ABSTRACT
Composite nanoparticles find application in catalysis, drug delivery, and energy storage and require increasingly fine control of their physical properties and composition. While composite nanoparticles have been widely synthesized and characterized, little work has systematically correlated the initial concentration of precursors and the final composition of flame synthesized composite nanoparticles. This relationship is explored in a diffusion flame aerosol reactor by coupling a scanning mobility particle sizer (SMPS) with an inductively coupled plasma optical emission spectrometer (ICP-OES). A framework for studying the relationship between the initial precursor concentrations of different elements and the final nanoparticle composition is explored. The size-resolved elemental composition was measured by directly injecting size-selected fractions of aggregated magnetite and silicon dioxide composite nanoparticles into the ICP-OES plasma. This work showed a correlation between precursor molar ratio and the measured elemental ratio in the mobility size range of 50 to 140 nm. Building on previous work studying size resolved elemental composition of engineered nanoparticles, the analysis is extended to flame synthesized composite nanoparticle aggregates in this work.
Copyright © 2017 American Association for Aerosol Research
EDITOR:
Introduction
Due to the potential social and economic impacts of engineered nanomaterials, extensive research has characterized their physical and chemical properties over a wide range of sizes, morphologies, and chemical compositions (Li et al. Citation2016). Annually, flame aerosol reactors (FLAR) produce an estimated 15 billion dollars' worth of engineered nanoparticles (Wegner and Pratsinis Citation2003). Due to its scalability and commercial success, combustion synthesis shows promise for the production of novel nanomaterials for catalysis (Sahu and Biswas Citation2011), water purification (Jiang et al. Citation2014), water splitting (Crawford et al. Citation2009), solar energy production (Tiwari et al. Citation2008; Wang et al. Citation2011), and nanomedicine (Gupta and Gupta Citation2005). While significant research into these materials has been reported, the formation mechanisms of these composite particles and their aggregates have not been fully elucidated by real time size-resolved elemental analysis (Ehrman et al. Citation1998; Worathanakul et al. Citation2008; Harra et al. Citation2013).
While aerosol instrumentation has provided improved characterization ability over the last 40 years, there is still a need for real-time measurement of size-resolved elemental composition. An inductively coupled optical emission spectrometer (ICP-OES) was first used for size-resolved elemental analysis of copper aerosols (Kawaguchi et al. Citation1986). While detection limits were initially a constraint, this methodology was adopted for analysis of manganese and calcium-based aerosol particles (Bochert and Dannecker Citation1989, Citation1992; Nomizu et al. Citation1992). Building on this technique, other works coupled a differential mobility analyzer (DMA) with the ICP-OES to better characterize the size distribution of silver particle aerosols and their agglomeration (Weber et al. Citation1991, Citation1992). Similar work coupled a DMA to an inductively coupled plasma mass spectrometer (ICP-MS) to measure lead and tin aerosols (Myojo et al. Citation2002; Okada et al. Citation2002). Recent works investigating size-resolved elemental analysis have coupled scanning mobility particle sizer (SMPS) with ICP-MS using a rotating disk diluter (RDD) to draw particles from the DMA of the SMPS to the inlet of the ICP-MS (Hess et al. Citation2015; Losert et al. Citation2015). These works report the use of this hyphenated system to analyze airborne silver particles and commercially available spray products containing many trace elements (Hess et al. Citation2015; Losert et al. Citation2015). Extending this approach, the coupling of an SMPS with an ICP-OES using a dilution probe in an effort to better relate Si to Fe ratio of our flame synthesized composite nanoparticle aggregates to their precursor concentrations was studied. While an ICP-OES has less detection sensitivity than an ICP-MS, the ICP-OES allows for analysis of higher particle concentrations and is less susceptible to contamination.
In this work, the use of a tandem SMPS-ICP-OES to explore multi-component nanoparticle formation in a diffusion flame aerosol reactor was studied. Aerosols downstream of the FLAR were sampled and measured at different precursor molarities of Si and Fe. The effect of precursor concentrations on nanoparticle formation by studying the particles' elemental composition throughout a size range of 50 nm to 140 nm for different Si to Fe precursor ratios was evaluated. Particle composition was measured by directly injecting size-selected nanoparticle agglomerates into the ICP-OES plasma. By scanning through the size distribution, Fe and Si concentration data from the ICP-OES were collected to form the elemental distribution of the agglomerated particles. This work provides a roadmap for studying aerosol synthesized composite nanoparticle formation and aggregation by tracking the elemental composition throughout the growth process.
Experimental
SMPS and ICP-OES coupling
Size distributions were measured using an SMPS (Model 3080, TSI Inc., Shoreview, MN, USA). The condensation particle counter (CPC, TSI model 3025A) of the SMPS was then coupled to the ICP-OES utilizing a dilution sampling probe, shown in . By matching the 1.5 lpm flow rate of the CPC to the dilution sampling flow and matching the 2 lpm input flow of the ICP-OES to its nebulizer flow, there was a successful coupling of both systems for real-time measurement of size-resolved elemental composition. The classified, monodispersed aerosol was injected into the ICP-OES, where optical emission intensities from the particles in the argon plasma were measured. Argon was maintained as an operational and dilution gas to sustain the ICP-OES plasma while coupling the SMPS and ICP-OES. Previous works have shown that utilization of argon as an operational gas for the SMPS provides a negligible change in size distribution measurement (Hess et al. Citation2015). Size-resolved elemental composition data were collected by fixing the DMA voltage through the SMPS interface to provide monodisperse aerosols in each of the 10 to 20 size bins selected with the DMA. The resulting ICP-OES intensities for Si and Fe were then used to calculate mass concentration using a calibration curve measured before each run. To create the calibration curve 10, 100, and 1000 μg/mL dispersions of 100 nm magnetite and silicon dioxide nanoparticles were atomized into the ICP-OES. The resulting measured intensities were used to calculate the linear relationship between ICP-OES intensity and Fe and Si mass concentration at the time of each run. This calculated mass concentration was then compared to the size distribution of agglomerate nanoparticles produced for a specific Si to Fe precursor molar ratio.
Figure 1. Schematic diagram of the coupled SMPS-ICP-OES for measurement of size resolved elemental composition. The main components from left to right are the diffusion flame aerosol reactor (FLAR), atomizer, charge neutralizer, differential mobility analyzer (DMA), condensation particle counter (CPC), dilution probe, and ICP-OES.
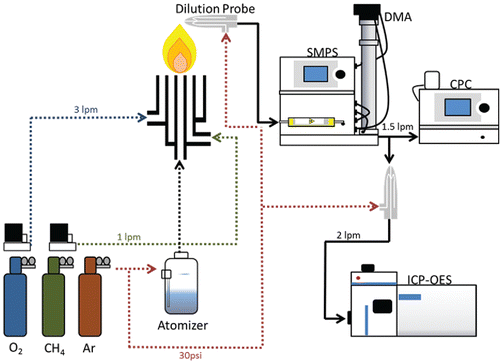
Calculating mass distribution of fractal aggregates
To address the inaccuracies of the mass estimation from the SPMS measured mobility diameter, the mass distribution and surface area distribution were calculated from the raw particle counts of the CPC while accounting for the fractal nature of the nanoparticle aggregates. Liu and Chakrabarty have derived an empirical equation (Equation Equation(1))[1] to relate mobility diameter (dm) to the number of primary particles (N) in each aggregate with a known fractal prefactor (kf), fractal dimension, and diameter of primary particles (d). The number of primary particles can then be used to find surface area and mass of aggregate nanoparticles with a known density (Liu and Chakrabarty Citation2016). For flame synthesized nanoparticle aggregates, it can be assumed they undergo a diffusion limited cluster-cluster aggregation process giving them a fractal dimension of 1.78 and fractal prefactor of 1.3 (Sorensen Citation2011). Primary particle diameter of the aggregate nanoparticles was measured using ImageJ analysis of TEM images of the nanoparticle aggregates and can be found in :
[1]
Table 1. Measured sizes of particles synthesized using diffusion flame aerosol reactor. Geometric mean diameter for number particle size (GMD #), geometric mean diameter for surface particle size (GMD SA), and geometric mean diameter for mass particle size (GMD Mass) were collected using SMPS. Primary particle diameter was measured from TEM images using ImageJ.
Diffusion flame aerosol reactor
The main components of the flame aerosol reactor include a diffusion burner, atomizer, quench ring, and dilution sampler. Design details of the diffusion burner used for this experiment are described by Jiang et al. (Citation2007). Further details on the operational parameters for the FLAR are listed in and the online supplemental information (SI). Solutions of tetraethyl orthosilicate and iron (III) acetylacetonate precursors in toluene were prepared at 1:4, 1:2, and 1:1 molar ratios of Si to Fe. These solutions were placed into the atomizer reservoir and magnetically stirred during the synthesis process. Argon at 30 psi was passed through the atomizer to form droplets of toluene containing the Si and Fe precursors, which evaporated in the heated tubing as it was transported to the burner head. At the burner head, the precursor decomposed and oxidized to form monomers, which grew into composite nanoparticles and agglomerates through condensation, sintering, coagulation, and agglomeration.
Magnetite and silicon dioxide composite nanoparticle aggregates were synthesized using a FLAR with atomizer precursor delivery. The phase separation of these materials was confirmed with X-ray powder diffraction (SI). The composition of the nanoparticle aggregates was controlled by varying Si and Fe precursor molar ratios in a toluene-based atomizer solution. At the burner head, the atomized droplets decomposed and oxidized to form monomers of Si and Fe oxides. In the flame, these monomers underwent nucleation, and then grew by condensation, coagulation, and agglomeration. A quench ring was used to freeze particle growth and was placed 7.5 cm above the burner head. By varying the precursor concentration and flame residence time, the size of the synthesized particles and the extent of agglomeration were controlled.
Materials and chemicals
Tetraethyl orthosilicate (TEOS 99.999%, Sigma Aldrich) and iron (III) acetylacetonate (99.9% trace metals grade, Sigma Aldrich) were utilized as Si and Fe precursors. Toluene (99.99%, Sigma Aldrich) was used as a solvent for these chemicals within the atomizer. Teflon filters collected the excess particles that were characterized using offline analysis.
Characterization
The morphology and size of the composite nanoparticle aggregates were characterized by transmission electron microscopy (TEM, Tecnai Spirit, FEI Company, Hillsboro, OR, USA). TEM images were then analyzed by using the ImageJ software (Rasband, W.S., ImageJ, U.S. National Institutes of Health, Bethesda, MD, USA, http://imagej.nih.gov/ij/, 1997-2015). X-ray powder diffraction (XRD) was used to verify the phases present for all the samples and was carried out using a Bruker D8 Advance (Bruker AXS, Germany). The particle size distribution of the flame synthesized nanoparticle aggregates was measured with an SMPS (TSI, Model 3080) and elemental composition was characterized by ICP-OES (OPTIMA 7300DV, Perkin Elmer Co., Waltham, MA, USA). The wavelengths selected for ICP-OES intensity measurements were 252.85 nm for Si and 239.56 nm for Fe. These wavelengths were selected due to their high signal-to-noise ratio and to avoid spectral interference between the two elements. Coupling these instruments provided real-time size-resolved elemental composition measurements.
Results and discussion
Particle size increased as the precursor molar concentration was increased due to increased coagulation rate of the higher number concentration of particles that were formed. TEM images in show the size and morphology of the synthesized particles with only Fe precursor () and with a ratio of 1:4 Si to Fe (). TEM images show that the particles are highly agglomerated with a primary particle size ranging from 4.2 to 5.6 nm () as the concentration of Fe stays constant at 1M and Si precursor increased from 0 to 1M. SMPS was used to determine the geometric mean diameter for number particle size (GMD #). Using Equation Equation(1)[1] to account for the fractal structure of the agglomerates, the geometric mean diameter for surface particle size (GMD SA), and geometric mean diameter for mass particle size (GMD Mass) were calculated. As observed in , agglomerate GMD # increases from 72.1 to 102.1 nm as the concentration of Si increases and the GMD SA and GMD Mass increase from 88.2 to 135.8 nm. Due to the fractal structure of the composite nanoparticle aggregates, GMD SA and GMD Mass were calculated to be the same for all cases.
Figure 2. Transmission electron microscope images of iron oxide and iron oxide/silicon oxide composite nanoparticle aggregates synthesized using the flame aerosol system. (a) Fe; (b) 1:4 Si:Fe.
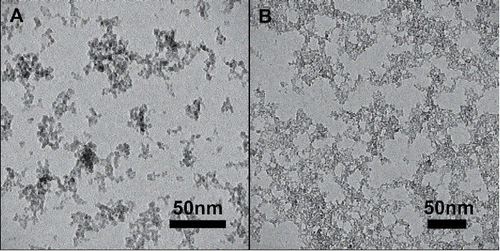
The size distribution data were also compared against the mass distribution for each element by coupling the SMPS and ICP-OES. Elemental concentration data were collected for monodisperse segments of the selected bins of the size distribution as classified by the SMPS. To assure that there was no drift in particle size over time, the size distribution was measured before and after the coupled SMPS-ICP-OES data were collected. It should be noted that carbon wavelengths were monitored during SMPS and ICP-OES coupled analysis to assure soot was not synthesized due to incomplete combustion within the flame. shows that peak Si and Fe concentrations measured from the ICP-OES match closely with the GMD #. This correlation to number concentration has been shown previously by Hess and Losert in 2015, although ICP-OES intensity is a function of mass ablated in the plasma, thus correlation to mass concentration is expected assuming efficient particle ablation in the plasma. To better illustrate this relationship, number and mass distributions for the case of 1:4 Si to Fe molar ratio particles were compared to the elemental mass distribution of Si and Fe in and . Number distribution was found to have the strongest correlation to the peak concentration for Si and Fe. shows a correlation between the Si and Fe concentrations and the number concentration while shows a large portion of the mass concentration calculated from the SMPS data is unmeasured by the ICP-OES, with larger nanoparticle aggregates being particularly under-represented. Particle losses and incomplete ablation cannot be ruled out as contributing to the unmeasured mass, but small fluctuations in the fractal dimension and fractal prefactors of the aggregate nanoparticles could also play a role. Future work studying elemental composition of nanoparticle aggregates should focus on coupling a centrifugal particle mass analyzer to ICP-OES or ICP-MS. While previous works, including those on single particle ICP-MS, have shown success in analyzing particles of many materials within the size regime described in this article, we were unable to find particle ablation efficiencies for agglomerated magnetite and silicon dioxide nanoparticles within ICP-OES systems. Future work will require full characterization of the ablation efficiency of a wide variety of nanoparticle chemical compositions and morphologies to understand the limitations of aerosol characterization using ICP-OES.
Figure 3. SMPS-ICP-OES results for composite nanoparticle aggregates synthesized with 1:4 Si:Fe molar ratio, comparing particle number concentration to ICP-OES intensities for iron (a) and silicon (b).
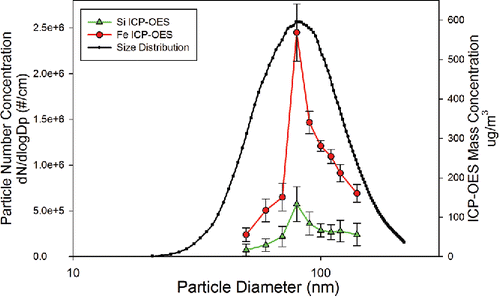
Figure 4. SMPS-ICP-OES results for composite nanoparticle aggregates synthesized with 1:4 Si:Fe molar ratio, comparing particle mass concentration to ICP-OES intensities for iron (a) and silicon (b).
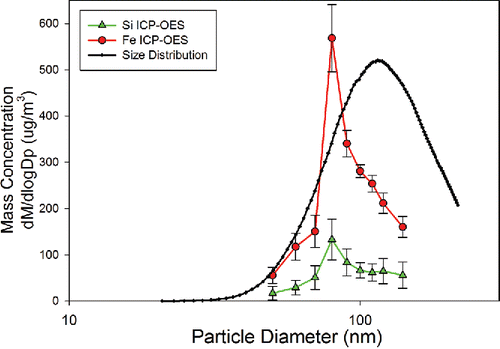
Elemental ratios of Si to Fe were compared in for particle diameters between 50 and 140 nm. From the analysis of these synthesized particles, it can be seen that these concentration ratios vary slightly throughout the size distribution but follow the trend of the expected ratio for each precursor molar ratio. Variation from the expected ratio is most notable for 1:1 Si to Fe molar ratio nanoparticle aggregates where the ratio of Si to Fe varies between 0.876 and 1.126. The variation in Si to Fe for 1:4 and 1:2 Si to Fe molar ratio particles is less extreme. If the elemental composition of each size of nanoparticle were uniform, the elemental ratios would remain constant throughout the size distribution. From the elemental ratios' results from the 1:4, 1:2, and 1:1 Si to Fe precursor molar ratios, it is hypothesized that measured elemental concentrations of Si and Fe are nearly constant throughout the size distribution with some variation. The variation measured is attributed to incomplete particle ablation in the plasma, non-linear detector response, different aggregation kinetics for the magnetite and silicon dioxide nanoparticles, or that a combination of these factors plays a role. Since there is a stronger correlation between number concentrations of the particles with the elemental concentrations, the measured concentrations do not directly correlate to the mass entering the ICP-OES and thus incomplete atomization of the particulate matter is thought to at least be partially accountable.
Conclusion
SMPS and ICP-OES were successfully coupled, allowing for elemental analyses of magnetite and silicon dioxide composite nanoparticle aggregates synthesized in a flame aerosol reactor. Size-resolved elemental analysis provided insights into the elemental distribution of Si and Fe for these nanoparticle aggregates. The data confirm that peak elemental concentrations of Si and Fe match GMD for number particle size for highly agglomerated Si and Fe oxide composite nanoparticles. The results highlight that this analytical technique is applicable in the study of flame synthesized nanoparticles and their aggregates. For characterization of nanoparticle dopants, this technique could easily be transitioned to ICP-MS due to its orders of magnitude improved detection limits for most elements. This in situ characterization of nanoparticle elemental concentration could also be implemented to aid in quality control of FLAR processes by providing real-time data necessary to make process changes on the fly. This work draws a roadmap for a more exhaustive determination of precursor fates in the aerosol synthesis of nanoparticles and their aggregates.
UAST_1271110_Supplementary_File.zip
Download Zip (16.5 MB)Acknowledgments
This work was performed in part at the Nano Research Facility (NRF) of Washington University in St. Louis which was previously a member of the National Nanotechnology Infrastructure Network (NNIN).
Funding
Partial support provided by the CMMN Grant NIH-NCI U54CA199092 is gratefully acknowledged.
References
- Bochert, U., and Dannecker, W. (1989). On-Line Aerosol Analysis by Atomic Emission Spectroscopy. J. Aerosol Sci., 20:1525–1528.
- Bochert, U., and Dannecker, W. (1992). Single Particle Analysis of Aerosols by Atomic Emission Spectrometry. J. Aerosol Sci., 23:417–420.
- Crawford, S., Thimsen, E., and Biswas, P. (2009). Impact of Different Electrolytes on Photocatalytic Water Splitting. J. Electrochem. Soc., 156:H346–H351.
- Ehrman, S., Friedlander, S., and Zachariah, M. (1998). Characteristics of SiO2/TiO2 Nanocomposite Particles Formed in a Premixed Flat Flame. J. Aerosol Sci., 29:687–706.
- Gupta, A. K., and Gupta, M. (2005). Synthesis and Surface Engineering of Iron Oxide Nanoparticles for Biomedical Applications. Biomaterials, 26:3995–4021.
- Harra, J., Nikkanen, J.-P., Aromaa, M., Suhonen, H., Honkanen, M., Salminen, T., Heinonen, S., Levänen, E., and Mäkelä, J. (2013). Gas Phase Synthesis of Encapsulated Iron Oxide–Titanium Dioxide Composite Nanoparticles by Spray Pyrolysis. Powder Technol., 243:46–52.
- Hess, A., Tarik, M., and Ludwig, C. (2015). A Hyphenated SMPS–ICPMS Coupling Setup: Size-Resolved Element Specific Analysis of Airborne Nanoparticles. J. Aerosol Sci., 88:109–118.
- Jiang, J., Chen, D.-R., and Biswas, P. (2007). Synthesis of Nanoparticles in a Flame Aerosol Reactor with Independent and Strict Control of their Size, Crystal Phase and Morphology. Nanotechnology, 18:285603.
- Jiang, Y., Wang, W.-N., Biswas, P., and Fortner, J. D. (2014). Facile Aerosol Synthesis and Characterization of Ternary Crumpled Graphene–TiO2–Magnetite Nanocomposites for Advanced Water Treatment. ACS Appl. Mater. Interf., 6:11766–11774.
- Kawaguchi, H., Fukasawa, N., and Mizuike, A. (1986). Investigation of Airborne Particles by Inductively Coupled Plasma Emission Spectrometry Calibrated with Monodisperse Aerosols. Spectrochim. Acta Part B: Atom. Spectrosc., 41:1277–1286.
- Li, S., Ren, Y., Biswas, P., and Stephen, D. T. (2016). Flame Aerosol Synthesis of Nanostructured Materials and Functional Devices: Processing, Modeling, and Diagnostics. Prog. Energy Combust. Sci., 55:1–59.
- Liu, P., and Chakrabarty, R. (2016). Sensitivity Analysis of Aggregate Morphology on Mass-Mobility Relationship and Improved Parameterizations. Aerosol Sci. Technol., 50(1):63–70.
- Losert, S., Hess, A., Ilari, G., and von Goetz, N., Hungerbuehler, K. (2015). Online Characterization of Nano-Aerosols Released by Commercial Spray Products using SMPS–ICPMS Coupling. J. Nanopart. Res., 17:1–14.
- Myojo, T., Takaya, M., and Ono-Ogasawara, M. (2002). DMA as a Gas Converter from Aerosol to “Argonsol” for Real-Time Chemical Analysis using ICP-MS. Aerosol Sci. Technol., 36:76–83.
- Nomizu, T., Nakashima, H., Hotta, Y., Tanaka, T., and Kawaguchi, H. (1992). Simultaneous Measurement of the Elemental Content and Size of Airborne Particles by Inductively Coupled Plasma Emission Spectrometry Combined with the Laser Light-Scattering Method. Analyt. Sci., 8:527–531.
- Okada, Y., Yabumoto, J., and Takeuchi, K. (2002). Aerosol Spectrometer for Size and Composition Analysis of Nanoparticles. J. Aerosol Sci., 33:961–965.
- Sahu, M., and Biswas, P. (2011). Single-Step Processing of Copper-Doped Titania Nanomaterials in a Flame Aerosol Reactor. Nanoscale Res. Lett., 6:1–14.
- Sorensen, C. (2011). The Mobility of Fractal Aggregates: A Review. Aerosol Sci. Technol., 45(7):765–779.
- Tiwari, V., Jiang, J., Sethi, V., and Biswas, P. (2008). One-Step Synthesis of Noble Metal–Titanium Dioxide Nanocomposites in a Flame Aerosol Reactor. Appl. Catal. A: Gen., 345:241–246.
- Wang, W.-N., Park, J., and Biswas, P. (2011). Rapid Synthesis of Nanostructured Cu–TiO2–SiO2 Composites for CO 2 Photoreduction by Evaporation Driven Self-Assembly. Catal. Sci. Technol., 1:593–600.
- Weber, A., Baltensperger, U., Gäggeler, H., Tobler, L., Keil, R., and Schmidt-Ott, A. (1991). Simultaneous in-situ Measurements of Mass, Surface and Mobility diameter of Silver Agglomerates. J. Aerosol Sci., 22:S257–S260.
- Weber, A. P., Keil, R., Tobler, L., and Baltensperger, U. (1992). Sensitivities of Inductively Coupled Plasma Optical Emission Spectrometry for Dry and Wet Aerosols. Analyt. Chem., 64:672–677.
- Wegner, K., and Pratsinis, S. E. (2003). Scale-up of Nanoparticle Synthesis in Diffusion Flame Reactors. Chem. Eng. Sci., 58:4581–4589.
- Worathanakul, P., Jiang, J., Biswas, P., and Kongkachuichay, P. (2008). Quench-Ring Assisted Flame Synthesis of SiO2-TiO2 Nanostructured Composite. J. Nanosci. Nanotechnol., 8:6253–6259.