vortexing for 2 min in 10 mL of sterile filtered de-ionized water. Aliquots of filter extracts were cultivated on TSA medium followed by incubation at 30°C or 37°C, depending on the species, for approximately 24 h. Aliquot volumes ranging from 200 µL to 5 mL were used. It is acknowledged that the viability (culturability) of damaged spores may depend on the culture medium; for example, some heat-treated species may become salt-sensitive. From this perspective, it is important to emphasize that we chose a commonly used “standard” TSA medium (containing 5 g of NaCl per liter). The highest volumes were chosen when the colony counts were expected to be close to the limit of quantification due to high microbial inactivation by combustion products (discussed and established earlier in Grinshpun et al. Citation2010a,Citationb). After incubation, the CFU enumeration was performed for every sample – exposed and control. For a specific run, an average CFU value per sample was determined from at least three replicate counts on each of the three identical filters. Repeated runs (in some cases, 4 to 6 runs per condition) were performed to minimize an influence of occasional sample contamination and other issues on the outcome. Based on the sampling flow rate and sampling time, the airborne concentration of culturable spores (CFU/m3) was determined. Accordingly, the IF value was calculated for each control-to-exposure ratio of the concentrations. With the data log-normally distributed, the geometric mean (GM) of IF as well as the geometric standard deviation (GSD) was calculated. The spore inactivation levels produced by different materials were compared using a two-tail, two-sample unequal variance t-test. A p-value of 0.05 or less was considered to identify statistically significant differences.
Results and discussion
Halogen release and stability of composite materials
TG traces quantifying how the volatile halogens are released as a function of temperature are presented in for all powders. The data shown in combine results published previously for Al·I2 (Zhang et al. Citation2010a,b,), Al·CHI3 (Zhang et al. Citation2012), and Al·B·I2 (Abraham et al. Citation2015; Aly et al. Citation2014) with new measurements conducted using Al·TiI4 and Al·NbCl5. In the figure, the vertical axis applies to the top curve only. For all materials, a relatively minor and slow weight loss occurs at low temperatures, when elemental iodine (boiling point 184.3°C), or other halogenated compounds used as starting materials, i.e., CHI3 (boiling point 218°C), TiI4 (boiling point 377°C), and NbCl5 (boiling point 248.2°C), are expected to volatilize. As only a relatively small fraction of the total observed weight loss occurs at such low temperatures, only a small portion of the starting halogenated compounds remains unbound to the metal or metalloid matrix after milling. Sharper weight loss steps are typically observed at temperatures higher than 400°C, which signify release of halogenated compounds that have been stabilized and thus bound to the metal matrix. Preliminarily, it was observed that the low-temperature weight loss was very significant for uncoated powders of Al·TiI4 and Al·NbCl5. After the Teflon™ coating was applied, the low-temperature weight loss for Al·NbCl5 substantially diminished. However, it remained fairly strong for Al·TiI4, even after that material was coated with Teflon™.
Figure 2. TG traces of Al-based composites at 10 K/min, prepared using room and cryogenic temperature milling. Numbers in parentheses under “Al·B·I2” indicate weight percentages for Al, B, and I2, respectively. Al·B·I2 powders were not aged for these measurements. The vertical axis applies to the top curve. All other curves are stacked vertically downward to help visually distinguish them. Each curve represents one measurement.
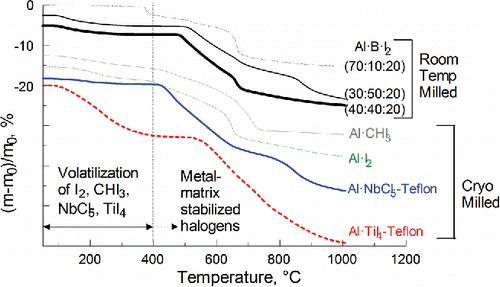
Handling and storage of the prepared powders and reactive compositions, in which they might serve as additives, is not expected to require high temperatures. Previously, it was reported that loosely bound iodine may be released at temperatures below 400 °C, while iodine that was stabilized more effectively, evolved as gas only at higher temperatures (Aly et al. Citation2014). A similar pattern is observed in . Thus, it becomes possible to consider a relative, pragmatic measure of material stability, S, previously introduced for Al·I2 composites (Aly et al. Citation2014). The parameter S was defined as the fraction of weight loss at temperatures exceeding 400°C (673 K): . It was used to rank all prepared powders as presented in : each bar, including the bars for coated and aged materials, corresponds to one TG measurement (). With the exception of one material, Al·TiI4, S is above 80% for all powders. In addition to data collected for the powders once they were prepared, changes in their stability caused by Teflon™ coating for Al·TiI4 and Al·NbCl5 and by initial aging for Al·B·I2 in argon are communicated with differently colored bars. Teflon™ coating helped to somewhat improve stability for the powders of Al·TiI4 and Al·NbCl5. However, the Al·TiI4 composite remained much less stable compared to other materials. This composite was difficult to handle and store for extended periods, making it impractical. Consequently, it was not used in further experiments. For the remaining materials, stabilities were close to one another.
Ignition
Ignition temperatures as a function of heating rate for all composites are shown in . The legend here and in the following figures presents all materials in a table-format, listing relative weight fractions of different elemental components. For reference, data for pure Al powder are also included (Zhang et al. Citation2012). Pure aluminum ignites at higher temperatures, compared to all the composite powders. Several materials, including unaged Al·B·I2 with 40 and 50 wt.% of boron, Al·NbCl5, and Al·I2 ignite within a very narrow range of temperatures, increasing from ca. 600 to 800°C as a function of the heating rate. The ternary Al·B·I2 with 10 wt.% of boron ignites at slightly elevated temperatures, approaching those of pure aluminum. The lowest ignition temperatures, around 500°C, are observed for Al·CHI3. The hitherto unpublished ignition temperatures for the Al·NbCl5 composite are very similar to those of the other composite powders. The obtained ignition temperatures as a function of the heating rate can be used to predict when different reactive material particles ignite while being heated in a fireball produced by an explosion. This further enables prediction of the dynamics of both heat and halogen release occurring while the particles are burning. The rates of both processes can be assessed accounting for the particle burn times discussed below.
Figure 4. Ignition temperatures of Al-based composites obtained in the heated filament experiments. The ignition temperature were reported in the following references: pure Al, Al·I2 (Zhang et al. Citation2012), Al·B·I2(70:10:20) (Aly et al. Citation2014), Al·B·I2(40:40:20) (Wang et al. Citation2016a), Al·B·I2(30:50:20) (Wang et al. Citation2016a), and Al·CHI3 (Abraham et al. Citation2014). New data on Al·NbCl5 are also presented. Each point shows an average value and the bars show a standard deviation for 3-5 individual measurements.
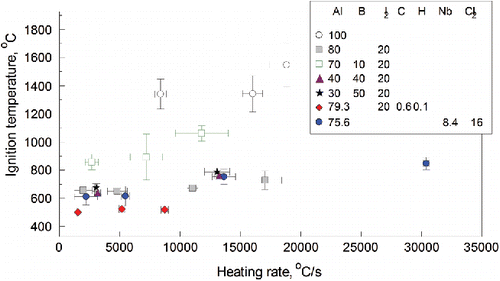
Particle combustion in a laminar flame
Particle size distributions of the composite materials prepared in this effort and used in the combustion experiments are shown in . All powders included particles with sizes in the range of 1–10 µm, while some powders contained coarser and/or finer particles as well.
Figure 5. Particle size distributions for composite materials used in the combustion experiments. Each distribution represents a single measurement.
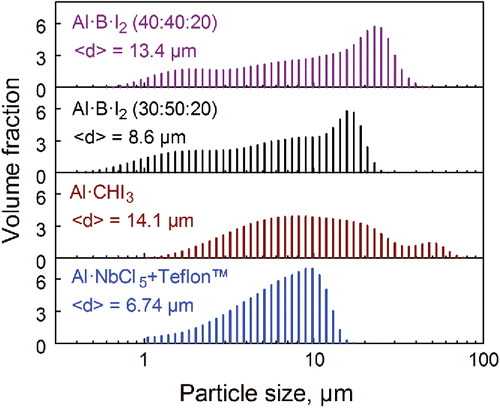
The durations of the recorded optical emission pulses were measured and a histogram of the pulse durations, interpreted as particle burn times, was obtained for each material, as described in detail elsewhere (Corcoran et al. Citation2013a,Citationb, Citation2015; Wang et al. Citation2015, Citation2016b). The burn time distributions were determined from 500 – 1000 individual burning particles, and then correlated with the corresponding particle size distributions shown in . Assuming that larger particles burn longer, correlations between the two distributions generated trends attributable to the effect of particle sizes on their burn times for all materials. These trends are shown in for all materials, for which this processing was performed, i.e., Al·CHI3, Al·B·I2, and Al·NbCl5 composites. For comparison, the burn times measured using the same methodology for a pure spherical Al powder (Wang et al. Citation2016b) are shown as well. Results indicate that all powders burn more slowly than pure Al. The effect of particle size d on the burn time t may be approximately described by a power law, , with exponents n in the narrow range of 0.50 – 0.56 for all samples except Al·CHI3, for which the exponent was 0.64. As noted above, these data are useful for predicting the rates of release of heat and halogen-bearing combustion products by reactive material particles of different sizes burning in a fireball.
Exposure of aerosolized BG and Bt(k) spores to combustion products
Biocidal effectiveness of the materials selected based on the characterization effort described above was evaluated using two challenge bioaerosols, BG and Bt(k) spores. All tests were performed at the same powder delivery rate, which translated into mass concentrations estimated at 35 to 42 mg per L of air, depending on the powder.
show the inactivation data obtained for both Bacillus species in experiments performed at lower temperatures (with the burner at low position) and higher temperature conditions (burner is at high position), respectively. In addition to the original results, the figures demonstrate—as reference points—IF values previously reported for unseeded flame as well as for the flame seeded with pure Al powder. The figures also contained some data that we published earlier for Al·I2 and Al·B·I2(70:10:20) (for BG only) (Aly et al. Citation2014).
Figure 7. Inactivation of aerosolized BG and Bt(k) spores by combustion products of different materials at low position of the burner (Tair ≈ 170°C). Each bar represents the geometric mean value with the error bars representing the geometric standard deviation calculated from 18 to 53 measurements (depending on the material tested). Estimated exposure time = 0.33 s; powder delivery concentration = 35 to 42 mg per L of air (depending on the powder).
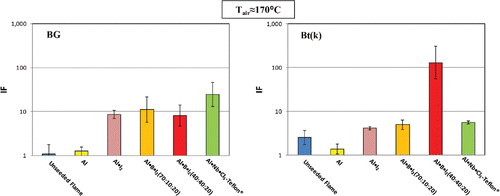
Figure 8. Inactivation of aerosolized BG and Bt(k) spores by combustion products of different materials at high position of the burner (Tair ≈ 259°C). Each bar represents the geometric mean value with the error bars representing the geometric standard deviation calculated from 21 to 44 measurements (depending on the material tested). Estimated exposure time = 0.33 s; powder delivery concentration = 35–42 mg per L of air (depending on the powder).
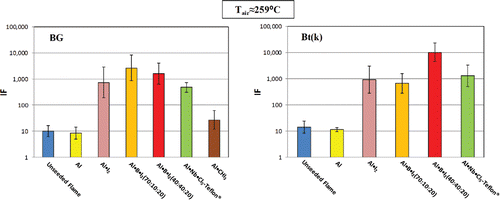
It is seen from that although the tested composites generally showed higher inactivation compared to unseeded flame and pure Al, the measured spore viability loss was not tremendous: IF was mostly around 10, except Al·B·I2(40:40:20) which generated IF∼102 for Bt(k). With the burner at lower position, the average air temperature in the exposure chamber was just below the boiling point of iodine. Thus, at least partially, the spore-inactivating iodine released during combustion of a composite was expected to condense. The observed limited biocidal effect can still be attributed to the chemical interaction involving iodine remaining in the gas phase, because no measurable thermal inactivation was expected at the present air temperatures (Grinshpun et al. Citation2010a). Minor differences between BG and Bt(k) spores were observed in response to combustion products released by the same material, except for Al·B·I2(40:40:20), which, as mentioned above, was about an order of magnitude more effective in inactivating Bt(k) than BG spores.
As expected, the IF-values obtained at high position of the burner () were by far greater than those obtained the lower position. To some extent, this reflects the contribution of heat stress to the spore inactivation. However, even at higher temperature (Tair ≈ 259°C) with the short bioaerosol exposure times occurring in the present tests, the heat-induced stress may lead to IF∼10 (Grinshpun et al. Citation2010a), as also seen for the unseeded or aluminum-seeded flames. Clearly, the IF∼103 and higher observed in is an evidence of chemical effect of halogen-containing combustion products. In particular, iodine remains in the vapor phase, making its interaction with the aerosolized spores much more effective. This finding is consistent with earlier results (Aly et al. Citation2014, Grinshpun et al. Citation2012).
At higher air temperature, combustion of Al:B:I2(40:40:20) generated IF as high as 104 for the Bt(k) bioaerosol, which appeared more susceptible to this composite than BG. No major between-species variation was identified for other materials under this condition. In order to explain the between-species differences found for Al:B:I2(40:40:20), we first hypothesized that the purification of Bt(k) performed before the experiment for crystal removal could have damaged the spore coat, making the spores more susceptible. However, additional experiments aiming at comparing the germination potential of Bt(k) before and after the purification procedure did not provide appropriate evidence to support this hypothesis. The difference is likely to relate to specific microbiological properties of BG and Bt(k) spores which remain to be identified in future studies. This question is important particularly given that both species are used as surrogate for B. anthracis. The analysis of data presented in shows that the products of combustion of Al·B·I2(40:40:20) caused a significantly stronger biocidal effect on the aerosolized viable Bt(k) spores than Al·I2. At the same time, when testing with BG spores, the difference between IFs obtained for these two materials was not as significant. It is noted that Al·B·I2 produced a superior biocidal effect although this composite yields the same concentration of iodine as Al·I2 and has lower burn rates (). This suggests the importance of slow release of iodine in the combustion products for inactivation of aerosolized spores. It is also noted that the weight ratio of components in Al·B·I2 (mostly changing Al/B ratio) did not affect inactivation of BG spores while it significantly impacted IF for Bt(k) spores. The differences can be attributed to the effect provided by boron rather than iodine. In addition to the Bt(k) data obtained with the burner at high position, the above point is supported by an abnormal IF value measured at lower air temperatures when the effect of iodine must have been substantially reduced. We anticipate that kinetics of iodine release is qualitatively different in the materials with higher boron concentration. A slower, more gradual release of iodine from boron (boron burns slower than aluminum) may enhance the mixing of iodine-bearing gas species with the challenge bioaerosol. It is possible that boron combustion products have biocidal effects of their own. It is also possible that aside from I2 and HI, which are expected to be produced, new boron and iodine-bearing biocidal gas species are generated by burning materials with higher boron concentrations. Thermodynamics of the respected phases are not well known; additional work is needed to identify the specific products generated and to establish their relative biocidal properties.
Another aluminum-based formulation developed and tested in this study, Al-NbCl5, was found to produce rather high inactivation effect against BG and B(k). The IF values were comparable to those obtained for Al·I2. Its biocidal efficiency was lower than that for the “leading composite”, Al·B·I2(40:40:20), when testing with the burner at high position. The mole fraction of chlorine in Al-NbCl5 is higher than that of iodine in all reference iodine-bearing materials. In addition, a minor contribution of the fluorine contained in the Teflon™ coating cannot be conclusively ruled out. Therefore, it is concluded that iodine molecules or iodine-bearing gases are potentially more biocidal than molecules of chlorine or chlorine-containing gas species.
Among the tested composites, Al·CHI3 revealed the weakest effect towards inactivating BG spores; this material was initially tested only with BG and at the high burner position (the most conservative assessment); since it was not found a promising candidate, further testing involving this composite was discontinued.
In summary, at the temperatures just below the iodine boiling point all aluminum-based materials containing iodine, except Al·B·I2(40:40:20), exhibited IF-values higher–by about an order of magnitude–than reference unseeded or seeded by Al flames [the difference was greater for BG compared to Bt(k)]. Combustion of Al·B·I2(40:40:20) under this condition generated a greater, (approximately 100-fold) inactivation increase. The IF values become three to four orders of magnitude higher than for the reference flames at the temperatures exceeding the boiling point of iodine. The spore inactivation achieved in these experiments is believed to be primarily associated with the chemical interaction of spores with halogen-containing gas species because the heat solely was not sufficient to produce such high IF values. Additional synergy of the thermal and chemical spore inactivation mechanisms should be further investigated and taken into consideration in future efforts to develop materials with effective biocidal combustion products.
Conclusions
Aluminum-based composite powders containing halogens were prepared using mechanical milling at both room and cryogenic temperatures. TG traces showed significant amount of halogens retained within the materials heated above 400°C, indicative of thermally stable composites. For Al·NbCl5 and Al·TiI4, a Teflon™ coating was used to reduce the low-temperature halogen release.
Ignition temperatures of the prepared Al-based composite powders were between 600 and 800°C, consistently lower than for pure Al. For particles injected in an air-acetylene flame, the individual particle combustion times for all materials were longer than for pure Al. The longest burn times were observed for ternary, Al·B·I2 composites.
Combustion products generated by powders injected into the air-acetylene flame were cooled to different temperatures and mixed with a well-characterized bioaerosol (spores of two tested surrogates of Bacillus anthracis: B. atrophaeus and B. thuringiensis var kurstaki). Inactivation of viable spores exposed at different temperatures to the combustion products for a short period of time (estimated to be 0.33 s) was quantified. The combustion products were found capable of effectively inactivating the aerosolized spores at temperatures both below and above the iodine boiling point. The biocidal effect was substantially stronger at higher temperatures when iodine primarily remains in the gaseous phase. Different composite materials demonstrated different biocidal characteristics. Based on the number of produced halogen-bearing gas molecules, chlorine-containing materials generate a weaker biocidal effect than the iodine-containing materials. Presence of boron was found to enhance the inactivation effect for the aluminum-based iodine-containing materials. A ternary composite with 20 wt% of iodine and 40 wt% of aluminum and boron each was found to be most attractive based on both its stability at low temperatures and effectiveness of its combustion products for inactivating the viable aerosolized spores. The spore inactivation achieved in this study by using the newly-developed Al-based composites was primarily attributed to chemical stresses as the thermal effect could not solely produce the observed high IF values. The findings point to a possible synergy of the thermal and chemical spore inactivation mechanisms.
It is acknowledged that this laboratory investigation was designed with challenge bioaerosols represented by single spores or small aggregates while a real-world scenario could involve larger particles (large agglomerates or nonbiological spore carriers). The latter may be harder to inactivate due to the shielding effect. The present investigation was limited to comparing the biocidal properties of different newly-developed materials and did not set any “target” level of inactivation. Additional research efforts should be undertaken to assess the spore inactivation in presence of larger aerosol particles and ultimately investigate the particle size effect on the spore inactivation.
Funding
This study was funded by the Defense Threat Reduction Agency (DTRA, US Department of Defense) through Grants HDTRA1-11-1-0017 and HDTRA1-15-1-0024. The authors are grateful for this financial support.
References
- Abraham, A., Obamedo, J., Schoenitz, M., and Dreizin, E. L. (2015). Effect of Composition on Properties of Reactive Al·B·I2 Powders Prepared by Mechanical Milling. J. Phys. Chem. Solids, 83:1–7.
- Abraham, A., Schoenitz, M., and Dreizin, E. L. (2013). Metal-based Reactive Materials with Biocidal Reaction Products. Proceedings of 2013 AIChE Annual Meeting: Global Challenges for Engineering a Sustainable Future. San Francisco, Nov. 3–8, pp. 441–442.
- Abraham, A., Zhang, S., Aly, Y., Schoenitz, M., and Dreizin, E. L. (2014). Aluminum-Iodoform Composite Reactive Material. Adv. Eng. Mater., 16 (7):909–917.
- Adhikari, A., Yermakov, M., Indugula, R., Reponen, T., Driks, A., and Grinshpun, S. A. (2016). Culturability of Bacillus Spores on Aerosol Collection Filters Exposed to Airborne Combustion Products of Al, Mg, and B·Ti. Environ. Res., 147:212–217.
- Aly, Y., Zhang, S., Schoenitz, M., Hoffmann, V. K., Dreizin, E. L., Yermakov, M., Indugula, R., and Grinshpun, S. A. (2014). Iodine-Containing Aluminum-based Fuels for Inactivation of Bioaerosols. Combust. Flame, 161 (1):303–310.
- Beuchat, L. R., Pettigrew, C. A., Tremblay, M. E., Roselle, B. J., and Scouten, A. J. (2005). Lethality of Chlorine, Chlorine Dioxide, and a Commercial Fruit and Vegetable Sanitizer to Vegetative Cells and Spores of Bacillus Cereus and Spores of Bacillus Thuringiensis. J. Ind. Microbiology Biotechnol., 32 (7):301–308.
- Caldicott, D. G. E., and Edwards, N. A. (2002). The Tools of the Trade: Weapons of Mass Destruction. Emergency Med., 14 (3):240–248.
- Clark, B. R., and Pantoya, M. L. (2010). The aluminium and iodine pentoxide reaction for the destruction of spore forming bacteria. Phys. Chem. Chemical Phys., 12 (39):12653–12657.
- Corcoran, A. L., Wang, S., Aly, Y., and Dreizin, E. L. (2015). Combustion of Mechanically Alloyed Al·Mg Powders in Products of a Hydrocarbon Flame. Combust. Sci. Technol., 187 (5):807–825.
- Corcoran, A. L., Hoffmann, V. K., and Dreizin, E. L. (2013a). Aluminum Particle Combustion in Turbulent Flames. Combust. Flame, 160 (3):718–724.
- Corcoran, A., Mercati, S., Nie, H., Milani, M., Montorsi, L., and Dreizin, E. L. (2013b). Combustion of Fine Aluminum and Magnesium Powders in Water. Combust. Flame, 160 (10):155–160.
- D'Amelio, E., Gentile, B., Lista, F., and D'Amelio, R. (2015). Historical Evolution of Human Anthrax from Occupational Disease to Potentially Global Threat as Bioweapon. Environ. Int., 85:133–146.
- Faille, C., Dennin, L., Bellon-Fontaine, M. N., and Benezech, T. (1999). Cleanability of Stainless Steel Surfaces Soiled by Bacillus Thuringiensis Spores under various Flow Conditions. Biofouling, 14 (2):143–151.
- Farley, C., and Pantoya, M. (2010). Reaction Kinetics of Nanometric Aluminum and Iodine Pentoxide. J. Therm. Anal. Calorimetry, 102 (2):609–613.
- Feng, J., Jian, G., Liu, Q., and Zachariah, M. R. (2013). Passivated Iodine Pentoxide Oxidizer for Potential Biocidal Nanoenergetic Applications. ACS Appl. Mater. Interfaces, 5 (18):8875–8880.
- Gibbons, H. S., Broomall, S. M., McNew, L. A., Daligault, H., Chapman, C., Bruce, D., Karavis, M., Krepps, M., McGregor, P. A., Hong, C., Park, K. H., Akmal, A., Feldman, A., Lin, J. S., Chang, W. E., Higgs, B. W., Demirev, P., Lindquist, J., Liem, A., Fochler, E., Read, T. D., Tapia, R., Johnson, S., Bishop-Lilly, K. A., Detter, C., Han, C., Sozhamannan, S., Rosenzweig, C. N., and Skowronski, E. W. (2011). Genomic Signatures of Strain Selection and Enhancement in Bacillus Atrophaeusvar. Globigii, a Historical Biowarfare Simulant. PLoS ONE, 6 (3):4. doi: 10.1186/2041-2223-1-4.
- Greenberg, D. L., Busch, J. D., Keim, P., and Wagner, D. M. (2010). Identifying Experimental Surrogates for Bacillus Anthracis Spores: A Review. Investigative Genetics, 1 (1): e17836. doi: 10.1371/journal.pone.0017836.
- Grinshpun, S. A., Adhikari, A., Li, C., Reponen, T., Yermakov, M., Schoenitz, M., Dreizin, E., Trunov, M., and Mohan, S. (2010a). Thermal Inactivation of Airborne Viable Bacillus Subtilis Spores by Short-Term Exposure in Axially Heated Air Flow. J. Aerosol Sci., 41 (4):352–363.
- Grinshpun, S. A., Adhikari, A., Yermakov, M., Reponen, T., Dreizin, E., Schoenitz, M., Hoffmann, V., and Zhang, S. (2012). Inactivation of Aerosolized Bacillus Atrophaeus(BG) Endospores and MS2 Viruses by Combustion of Reactive Materials. Environ. Sci. Technol., 46 (13):7334–7341.
- Grinshpun, S. A., Li, C., Adhikari, A., Yermakov, M., Reponen, T., Schoenitz, M., Dreizin, E., Hoffmann, V., and Trunov, M. (2010b). Method for Studying Survival of Airborne viable Microorganisms in Combustion Environments: Development and Evaluation. Aerosol Air Qual. Res., 10 (5):414–424.
- Helfinstine, S. L., Vargas-Aburto, C., Uribe, R. M., and Woolverton, C. J. (2005). Inactivation of Bacillus Endospores in Envelopes by Electron Beam Irradiation. Appl. Environ. Microbiology, 71 (11):7029–7032.
- Henderson, J., Longbottom, A. W., Milne, A. M., Lightstone, J. M., Milby, C., Stamatis, D., Svingala, F. R., Daniels, A. L., Bensman, M., Bohmke, M., and Miller, K. (2015). Experiments and Modeling for Biocidal Effects of Explosives. Propellants, Explosives, Pyrotechnics, 40 (5):712–719.
- Kerwat, K., Becker, S., Wulf, H., and Densow, D. (2010). Biological Weapons. Deutsche Med. Wochenschrift, 135 (33):1612–1616.
- Luna, V. A., Cannons, A. C., Amuso, P. T., and Cattani, J. (2008). The Inactivation and Removal of Airborne Bacillus Atrophaeus Endospores from Air Circulation Systems using UVC and HEPA Filters. J. Appl. Microbiology, 104 (2):489–498.
- Nelson, R. W. (2003). Nuclear Bunker Busters, Mini-Nukes, and the US Nuclear Stockpile. Phys. Today, 56 (11):32–37.
- Orson, J. A., Bagby, W. F., and Perram, G. P. (2003). Infrared Signatures from Bomb Detonations. Infrared Phys. Technol., 44 (2):101–107.
- Rice, E. W., Adcock, N. J., Sivaganesan, M., and Rose, L. J. (2005). Inactivation of Spores of Bacillus Anthracis Sterne, Bacillus Cereus, and Bacillus Thuringiensis Subsp. Israelensisby Chlorination. Appl. Environ. Microbiol., 71 (9):5587–5589.
- Shoshin, Y. L., Trunov, M. A., Zhu, X., Schoenitz, M., and Dreizin, E. L. (2006). Ignition of Aluminum-rich Al-Ti Mechanical Alloys in Air. Combust. Flame, 144 (4):688–697.
- Sinclair, R. G., Rose, J. B., Hashsham, S. A., Gerba, C. P., and Haase, C. N. (2012). Criteria for Selection of Surrogates used to Study the Sate and Control of Pathogens in the Environment. Appl. Environ. Microbiol., 78 (6):1969–1977.
- Sullivan, K. T., Piekiel, N. W., Chowdhury, S., Wu, C., Zachariah, M. R., and Johnson, C. E. (2011). Ignition and Combustion Characteristics of Nanoscale Al/AgIO 3: A Potential Energetic Biocidal System. Combust. Sci. Technol., 183 (3):285–302.
- Sullivan, K. T., Wu, C., Piekiel, N. W., Gaskell, K., and Zachariah, M. R. (2013). Synthesis and Reactivity of Nano-Ag2O as an Oxidizer for Energetic Systems Yielding Antimicrobial Products. Combust. Flame, 160 (2):438–446.
- Tufts, J. A. M., Calfee, M. W., Lee, S. D., and Ryan, S. P. (2014). Bacillus Thuringiensis as a Surrogate for Bacillus Anthracis in Aerosol Research. World J. Microbiology Biotechnol., 30 (5):1453–1461.
- Wang, S., Abraham, A., Zhong, Z., Schoenitz, M., and Dreizin, E. L. (2016a). Ignition and Combustion of Boron-based Al·B·I2 and Mg·B·I2 Composites. Chemical Eng. J., 293:112–117.
- Wang, S., Corcoran, A. L., and Dreizin, E. L. (2015). Combustion of Magnesium Powders in Products of an Air/Acetylene Flame. Combust. Flame, 162 (4):1316–1325.
- Wang, S., Mohan, S., and Dreizin, E. L. (2016b). Effect of Flow Conditions on Burn Rates of Metal Particles. Combust Flame, 168:10–19.
- Ward, T. S., Trunov, M. A., Schoenitz, M., and Dreizin, E. L. (2006). Experimental Methodology and Heat Transfer Model for Identification of Ignition Kinetics of Powdered Fuels. Int. J. Heat Mass Transfer, 49 (25-26):4943–4954.
- Zhang, S., Badiola, C., Schoenitz, M., and Dreizin, E. L. (2012). Oxidation, Ignition, and Combustion of Al·I 2 Composite Powders. Combust. Flame, 159 (5):1980–1986.
- Zhang, S., Schoenitz, M., and Dreizin, E. L. (2010a). Iodine Release, Oxidation, and Ignition of Mechanically Alloyed Al-I Composites. J. Phys. Chem. C, 114 (46):19653–19659.
- Zhang, S., Schoenitz, M., and Dreizin, E. L. (2010b). Mechanically Alloyed Al-I Composite Materials. J. Phys. Chem. Solids, 71 (9):1213–1220.
- Zhou, W., DeLisio, J. B., Li, X., Liu, L., and Zachariah, M. R. (2015). Persulfate Salt as an Oxidizer for Biocidal Energetic Nano-Thermites. J. Mater. Chem. A, 3 (22):11838–11846.