ABSTRACT
The critical velocity of rebound was determined for spherical ammonium fluorescein particles in the size range of 0.44–7.3 μm. The method was based on measurements with a variable nozzle area impactor (VNAI) and numerical simulations. A comparison to previous results with spherical silver particles obtained with the same method showed that the critical velocity was approximately two orders of magnitude higher for ammonium fluorescein than for silver at the same size range. Among the hard test materials, including steel, aluminium, molybdenum, and Tedlar, the surface material had no significant effect on the critical velocity of rebound within the accuracy of the method. On the contrary, the critical velocity was observed to be highly dependent on the obliquity of the impact at the onset of rebound. While the ratio of the maximum tangential and normal velocities was defined as a measure for the obliquity, the critical velocity was found to be more than a magnitude smaller for very oblique impacts with the velocity ratio above 9 than for close-to-normal impacts with the velocity ratio below 1.5. The results of this study can be considered as a link between the recently published critical velocity results for nanoparticles and the older results for micron-sized particles.
Copyright © 2017 American Association for Aerosol Research
EDITOR:
1. Introduction
Particle rebound from a surface is of interest in many fields of aerosol science. It has been known for a long time as an unwanted phenomenon causing artifacts in inertial impactors (Dzubay et al. Citation1976; Chang et al. Citation1999) and triboelectric charging of particles in medical inhalators (Matsusaka et al. Citation2010). Also in aerosol mass spectrometers, the particle rebound has more recently been recognized as a significant source of error and uncertainty (Kang et al. Citation2015). On the other hand, the research of the particle rebound has provided valuable information on some particle properties. The amorphous solid state of secondary organic aerosol particles (SOA) was discovered by investigating the bounce behavior of the particles in a cascade impactor (Virtanen et al. Citation2010). The research has been further developed toward monitoring the particle rebound as a function of the relative humidity in specific low pressure impactor systems (Saukko et al. Citation2012; Bateman et al. Citation2014; Pajunoja et al. Citation2015). In the field of engineered nanoparticles, the particle rebound and fragmentation has been exploited to study the binding energies of agglomerates (Froeschke et al. Citation2003; Seipenbusch et al. Citation2007; Ihalainen et al. Citation2014). Recently, the triboelectric charging during resuspension and rebound was proposed to have a significant effect on the dynamics, sampling, and filtration of indoor aerosols such as fungal spores (Kuuluvainen et al. Citation2016).
The critical velocity of rebound is a fundamental parameter in the interaction of a particle and a surface. It is the smallest incident velocity with which the particle rebounds from the surface. A theory predicting the critical velocities for aerosol particles was formed by Dahneke (Citation1971) and later specified by Wall et al. (Citation1990). The basic idea in the theory is that the sum of the initial kinetic energy and the potential energy of the adhesion force field, reduced by the energy losses during the collision, has to be larger than the adhesion energy for a particle to rebound. According to the theory, the critical velocity is inversely proportional to the particle size and it depends on the material properties of both the particle and the surface. The effect of adhesion in the particle–surface interaction can be described quantitatively with the Hamaker constant and the energy losses with a coefficient of restitution, i.e., the relation of the rebound and incident velocities. The critical velocity of rebound is in principle linked to a normal impact or to the normal component of the incident velocity. However, it has been found that in oblique impacts the capture limit for the normal velocity may be lower than in normal impacts. The experiments with large microspheres have shown that the coefficient of restitution may increase with a decreasing contact angle on rough surfaces, even up to unrealistically high values above unity with contact angles smaller than 10 (Brach et al. Citation2000). Wang and John (Citation1988) concluded that the critical velocity of micron-sized particles determined with an impactor was lower than the critical velocity based on the direct measurement of the velocities and the coefficient of restitution, because of the tangential velocity in the impactor. However, they did not present any quantitative analysis on the effect of the obliquity on the critical velocity. Also a recent modeling work by Chen et al. (Citation2015) predicted a rapidly decreasing critical velocity with an increasing contact angle.
Experimental results for the critical velocity of rebound in the size range above 1 m were reviewed by Wall et al. (Citation1990). They found differences of more than a magnitude between the critical velocities for different particle and surface materials. However, the variety of different experimental methods and the lack of uncertainty estimation may prevent to reveal the actual role of material properties and their effect on the critical velocity. The critical velocity of rebound has also recently been determined in the sub-micron size range. Rennecke and Weber (Citation2013) obtained critical velocity values for silver and sodium chloride nanoparticles using a combination of experimental and numerical methods for inertial impactors. A similar method with a new type of an impactor, the variable nozzle area impactor (VNAI), was exploited to determine the critical velocity of rebound for spherical silver particles in a wide size range from 20 nm to 1
m (Arffman et al. Citation2015). Both of these studies in the sub-micron size range predicted an exponential decay of the critical velocity as a function of the increasing particle size, similar to the results reviewed by Wall et al. (Citation1990). However, if these critical velocity values are extrapolated to the same particle size range, a difference of several orders of magnitude would be seen. Despite the relatively large amount of data, it is still unclear what is the role of different particle and surface materials on the critical velocity. Furthermore, it is not known if the different methods used in the experiments and data analysis agree with the same materials and in the same size range. The experimental data of the critical velocity of rebound have also recently motivated researchers to develop and improve theoretical models (Stace Citation2015; Xie et al. Citation2016).
In this study, we present new results for the critical velocity of rebound for ammonium fluorescein particles, obtained using the recently introduced VNAI and numerical simulations. The main idea of this study is to compare the critical velocity for different particle and surface materials determined with the same method, and, on the other hand, discuss about the differences between the various experimental methods. The goal of this study is to form a link between the recently published critical velocity results for nanoparticles and the older results for micron-sized particles. In addition, we investigate the effect of tangential velocity component on the critical velocity in the impactor.
2. Methods
2.1. Particle generation and size classification
Solid spherical ammonium fluorescein (CH
NO
) particles in the size range of 2–8
m were generated using a vibrating orifice aerosol generator (VOAG) introduced by Berglund and Liu (Citation1973). In the VOAG, a liquid solution is fed into a vibrating orifice generating highly monodisperse droplets. Then the liquid droplets are dispersed and dried in order to generate a monodisperse aerosol of desired nonvolatile material and particle size. The diameter of the liquid droplet can be calculated through an equation depending on the volumetric liquid feed flow and the frequency of the disturbance in the vibrating orifice (Berglund and Liu Citation1973). The final particle diameter is a function of the droplet diameter and the volumetric concentration of a nonvolatile solute in a volatile solvent. In this study, solutions of ammonium fluorescein were prepared by dissolving fluorescein powder (C
H
O
, Alfa Aesar, purity 90
%) in aqueous ammonia (NH
OH) as described by Vanderpool and Rubow (Citation1988). The final concentration of ammonium fluorescein varied from 0.08 to 2.2
and the frequency of the disturbance was 33 or 60 Hz. The feed flow induced by a syringe pump was kept constant (10 ml/h) and the diameter of the orifice was 20
m. The relative humidity (RH) of the dispersion and dilution air was adjusted to 30% in order to prevent too fast drying of the droplets as described by Wall et al. (Citation1990). A schematic of the experimental setup using the VOAG is seen in . The generated particles were neutralized with a radioactive source (Am-241) after the VOAG. The stability of the generator was monitored along with the particle detection as described later.
Figure 1. Schematics of the measurement setups (a) for the particles generated with a vibrating orifice aerosol generator (VOAG) covering the particle size range of 2–8 , and (b) for the particles generated with an atomizer and classified with a differential mobility analyzer (DMA) covering the size range below 1
. MFC is an abbreviation for a mass flow controller, VNAI for a variable nozzle area impactor, and UV-APS for an ultraviolet aerodynamic particle sizer.
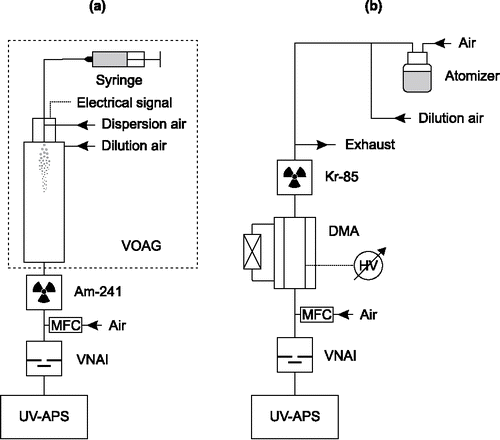
Particles in the size range below 1 m were generated using an atomizer aerosol generator (Topas ATM 220) and an ammonium fluorescein solution with a concentration of 8.8
. After the atomizer, the aerosol was dried with a humidity-controlled dilution air flow (RH 30–32%). The particles were size-classified with a Vienna type differential mobility analyzer (DMA). A schematic of the measurement setup is seen in . Because of multiply charged particles, the output of the DMA was not ideally monodisperse and the multiply charged particles were distinguished with the detection method. A radioactive source (Kr-85) was used before the DMA to create a net neutral equilibrium charge distribution for the aerosol.
2.2. Critical velocity experiment
The measurements were carried out using a variable nozzle area impactor (VNAI). This recently introduced instrument (Arffman et al. Citation2015) is designed to study the critical velocity of rebound and other surface interaction phenomena as a function of the particle impact velocity. It consists of a narrow slit, and the nozzle area can be varied by manually changing the slit length (3–30 mm). A detailed description of the VNAI can be found from the work of Arffman et al. (Citation2015). In this study, some of the dimensions and parameters of the impactor varied between different experiments. The volumetric flow through the impactor varied from 0.7 to 10 lpm, the impactor nozzle width from 0.1 to 2.0 mm, and the jet-to-plate distance from 0.5 to 2.0 mm, depending on the operated particle size range. The impactor flow was adjusted with the help of a mass flow controller (MFC) before the VNAI (). Four different substrate materials, including steel, aluminium, molybdenum, and Tedlar (polyvinyl fluoride), and a substrate greased with Apiezon-L vacuum grease were used in the impactor. The metal substrates were equally manufactured and finally polished. The Tedlar substrate was compiled from a steel substrate that was covered with a layer of commercial adhesive tape with a Tedlar backing (3M, Weather Resistant Film Tape 838). Before the experiments, the non-greased substrates were flushed with isopropanol, distilled water, and clean pressurized air, respectively.
The collection efficiency of the VNAI was determined as a function of the slit length by measuring the particle concentration after the impactor and dividing it by a reference. As a primary reference, the concentration was measured after the impactor with the slit fully open. The secondary reference was the concentration measured by bypassing the impactor, but this was only used to ensure that the collection efficiency of the impactor was insignificant with the slit fully open. Different detection methods were used to measure the particle concentration, depending on the operated particle size range. For the particles generated with the VOAG, the concentration was measured with an ultraviolet aerodynamic particle sizer (UV-APS, Model 3314, TSI Inc., Shoreview, MN, USA) using the total concentration given by the instrument. In the size range of 0.47–1.0 m, the concentration was also measured with the UV-APS, but only a single channel from the size distribution, corresponding to the singly charged particle size penetrated through the DMA, was taken into account. In both cases, the UV-APS was used without the ultraviolet laser and fluorescence measurement, operating as an aerodynamic particle sizer.
An overview of the conducted experiments can be seen in . Altogether, nine different particle sizes were measured, two different particle generation methods were used and the concentration was measured using two different detection methods. The particle sizes for the VOAG generated particles were calculated and the particle sizes classified with the DMA were determined from the voltage and sheath flow. also includes the impactor nozzle width and impactor flow that are crucial input parameters for the numerical simulation and the determination of the impact velocities. Each particle size was measured with a greased impactor substrate and with four different non-greased substrates. An experiment for one particle size with a single substrate included the measurement of the collection efficiency of the VNAI as a function of the slit length. The concentration was measured from 30 to 60 s for a single slit length in order to minimize the effect of random noise to the results. The reference concentration measured with the slit fully open was taken between every fourth or fifth slit lengths. The substrate loading caused by the collected particles was calculated to be less than 5%.
Table 1. Experimental parameters for each particle size. VOAG refers to a vibrating orifice aerosol generator. Either (1) the concentration of a single size distribution channel or (2) the total concentration of an ultraviolet aerodynamic particle sizer (UV-APS) was used in the detection. The impactor nozzle width, jet-to-plate distance and flow are the parameters of a variable nozzle area impactor (VNAI).
2.3. Numerical simulation
The impact velocities were determined by using computational fluid dynamics (CFD) simulation. A detailed description and the principles of the method can be found in previous studies (Arffman et al. Citation2011, Citation2015). Briefly, the simulations were conducted in two steps. The flow field was first simulated (2D) with ANSYS Fluent software by using the mass flow rate through the impactor and the static pressure at the outlet as boundary conditions. As the Reynolds’ numbers were in the order of 500, the flow field could be assumed to be laminar. The changes of the slit length and the nozzle area were taken into account in the simulation by changing the mass flow rate per depth of the slit length. In the second step, the particle trajectories were solved using a Lagrangian particle tracking method. By solving the trajectories, we were able to determine the collection efficiencies and impact velocities of the particles. The computation grid independence of the results was confirmed by adapting the grid until the results remained unaffected by further refinements. The grid had to be more dense near the collection substrate, where the resolution of the grid was below 1 m.
Experimental and simulated collection efficiency curves were fitted by superimposing the cutpoints of the two curves. The outcome from the fit was the effective density parameter obtained for each measurement with greased substrates. The effective density parameter was not only an estimate for the particle density, but it could be used to validate the simulation method as described by Arffman et al. (Citation2015). Several non-idealities, such as the uncertainties in the measured flow rates and pressures, could slightly affect the value of the effective density parameter. However, as the density of the particle material was known a priori, a comparison of the effective density values to the material density could be used as a validation for the simulation.
Two parameters obtained from the measurement, corresponding to the onset of rebound, were used for the simulation to determine the critical velocity of rebound. They were the critical slit length and the critical collection efficiency, for which the experimental determination is presented later. Because the simulated collection efficiency curves were sharper compared to measured ones, the impact velocities had to be determined using a parameterization when the collection efficiency was below 50%. At this region, the critical collection efficiency was used to determine the corresponding slit length on the simulated collection efficiency curve. Above 50%, the critical slit length obtained from the measured curve was directly used for the simulation. This procedure and the parameterization was described in detail by Arffman et al. (Citation2015).
3. Results and discussion
3.1. Morphology, density, and collection efficiency
In order to have a fundamental approach on the critical velocity of rebound for ammonium fluorescein particles corresponding to previous studies (Wang and John Citation1988; Wall et al. Citation1990), we wanted to measure dense and spherical particles. and show two examples of the scanning electron microscope (SEM) micrographs of the generated ammonium fluorescein particles. A clear spherical morphology can be seen in both of these micrographs, despite of the variation of the generation method and the particle size. The 2.5 m particles generated with the VOAG () seemed to have a slightly apple-like morphology with a pit on top of the particles. However, the projection of the particles was spherical with very smooth surface and dense structure. Among the 0.47
m particles generated with the atomizer and size-classified with a DMA (), also larger doubly charged particles, penetrated through the DMA but not detected for the collection efficiency, can be seen. The morphology seemed to be very spherical in a wide particle size range with this generation method.
Figure 2. (a–b) Scanning electron microscope (SEM) micrographs of the ammonium fluorescein particles and (c) the effective density parameter obtained from the fitting process as a function of the particle size. (a) 0.47 particles were generated with an atomizer and size-classified with a differential mobility analyzer (DMA). Also doubly charged larger particles penetrated through the DMA can be seen. Monodisperse (b) 2.5
particles were generated with a vibrating orifice aerosol generator (VOAG). (c) The solid line represents the bulk density of ammonium fluorescein.
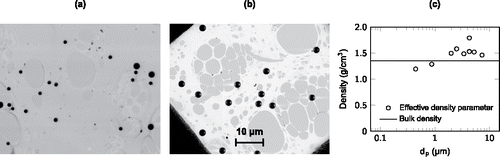
The effective density values obtained from the fitting process of the model and the experiment are seen in . The values were close to the bulk density of ammonium fluorescein that is 1.35 according to Stöber and Flachsbart (Citation1973). This verified the validity of the used simulation methods because the use of the effective densities close to the bulk density provided simulated collection efficiencies corresponding to the measured collection efficiencies. Therefore, also the impact velocities provided by the simulation could be assumed to be realistic. A similar validation for the same method was carried out by Arffman et al. (Citation2015) with spherical silver particles.
shows two examples of the collection efficiency of the VNAI as a function of the slit length. Both of these examples were measured using a greased substrate and a non-greased steel substrate in the impactor. In every case, the collection efficiency was approximately zero at the slit lengths close to the maximum. When the slit length was shortened, the collection efficiency started to increase, reaching approximately one with the greased substrate. With the non-greased substrate, the collection efficiency differed from the curve measured with the greased substrate at some point, indicating the onset of rebound. The slit length and the collection efficiency corresponding to the onset of rebound were the critical slit length and the critical collection efficiency
, respectively. The reincrease of the collection efficiency seen in with slit lengths below 20 mm most probably referred to the onset of plastic deformation with higher impact velocities. Similar behavior was also observed by Arffman et al. (Citation2015) for silver particles.
Figure 3. (a–b) Two examples of the collection efficiency of a variable nozzle area impactor (VNAI) as a function of the slit length. In these examples, the non-greased substrate was steel. The onset of rebound was determined from (c–d) the difference of the non-greased collection efficiency and the fitted curve. The critical slit length and the critical collection efficiency
correspond to the onset of rebound.
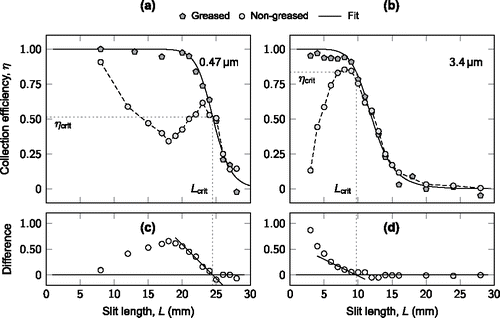
3.2. Critical velocity of rebound and obliquity of impacts
In an impactor, particles can impact with different normal and tangential velocities as shown in the schematic in . At the onset of rebound, some of the impacted particles have a normal velocity that exceeds the critical velocity and they rebound. According to previous studies, the presence of the tangential velocity component may lower the critical normal velocity required for the rebound (Wang and John Citation1988; Brach et al. Citation2000). This may lead to a situation where the rebound begins at a certain location on the impactor substrate from the particles having a lower normal velocity and higher tangential velocity compared to the particles impacting with the maximum normal velocity. It is not possible to distinguish the location of the onset of rebound and the exact velocity components involved. The maximum normal velocity forms an upper limit for the critical velocity of rebound and the lower limit is the normal velocity component from the particles with the maximum tangential velocity. For the experiments of this work, the lower limit was calculated to be close to the upper limit. Even in the worst case, it was approximately 40% of the upper limit. We could have chosen any estimate between these limits to represent the critical velocity, but to be comparable also with the previous studies (Wang and John Citation1988; Rennecke and Weber Citation2013; Arffman et al. Citation2015), we used the maximum normal velocity . Furthermore, the maximum tangential velocity
was used to describe the obliquity of the impact. These values, as well as the jet velocity
, were obtained from the simulation.
Figure 4. (a) A schematic of the particles impacting with different normal and tangential velocities. Bold arrows represent the maximum values of these components. (b) The velocities corresponding to the onset of rebound as a function of the particle size for ammonium fluorescein particles. The maximum value of the normal velocity component and the jet velocity
were determined using simulations. Also the theoretical size dependency of the critical velocity of rebound
is shown. (c) The maximum normal velocity normalized with the theoretical critical velocity as a function of the ratio of the maximum tangential
and normal velocities. The ratio of the maximum tangential and normal velocities corresponds to the obliquity of the impact. Three different groups of data can be seen.
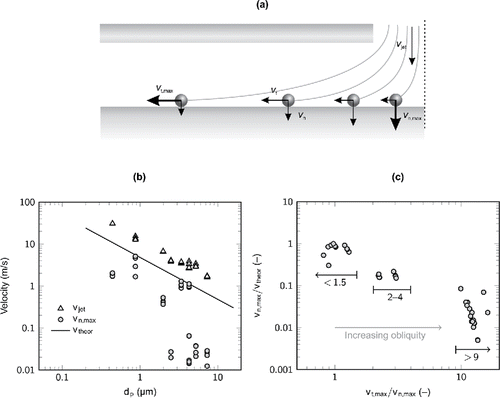
shows the maximum normal velocity and the jet velocity corresponding to the onset of rebound as a function of the particle size. It should be noted that the jet velocity was only one factor describing the impactor flow field at the onset of rebound. Also the flow through the impactor and the nozzle width had been changed between different measurements. A lot of deviation can be seen in the maximum normal velocities compared to the theoretical particle size dependency shown in . The theoretical critical velocity shown in is inversely proportional to the particle size and it was fitted to form an upper limit for the experimental values. The explanation for the deviation of the experimental values was found in the variation of the impactor flow field and the tangential velocity component between different measurements. The ratio of the maximum tangential and normal velocities was defined as a measure of obliquity for the impact. Furthermore, we normalized the maximum normal velocity at the onset of rebound with
in order to have a size-independent but arbitrary quantity for the critical velocity. This normalized maximum normal velocity is presented as a function of the ratio of the maximum tangential and normal velocities in . The results showed that the increase of obliquity and the tangential velocity component remarkably decreased the normal velocity required for the rebound. also shows that the data could be divided into three different groups. The first group was formed for the data of close-to-normal impacts with the velocity ratio below 1.5 and the second group for higher obliquity and the velocity ratios between 2 and 4. In the third group, the impacts were extremely oblique with the velocity ratio above 9.
Consequently, the results of the critical velocity corresponding the maximum normal velocity are shown in divided into the three groups. The obliquity seemed to affect significantly the critical velocity of rebound. Based on the fits with the same theoretical size dependency, the data for velocity ratios of 2–4 differed from the data of the close-to-normal impacts (ratio <1.5) by a factor of 4. The critical velocity values for the most oblique impacts with a velocity ratio above 9 were more than a magnitude lower than the critical velocities for the close-to-normal impacts. The latter data had a significant deviation and uncertainty in the particle size dependency because of the decreasing accuracy of the simulation with extremely low velocities. These results are in line with the previous observations (Wang and John Citation1988; Brach et al. Citation2000; Chen et al. Citation2015). Based on our results, it is possible to estimate quantitatively the effect of obliquity on the critical velocity in impactors.
Figure 5. The critical velocity of rebound as a function of the particle size for ammonium fluorescein particles with respect to the obliquity of the impact. Three different groups having different ratios of the maximum tangential and normal velocities are distinguished. Also the theoretical fits are shown, separately for different groups.
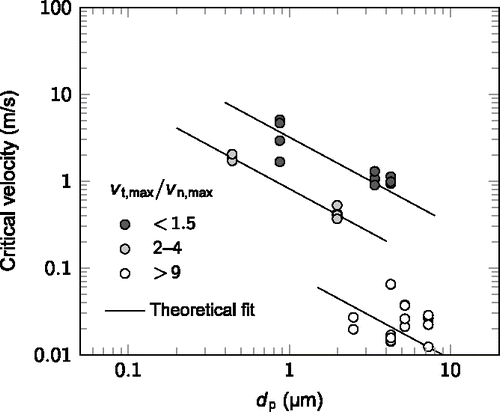
3.3. Effect of different particle and surface materials
Based on the observation of the critical velocity of rebound and the obliquity of impacts, we use the data of the close-to-normal impacts for a detailed analysis and comparison to previous studies. shows this critical velocity as a function of the particle size with respect to the previous results for silver particles (Arffman et al. Citation2015) and for ammonium fluorescein particles (Wang and John Citation1988; Wall et al. Citation1990). We concentrate on three issues, which are the effect of the particle material, the effect of the surface material, and different methods in determining the critical velocity of rebound.
Figure 6. The critical velocity of rebound as a function of the particle size. The results of this study for ammonium fluorescein (CH
NO
) are shown with respect to the previous results for silver particles (Arffman et al. Citation2015) and for ammonium fluorescein particles (Wang and John Citation1988; Wall et al. Citation1990). The marker shape represents the surface material. Power fits for steel (solid lines) and aluminium (dashed lines) are shown. The error bars, shown as an example for steel, represent the sensitivity of the method.
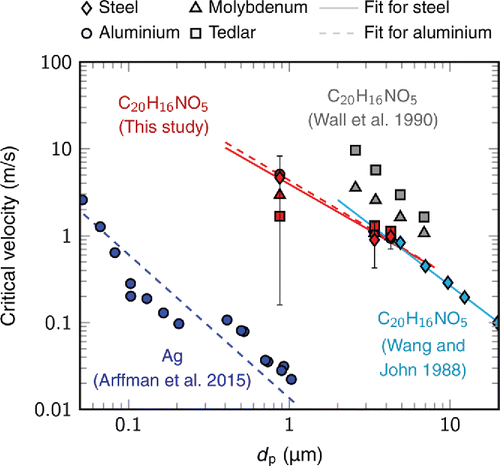
To be sure that the results of Arffman et al. (Citation2015) were comparable to the results of this work, the old results were resimulated and the impacts were found to be close-to-normal with a velocity ratio below 2. The effect of the particle material on the critical velocity seemed to be significant. Arffman et al. (Citation2015) used an aluminium surface for silver particles, but the difference to the results of this study for ammonium fluorescein particles with the same surface material and the same method was substantial (). At the particle size of one micron, the difference in the critical velocity was approximately two orders of magnitude. There was also a difference in the particle size dependence of these two particle materials. A power fit for the silver data resulted in an exponent value of 1.6 and for the ammonium fluorescein data an exponent value of
1.1. However, the value for ammonium fluorescein may not be as reliable as for silver because of a smaller amount of data, more restricted size range and uncertainty related to some of the data points. The error bars for our results describing the sensitivity of the method were estimated by assuming an uncertainty of
1 mm in the measured critical slit length (). The particle sizes of 3.4 and 4.3
m were the most reliable data points in this respect. It should be noted that the error bars only formed an estimation for the accuracy of the method, and also a different estimation could have been used, for example, by assuming a smaller uncertainty in the measured critical slit length. However, with the current error limits, we were able to estimate the relative reliability of different data points and the overall accuracy of the method.
A good agreement was achieved between the results of this study for ammonium fluorescein particles on a steel surface and the results of Wang and John (Citation1988) with the same materials (). The agreement was especially good with the most reliable data points of our results. Wang and John (Citation1988) also used an impactor and computational methods to determine the critical velocity. Comparing the results of this study for molybdenum and Tedlar surfaces to the results of Wall et al. (Citation1990) with the same materials showed relatively small but clear differences (). Wall et al. (Citation1990) used laser Doppler velocimetry to measure the impact and rebound velocity in accurately normal collisions. In an impactor, particles always have some tangential velocity when they impact, which may result in lower critical velocities compared to the laser Doppler velocimetry measurements. This explanation was already raised by Wang and John (Citation1988) and it is in line with our results for increasing obliquity.
The surface material seemed to have no observable effect on the critical velocity of rebound (). It is possible that the effect of the surface material was hidden in the uncertainty of the method and could be seen with a more accurate method. However, no difference was seen between the test materials even for the most reliable data points of our results. Also the power fits shown in for steel and aluminium were almost the same with the exponent value of 1.1. All of the surface materials used in this work were hard and plane on a macroscopic scale, including three metals and one plastic material. Most likely, the results would have been different with softer or porous materials. Even if the effect of obliquity on the critical velocity is linked to the microscopic surface roughness, we did not see any clear differences between the polished metal surfaces and the Tedlar surface. Wall et al. (Citation1990) reported that the adhesion surface energy was an important factor affecting the critical velocity of rebound. However, the effect of the surface energy was small compared to the effect of the particle material and the obliquity of the impact found in this study, as well as, compared to the accuracy of our method. To investigate the effect of the surface material properties on the critical velocity of rebound, measurements for soft and porous materials should be conducted in future and the accuracy of the method should be improved.
4. Summary and conclusions
In this study, the critical velocity of rebound was determined for ammonium fluorescein particles in a size range of 0.44–7.3 m by using a variable nozzle area impactor (VNAI) and numerical simulations. The variation of impactor parameters, primarily used for covering different particle sizes, caused a divergence of the critical velocities. It was found that the obliquity of the impact and the tangential velocity component remarkably lowered the normal velocity required for the rebound, i.e., the critical velocity. The obliquity was characterized quantitatively by the ratio of maximum tangential and normal velocities at the onset of rebound. For velocity ratios of 2–4, the critical velocities were four times lower than the critical velocities for close-to-normal impacts having a velocity ratio smaller than 1.5. The most oblique impacts with a velocity ratio above 9 resulted in critical velocities more than a magnitude smaller compared to the close-to-normal impacts. The strong dependence of the critical velocity on the obliquity of the impact may have an influence on the impactor design and instrument development. Depending on the application, if particle rebound must be avoided or is desired, the impactor should be designed to have high or low tangential velocities, respectively. The effect of obliquity on the critical velocity can be estimated qualitatively in impactors based on the results of this study.
The surface material was found to have no significant effect on the critical velocity of rebound within the accuracy of the method. The test materials in this study were steel, aluminium, molybdenum, and Tedlar. Even though the particle bounce can generally be avoided with greased or porous surface materials in impactors, there were relatively small differences in the rebound sensitivity of these hard test materials. On the other hand, the particle material may have a substantial effect on the critical velocity. This was confirmed by the comparison between the results of this study for ammonium fluorescein particles and the results of Arffman et al. (Citation2015) for silver particles. Both of these results were determined with the same method and the impacts were checked to be close-to-normal with respect to the obliquity. The comparison showed that the critical velocity was approximately two orders of magnitude higher for ammonium fluorescein than for silver at the particle size of one micron.
The results of this study for ammonium fluorescein particles on a steel surface agreed well with the previous results of Wang and John (Citation1988) for the same materials. The agreement practically connects our method based on the VNAI and numerical simulations to the set of previous critical velocity results for micron-sized particles. Since many of the methods applied in the size range above 1 m are not applicable in the nanoparticle size range, this connection is an important link between the nanoparticles and micron-sized particles. We also saw a similar difference between our results and the results of Wall et al. (Citation1990) with the corresponding materials that was seen by Wang and John (Citation1988) in their study. The direct measurement of the incident and rebound velocity in accurately normal collisions, such as performed by Wall et al. (Citation1990), may result in a higher critical velocity than the critical velocity determined using an impactor with close-to-normal impacts. However, this difference is relatively small compared to the effect of the particle material and very oblique impacts outlined above.
Acknowledgments
The authors thank Dr. Mari Honkanen at the Department of Materials Science (TUT) for the scanning electron microscopy images.
Funding
Heino Kuuluvainen acknowledges the TUT's graduate school for financial support.
References
- Arffman, A., Kuuluvainen, H., Harra, J., Vuorinen, O., Juuti, P., Yli-Ojanperä, J., Mäkelä, J., and Keskinen, J. (2015). The Critical Velocity of Rebound Determined for Sub-Micron Silver Particles with a Variable Nozzle Area Impactor. J. Aerosol Sci., 86:32–43.
- Arffman, A., Marjamäki, M., and Keskinen, J. (2011). Simulation of Low Pressure Impactor Collection Efficiency Curves. J. Aerosol Sci., 42(5):329–340.
- Bateman, A., Belassein, H., and Martin, S. (2014). Impactor Apparatus for the Study of Particle Rebound: Relative Humidity and Capillary Forces. Aerosol Sci. Technol., 48(1):42–52.
- Berglund, R., and Liu, B. (1973). Generation of Monodisperse Aerosol Standards. Environ. Sci. Technol., 7(2):147–153.
- Brach, R., Dunn, P., and Li, X. (2000). Experiments and Engineering Models of Microparticle Impact and Deposition. J. Adhesion, 74(1–4):227–282.
- Chang, M., Seongheon, K., and Sioutas, C. (1999). Experimental Studies on Particle Impaction and Bounce: Effects of Substrate Design and Material. Atmos. Environ., 33(15):2313–2322.
- Chen, S., Li, S., and Yang, M. (2015). Sticking/Rebound Criterion for Collisions of Small Adhesive Particles: Effects of Impact Parameter and Particle Size. Powder Technol., 274:431–440.
- Dahneke, B. (1971). The Capture of Aerosol Particles by Surfaces. J. Colloid Interf. Sci., 37(2):342–353.
- Dzubay, T. G., Hines, L. E., and Stevens, R. K. (1976). Particle Bounce Errors in Cascade Impactors. Atmos. Environ., 10(3):229–234.
- Froeschke, S., Kohler, S., Weber, A. P., and Kasper, G. (2003). Impact Fragmentation of Nanoparticle Agglomerates. J. Aerosol Sci., 34(3):275–287.
- Ihalainen, M., Lind, T., Arffman, A., Torvela, T., and Jokiniemi, J. (2014). Break-Up and Bounce of TiO2 Agglomerates by Impaction. Aerosol Sci. Technol., 48(1):31–41.
- Kang, M., Cho, H.-J., Kwak, H., and Park, K. (2015). Evaluation of Particle Bounce in Various Collection Substrates to be used as Vaporizer in Aerosol Mass Spectrometer. Aerosol Sci. Technol., 49(5):332–339.
- Kuuluvainen, H., Saari, S., Mensah-Attipoe, J., Arffman, A., Pasanen, P., Reponen, T., and Keskinen, J. (2016). Triboelectric Charging of Fungal Spores During Resuspension and Rebound. Aerosol Sci. Technol., 50(2):187–197.
- Matsusaka, S., Maruyama, H., Matsuyama, T., and Ghadiri, M. (2010). Triboelectric Charging of Powders: A Review. Chem. Eng. Sci., 65(22):5781–5807.
- Pajunoja, A., Lambe, A., Hakala, J., Rastak, N., Cummings, M., Brogan, J., Hao, L., Paramonov, M., Hong, J., Prisle, N., Malila, J., Romakkaniemi, S., Lehtinen, K., Laaksonen, A., Kulmala, M., Massoli, P., Onasch, T., Donahue, N., Riipinen, I., Davidovits, P., Worsnop, D., Petäjä, T., and Virtanen, A. (2015). Adsorptive Uptake of Water by Semisolid Secondary Organic Aerosols. Geophys. Res. Lett., 42(8):3063–3068.
- Rennecke, S., and Weber, A. P. (2013). The Critical Velocity for Nanoparticle Rebound Measured in a Low Pressure Impactor. J. Aerosol Sci., 58:135–147.
- Saukko, E., Kuuluvainen, H., and Virtanen, A. (2012). A Method to Resolve the Phase State of Aerosol Particles. Atmos. Meas. Technique, 5(1):259–265.
- Seipenbusch, M., Toneva, P., Peukert, W., and Weber, A. P. (2007). Impact Fragmentation of Metal Nanoparticle Agglomerates. Part. Part. Sys. Character., 24(3):193–200.
- Stace, A. (2015). Molecular Dynamics Study of the Surface Scattering and Capture of Nanoparticles at High Velocities. J. Aerosol Sci., 83:32–38.
- Stöber, W., and Flachsbart, H. (1973). An Evaluation of Nebulized Ammonium Fluorescein as a Laboratory Aerosol. Atmos. Environ., 7(7):741–748.
- Vanderpool, R., and Rubow, K. (1988). Generation of Large, Solid, Monodisperse Calibration Aerosols. Aerosol Sci. Technol., 9(1):65–69.
- Virtanen, A., Joutsensaari, J., Koop, T., Kannosto, J., Yli-Pirilä, P., Leskinen, J., Mäkelä, J. M., Holopainen, J. K., Pöschl, U., Kulmala, M., Worsnop, D. R., and Laaksonen, A. (2010). An Amorphous Solid State of Biogenic Secondary Organic Aerosol Particles. Nature, 467(7317):824–827.
- Wall, S., John, W., and Wang, H.-C. (1990). Measurements of Kinetic Energy Loss for Particles Impacting Surfaces. Aerosol Sci. Technol., 12(4):926–946.
- Wang, H.-C., and John, W. (1988). Dynamic Adhesion of Particles Impacting a Cylinder, in Particles on Surfaces 1: Detection, Adhesion, and Removal, K. L. Mittal, ed., Plenum Press, New York, pp. 211–224.
- Xie, J., Dong, M., and Li, S. (2016). Dynamic Impact Model of Plastic Deformation between Microparticles and Flat Surfaces Without Adhesion. Aerosol Sci. Technol., 50(4):321–330.