ABSTRACT
Continuous ultraviolet germicidal irradiation (UVGI) has been extensively studied, but research on pulsed UVGI (PUVGI) is lacking and has primarily focused on disinfection of solid surfaces or liquids. This study addressed the gap in knowledge on the effectiveness of pulsed UVGI for disinfecting virus-laden calm air, with relevance to indoor rooms. Φ6 bacteriophage (a surrogate used to study communicable enveloped human respiratory viral pathogens such as influenza virus) was aerosolized by a Collison device into an enclosed test chamber, wherein the bioaerosol was exposed to PUVGI. The spectral content and performance of a pulsed white light lamp with a substantial UVC component were defined. Pulsed UV exposure of 10 to 30 s resulted in a two-log reduction in viable recovered virus from filter membranes and cyclone-based samplers. The small differences in Φ6 survival, after 10 to 30 s of exposure, emphasized the difficulty of complete eradication. However, exposure to 10 s of PUVGI resulted in significant reduction of virus viability. The dose–response displayed clear regimes of fast and slow exponential decay. Susceptibility factor for the fast-decay regime of aerosolized Φ6 (Z = 0.24 m2/J) was similar to those reported for influenza A virus aerosols at similar relative humidity. Our study demonstrated the potency of PUVGI against a viral bioaerosol. This has potential implications for the control of infectious bioaerosols in the healthcare setting.
© 2017 American Association for Aerosol Research
EDITOR:
Introduction
Seasonal outbreaks of viral respiratory disease, such as influenza, represent a significant healthcare burden. Peccia et al. (Citation2008) note that the dilute nature of biological materials in air is a significant barrier to identifying, quantifying, and describing their environmental fate. They estimate a range of bioaerosol concentrations within the environment that spans six orders of magnitude (1000 to 0.001 infectious units/L). Further research on transmission and decontamination of virus in air is needed to give evidence for enhanced control and prevention measures. Irradiation with ultraviolet (UV) light can neutralize pathogenic micro-organisms by damaging nucleic acids. Typically 0.01% to 1% of a microbial population (but as high as 10% for certain species; Kowalski and Bahnfleth Citation2000a; Kowalski et al. Citation2000) is resistant to inactivation by irradiation for prolonged exposures due to genetic variation or physical clumping (Mitscherlich and Marth Citation1984). Relative humidity (RH) may also affect inactivation. Studies with bacteriophages, including Φ6, found that UV susceptibility was lower at 85% RH than at 55% RH, possibly because more water sorption on the virus surface may protect against UV-induced damage (Tseng and Li Citation2005). Similarly, the susceptibility of aerosolized influenza virus (A/Puerto Rico/8/1934 H1N1) to UVC doses of 4 to 12 J/m2 decreased with increasing RH (McDevitt et al. Citation2012).
Continuous ultraviolet germicidal irradiation (UVGI) is well-established for the electromagnetic radiation wavelength range of 225 nm to 302 nm (Kowalski and Bahnfleth Citation2000a). Implementation of UVGI to disinfect air in the upper, unoccupied regions of rooms was motivated by the need to control the spread of tuberculosis and measles in public spaces (Wells et al. Citation1942; Perkins et al. Citation1947). UVGI of atomized influenza virus A/Puerto Rico/8/1934 H1N1 prevented infection of ferrets inoculated with recovered centrifugate from the infected air (Wells and Brown Citation1936). UV doses of greater than 40 J/m2 were required for 100% typical kill rate of influenza virus (Kowalski and Bahnfleth Citation2004).
Pathogen populations exponentially decay with exposure to irradiation as described by N = N0e−kIt, where N is the population size at time t, N0 is the population size at t = 0, I is irradiation intensity, and k is the standard decay rate constant (Mitscherlich and Marth Citation1984). The product of I and UV exposure duration (t) is the dose. This relationship implies reciprocity between irradiation intensity and time of action as it is simply the dose that determines the germicidal effect (Bunsen and Roscoe Citation1862). The relationship between UV dose and the natural logarithm of survival fraction is linear (McDevitt et al. Citation2012). The resilience of a micro-organism to UVGI is quantified by the gradient of this line, which is known as the UV susceptibility constant or Z-value (with units of m2/J). Influenza A virus in air has intermediate resilience (0.12 m2/J from Kowalski et al. Citation2000 referencing Jensen Citation1964; 0.22 to 0.29 m2/J from McDevitt et al. Citation2012) between strongly resilient Aspergillus asexual spores (conidia) (0.003 m2/J) and weakly resilient vegetative cells of S. aureus bacteria (0.960 m2/J). For the same micro-organism, different researchers have found Z-values can vary by a factor of five, which may be due to the use of different strains of the species or differences in experimental technique (Kowalski et al. Citation2000). A single linear regression may not be adequate as three regimes were required to describe the dose–response of S. aureus (Sharp Citation1940): (Equation1[1] ) a “shoulder” regime, from zero to a threshold dose, where a microbe negligibly responds to UVGI or even recovers from irradiation damage; (Equation2
[2] ) “first-stage” inactivation or exponential “fast-decay” of the vulnerable majority of the microbial population; (Equation3
[3] ) “second-stage” inactivation or exponential “slow-decay” of the resilient minority of the microbial population. Shoulder parameters for reovirus and two-stage decay parameters for adenovirus and Coxsackie virus are available (Kowalski et al. Citation2000).
Pulsed irradiation has been applied to sterilization of medical devices/packaging and disinfection of water (Kowalski Citation2009). Identified deficiencies in design guides for UVGI systems were insufficient consideration of lamp location, lamp type, surface reflectivity and variability between pathogen species, as well as inadequate definition of the irradiation intensity field by an inverse-square law (Kowalski and Bahnfleth Citation2000b). High-powered pulsed UV lamps emit a broad band of wavelengths with a large energy contribution from the UVC range and their output is accurately described as pulsed white light (PWL) with a large UVC component. Irradiation outside the UVC range reputedly also has germicidal benefits. The UV fluences measured for pulsed and continuous UV lamps are shown to be in good agreement (although the former can have noticeably higher power outputs than the latter), yet PUVGI has been found to be approximately twice as effective as continuous UVGI, at equivalent dose, for inactivation of T4 or T7 phage (Bohrerova et al. Citation2008). By modeling pulsed irradiation as a series of rectangular waves, a cumulative dose can be defined as the sum of the dose of every pulse. In the absence of pulsation-related effects, PUVGI treatments of differing power and time of action are equivalent if they have the same cumulative dose, while the cumulative dose–response is expected to follow exponential decay. Most studies with pulsed UV lamps only examine doses in the second stage of a cumulative dose–response curve (Kowalski Citation2009).
Wekhof (Citation2000) and Krishnamurthy et al. (Citation2010) identified possible biocidal mechanisms of pulsed UV irradiation: photochemical effects (e.g., induced photodimers in nucleic acids); photothermal effects (e.g., localized heating for >5 s of UVGI); and photophysical effects (e.g., structural damage due to a pulsing energy field, or the sudden vaporization of water content leading to cell wall rupture, attributed to the UVA and infrared content of PWL and reported as more evident at higher power levels). Furthermore, ozone is produced by dissociation of oxygen by shortwave UV radiation (Chapman Citation1930) and its germicidal effect has typically been overlooked. Tseng and Li (Citation2006) found that 18.4 s of contact time with 0.64 ppm of ozone at 55% relative humidity resulted in 90% inactivation of Φ6 within a 23 L cylinder. In practice, the overall disinfection effect of a germicidal system is of primary relevance.
PUVGI has mainly been studied with Staphylococcus and Aspergillus (Kowalski Citation2009). The aim of the present work is to study the effectiveness of PUVGI for inactivating an aerosolized surrogate of a human respiratory virus. The behavior of influenza virus is known to resemble that of Φ6 bacteriophage (Turgeon et al. Citation2014). Although Φ6 does not infect human cells, it is regarded as more similar to eukaryotic viruses than to other bacteriophages and, thus, is a proxy in studying communicable viruses between humans. Hence, the present work focuses on PUVGI of virus in calm air and utilizes Φ6 as a surrogate pathogen that is aerosolized into an enclosed chamber and exposed to pulsed white light.
Experimental methodology
Artificially generated aerosols containing RNA bacteriophage were introduced into a test chamber and the effect of various PUVGI exposures on reducing the viable viral burden of the droplets was assessed. The separate phases of each trial were generation of virus aerosol into the test chamber for 20 min, followed by PUVGI treatment (except in baseline trials with no PUVGI treatment) and then air sampling for 30 min using either open-faced filters that are commonly used for field assessment of indoor air quality or research-grade NIOSH BC 251 air samplers.
Bioaerosol chamber
Virus-laden airstreams were studied in a quiescent enclosed space, shown as and referred to as the FLUGIE (Savory et al. Citation2014), where air disturbances related to occupant movement or heating, ventilation, and air conditioning systems were minimized. This cubic chamber was amply sized (1.8 m per side) to allow study of the bioaerosol prior to the confining influence of the chamber walls upon the flow. The maximum and minimum values (i.e., range) of air temperature and relative humidity, within the test chamber during experiments, were measured using a digital thermometer and hygrometer (pen-type with integral probe; RP Electronics, Burnaby, BC, Canada) with temperature range, resolution, and accuracy of 0 to 50°C, 0.1°C, and ±1°C, respectively, and relative humidity range, resolution, and accuracy of 2% to 98%, 0.1%, and ±5%, respectively. The ambient conditions within the test chamber were representative of a typical indoor environment during the warm season of a humid continental climate (RH of 41% to 58%; air temperature of 21°C to 26°C). Interior surfaces of the test chamber were coated with a black matte finish in order to minimize reflections of light. The test chamber was sufficiently airtight to prevent any apparent flow or escape of introduced bioaerosol while permitting equalization of internal and external pressures via a gap between the Collison outlet and the test chamber inlet, which was sealed with plastic sheeting.
Figure 1. (a) Layout of the test chamber showing core components. (b) Layout of the test chamber showing centerline sampling locations (C1 & C2). (c) Layout of the test chamber showing an overhead view of corner sampling locations (NF: Near-inlet and Far-from-lamp; NN: Near-inlet and Near-lamp; FN: Far-from-inlet and Near-lamp; FF: Far-from-inlet and Far-from-lamp), where the proximity of the pathogen source to each air sampler is shown as dashed (red) lines, and the proximity of the irradiation source to each air sampler is shown as long-dashed (blue) lines. All numerical values are given in units of meters and for the case where all air samplers and both sources were located at 0.80 m above the test chamber floor.
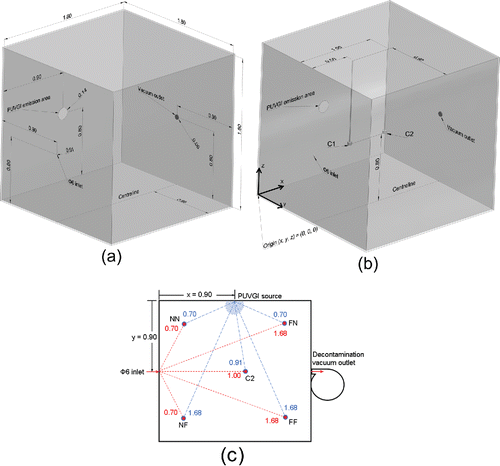
Pulsed irradiation
The pulsed xenon UVGI system (Solaris UV Technologies, Mississauga, ON, Canada) had a circular emission area of 0.140 m diameter (Figure S1 in the online supplementary information [SI]) and was mounted at the location labeled as “PUVGI emission area” in . The pulse frequency and pulse width (duration of each flash of irradiation) were fixed at 15 Hz and 100 µs, respectively. These values were selected based on known effective settings for PUVGI of contaminated surfaces. Emission at wavelengths of less than 190 nm was cut off. A new spiral bulb with 0.107 m diameter was installed at the start of the study. Exposure duration was set with an automated timer. The pulsed xenon lamp was mounted on a vertical wall of the test chamber with the bulb centered at 0.80 m above the test chamber floor (). There was unobstructed line-of-sight between the lamp and air sampling locations. Light reflections at the interior surfaces of the test chamber were negligible.
Cumulative dose (D) was determined as the product of pulse width (Δtp), pulsing frequency (fp), pulsing duration (Tp), and absolute irradiance (Ee) over the spectral band of interest (wavelength λ1 to wavelength λ2), according to Equation (Equation1[1] ).
[1]
Spectrometry
The spectral shape of emissions from the PUVGI system was characterized by a spectrometer (USB2000+, Ocean Optics, Dunedin, FL, USA; 2048-element CCD array detector) with a spectral range of 200 nm to 1100 nm. The spectrometer provided absolute spectral irradiance values with a wavelength resolution that varied from 0.39 nm at the short-wavelength end (200 nm) of the spectral measurement range to 0.29 nm at the long-wavelength end (1100 nm) of the spectral measurement range.
Absolute irradiance over particular spectral bands was determined by piecewise integration of the spectrometer output, following Simpson's rule. Equation (Equation2[2] ) was used to integrate over a bandwidth of 0.8 nm about local spectral peaks. The absolute irradiance over each of the UVC, UVB, UVA, visible and infrared (IR) spectral ranges was determined by applying the calculation shown by Equation (Equation2
[2] ) to adjacent three-point segments of the spectrometer data and summing the absolute irradiance from these segments over the spectral range of interest:
[2] where, Eeλ is the narrowband absolute irradiance at a nominal local peak wavelength; λp + 1 and λp − 1 are the neighboring measured wavelengths about the local peak wavelength; Ee, p is the measured absolute irradiance at the local peak wavelength; and Ee, p+1 and Ee, p−1 are the neighboring measured absolute irradiances.
The spectrometer was positioned at various distances from the PUVGI emission area (y = 0.43, 0.93, and 1.40 m). The spectrometer was horizontally leveled such that its receiving face was parallel to the PUVGI emission area and in line with the lamp bulb.
Aerosolized bacteriophage
Overnight cultures of Φ6 bacteriophage (Félix d'Hérelle, Québec City, QC) were propagated in a lawn of their bacterial host Pseudomonas syringae at 25°C. Plates with confluent plaques were selected. Soft agar was gently removed from plates, placed into a conical tube containing 5 mL of sterile culture media, mixed gently for 20 min, and then centrifuged at 3500 RPM for 10 min at room temperature. Titration by plaque assay in P. syringae was done to confirm that the filtered phage lysate contained 1010 plaque-forming units per milliliter (PFU/mL).
A mixture of one part of Φ6 to fourteen parts of phosphate-buffered saline was aerosolized with a standard three-jet Collison nebulizer (Mesa Laboratories, Lakewood, CO, USA) that was operated in steady state at 6 L/min (138 kPa = 20 psig) for a duration of 20 min during each trial. The only airstream introduced into the test chamber was from the Collison, at the location labeled as “Φ6 inlet” in , with the total introduced volume corresponding to 2% of the test chamber volume or a theoretical maximum internal pressure rise after nebulization of 2 kPa (0.3 psig) in the idealized case of perfect airtightness of the test chamber.
Bioaerosol sampling
Two types of air samplers were used, in turn, to sample air at a flow rate of 4000 mL/min per sampling device over a duration of 30 min. The majority of trials involved filtration through polytetrafluoroethylene (PTFE) membranes of 1.0 μm pore size and 37 mm diameter (SKC Inc., Eighty Four, PA, USA), which are commonly used for field assessment of indoor air quality. A filter and cellulose support pad were held within a clear styrene cassette cylinder and exposed to the sampled air (Figure S2a in the SI). For polydisperse NaCl particles aerosolized from 0.34% (v/v) NaCl solution with a 6-jet Collison nebulizer, Soo et al. (Citation2016) reported minimum collection efficiencies of 99.03% and 99.95% with flow rates of 2.5 L/min and 4.4 L/min, respectively, through PTFE filters with a pore size of 1 μm.
Additional trials were conducted with a version of the two-stage cyclone sampler developed at the National Institute for Occupational Safety and Health (NIOSH, Morgantown, WV, USA; Blachere et al. Citation2009; Cao et al. Citation2011; Figure S2b in the SI). The NIOSH BC 251 sampler collected non-respirable particles, with aerodynamic diameter >4 µm, in a first-stage 15 mL centrifuge tube. Respirable particles were collected in a second-stage 1.5 mL tube (particles with aerodynamic diameter of 1 to 4 µm) or on a terminal PTFE filter (particles with aerodynamic diameter <1 µm). With a flow rate of 3.5 L/min, 50% cut-off sizes (and sharpness) for the NIOSH BC 251 sampler were specified as 4.1 µm and 1.0 µm (1.51 geometric standard deviations and 1.59 geometric standard deviations) for the first stage and second stage, respectively.
Each open-faced PTFE filter cassette or BC 251 sampler was attached to a constant-flow air sampling pump (Airchek 224-PCXR3, SKC Inc., Eighty Four, PA, USA), which drew air out of the test chamber at a flow rate of 4000 ± 40 mL/min that was verified with a calibrator (DryCal DC-1, BIOS International, Lakewood, CO, USA), before and after each set of trials. Both types of samplers were suspended from the chamber roof and, at a given sampling location, the flow was drawn through the same sampling pump and Tygon tubing.
depicts the open-faced PTFE filter cassettes and a co-ordinate system (x, y, z) for identifying sampling locations. PTFE filters were initially deployed at the locations identified as “C1H” and “C2H” in , which are at two distances downstream of the Φ6 inlet on the chamber centerline and at Φ6 inlet height. Set-up details for each trial are presented in the SI (Table S1). Two sampling pumps were concurrently operated during these trials; thus, 120 L of Φ6 bioaerosol was introduced at the Collison nebulizer and then a total of 240 L of infected air was extracted from the test chamber during each trial.
Table 1. Air sampling locations, where increasing x is defined as the downstream direction perpendicular to the Φ6 inlet face, y is the downbeam direction perpendicular to the PUVGI emission face, z is vertically upward from the test chamber floor, and proximity is the minimal linear distance between two locations.
Further study of the spatial distribution of the aerosolized pathogen within the test chamber was conducted with both types of samplers, at various combinations of centerline (C1 and C2) and corner locations (NN, NF, FN, and FF). Two sampling location heights were investigated, corresponding to the Φ6 inlet height and half of this height, which are denoted by the identifiers “H” and “L,” respectively, in . Proximities of samplers to the bioaerosol and irradiation sources, as defined by minimal linear separation distance, are illustrated in and listed in . Details about these trials with PTFE filters and BC 251 samplers are shown in Tables S2 and S3, respectively, in the SI. Three sampling pumps were concurrently operated during these trials; thus, 120 L of Φ6 bioaerosol was introduced at the Collison nebulizer and then a total of 360 L of infected air was extracted from the test chamber during each trial.
Data extraction from bioaerosol samples
Each PTFE filter sample was eluted with 5 mL of sterile tryptic soy broth (TSB) and gently rocked for 20 min. Virus was eluted from stages 1 and 2 of the BC 251 sampler by rinsing with 0.25 mL of TSB and light vortexing (pulse-shaken 10 times for 2 s each time). Each final-stage filter sample from the BC 251 sampler was rocked as for the PTFE filter samples. Titration by plaque assay in the bacterial host P. syringae was performed as above. Recovered pathogen concentration was calculated from Equation (Equation3[3] ).
[3]
where Φ6 concentration had units of PFU/L of sampled air; Φ6 concentration from plaque assay had units of PFU/mL of elution media; elution media volume had units of mL; flow rate had units of L of sampled air per minute; and duration had units of minutes. Survival fraction (S) was calculated as the ratio of the recovered pathogen concentration after pulsed irradiation to the recovered pathogen concentration after no pulsed irradiation.
Purging of the test chamber
A vacuum system (Emerson, St. Louis, MO, USA) was used to evacuate the airborne contents of the test chamber into a separate exhaust containment and filtration unit as part of bacteriophage and ozone removal between trials (30 min run time = 25 test chamber volumetric air-changes). The vacuum system was mounted at the location labeled as “Vacuum outlet” in . It was only operated between experimental trials.
Results and discussion
Characterizing the PUVGI lamp emissions
The detection limit for measurements of absolute spectral irradiance with the spectrometer was 0.46 W/m2/nm. shows the three local peaks in the germicidal UVC band (230, 248, and 261 nm) that were identified. The irradiance was broadband and more accurately described as pulsed white light with a significant UVC component. Genetic material was reported to be particularly absorptive and vulnerable to UVC light at 254 nm. The vacuum UV region (<200 nm) is strongly absorbed by atmospheric oxygen and, thus, is expected to have a limited role for UVGI.
At the chamber centerline (y = 0.93 m), the absolute irradiances for the spectral peaks at 230 nm and 248 nm, for a bandwidth of 0.8 nm about each peak, were 7.8 and 4.3 W/m2, respectively, from Equation (Equation2[2] ) and averaged over duplicate trials. Closer to the PUVGI emission area (y = 0.43 m), the absolute irradiances of these spectral peaks were 35.0 and 15.7 W/m2, respectively, with corresponding dose per pulse of 0.00350 and 0.00157 J/m2, respectively. Thus, with total PUVGI exposure durations of 10 to 30 s and Equation (Equation1
[1] ), a representative range of the individual contribution to cumulative dose from a spectral peak with bandwidth of 0.8 nm, in our experiments, was 0.0645 to 1.58 J/m2. These cumulative peak doses were 2 to 960 times less than the reported continuous UVGI doses of previous investigators (3.2 to 62 J/m2; Gates Citation1929; Sharp Citation1938, Citation1940; Walker and Ko Citation2007; McDevitt et al. Citation2007, Citation2012). Although the spectral range of reported UVGI doses has not been clearly reported, dose values in the literature appear to be determined from measurements over a much broader spectral bandwidth than 0.8 nm.
UVGI levels reportedly decreased as the inverse of the square of the distance from a point source and as the inverse of the distance from a line source (Lin and Li Citation2002). In addition, surfaces may reflect varying amounts of UV light due to varying optical properties and, hence, affect the irradiance of the enclosed air. The attenuation with distance from the spiral lamp bulb was investigated at the three wavelengths of measured peak irradiance. An approximate inverse-square relationship between irradiance and distance from the lamp was observed (Figure S3 in the SI). Moderate deviations from an inverse-square relationship could be due to the spiral lamp bulb being a more complex geometry than a point source or a line source. The present measurements supported the reasoning that the irradiance can deviate from an exact theoretical inverse-square relationship with distance, since that type of relationship fails to account for the finite dimensions of the bulb.
Moreover, accurate determination of lamp output can be a notable factor in experimental design accuracy based on examining the dimensionless parameters governing pathogen survival in air after UVGI (Kowalski et al. Citation2003). With due care, the test chamber and the lamp bulb dimensions, as well as spatial locations, can be accurately determined within ± 0.5%. In addition to the challenges of assessing dose and response for the decay rate constant, the reflectivity of irradiated surfaces and the relative humidity, careful quantification of the lamp spectral irradiance field is needed, rather than simply relying on an inverse-square relation with distance, which does not account for the finite dimensions and complex geometry of a lamp bulb.
Sampling by open-faced filter
An initial round of trials established that a starting quantity between 108 and 1010 PFU/mL of Φ6 bacteriophage was desired to be certain that the aerosolized pathogen concentration substantially exceeded the detection limit of Φ6 bioaerosol sampling with PTFE filters. Control samples taken from the Collison reservoir showed that the bacteriophage concentration typically diminished from 108 PFU/mL of media before nebulization to 107 PFU/mL of media after nebulization. Our detection limit for sample collection of Φ6 bacteriophage by PTFE filter was 0.6 PFU/L of sampled air.
The results from the infectivity assay in showed that irradiating with pulsed UV light for at least 10 s reduced the pathogen concentration in the sampled air by at least a factor of 20 (from 200–300 PFU/L of sampled air to less than 10 PFU/L of sampled air). The present results suggested that irradiation of no more than 10 s can account for significant disinfection and a reduction of Φ6 bacteriophage concentration in air by two orders of magnitude.
Sampling by cyclonic devices
In addition to initial air sampling with open-faced PTFE filters at x = 0.5 m and x = 1.0 m, in triplicate, NIOSH BC 251 cyclone air samplers were operated at these same sampling locations. Our detection limit for Φ6 bacteriophage sample collection by BC 251 sampler was 2.3 PFU/L of sampled air. shows that the recovered viable bacteriophage particles, with no exposure to UVGI, were predominantly in the 1 to 4 µm size range from the second-stage (1.5 mL) collection tube. Relatively insignificant concentrations of viable pathogen were recovered from the 15 mL first-stage collection tube (>4 µm particles) and no viable pathogen associated with sub-micron particles was recovered on the terminal filter.
Figure 4. Recovered pathogen mainly found at intermediate particle size in the BC 251 samplers for trials without PUVGI. Recovered pathogen concentration values shown are the mean and standard deviation from duplicate trials (n = 2).
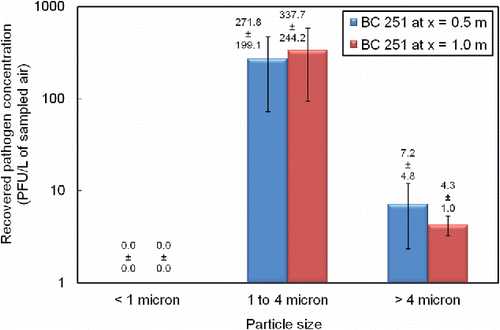
Furthermore, after 30 s of exposure to PUVGI, no viable pathogen was recoverable from the BC 251 air samplers. The present study found the PTFE filter to be a more sensitive tool for viral recovery since it recovered small amounts of Φ6 after 30 s of PUVGI, however, the PTFE filter does not separate recovered particles as respirable and non-respirable fractions. Since the exposed face of the 37-mm-diameter PTFE filter presented a sampling area of 1075 mm2 while the BC 251 inlet area was approximately 20 mm2, given identical flow rates, the air speed into the latter device would be about fifty times larger than the former with a greater likelihood of turbulence and shear flow that may mechanically damage viral material. Other possible reasons for this result include lower extraction efficiency from the microcentrifuge tube than from the PTFE filter and greater potential for unrecovered virus between the multiple stages of the BC 251 device as compared with the single-stage collection with the PTFE filter.
Broadband UVC dose–response
The broadband dose at the test chamber centerline was primarily comprised of contributions from the UVC and IR bands, which contributed 73% of the total measured absolute irradiance (). The UVC dose–response (), where cumulative UVC dose was determined from Equation (Equation1[1] ), showed two distinct regimes of exponential decay (fast- and slow-decay) and the tailing phenomenon associated with a resilient pathogen sub-population. The smallest non-zero dose was too large for a shoulder to be observed, like in other PUVGI studies (Kowalski Citation2009). Survival fraction exhibited a spread of about one log (base 10) over triplicate trials. The results at two distances from the bioaerosol inlet (x = 0.5 and 1.0 m) were alike (Figure S4 in the SI).
Table 2. Distribution of measured absolute irradiance over various spectral ranges.
Figure 5. Reduction in survival fraction on PTFE filters with increasing cumulative UVC dose. Survival fraction (S) was calculated as the ratio of the recovered pathogen concentration (for varying PUVC dose) to the recovered pathogen concentration for zero PUVC dose. Cumulative dose (DUVC) was calculated as the product of the exposure duration to PUVC, the pulsing frequency, the pulse width, and the UVC component of absolute irradiance (spectral band from 200 nm to 280 nm). Survival fraction values shown are the mean and standard deviation from triplicate trials at two locations (n = 6).
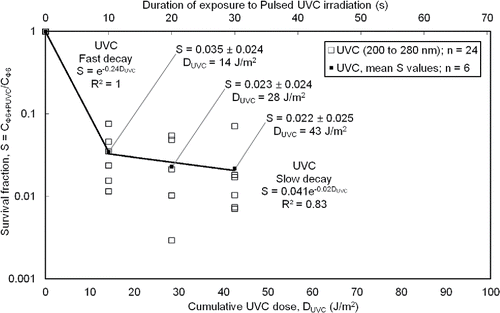
The cumulative UVC doses (14, 28, or 43 J/m2) from the pulsed lamp in our study were larger than, but within an order of magnitude of, the UVC doses (4 to 12 J/m2) from continuous lamps used with influenza A virus aerosols by McDevitt et al. (Citation2012). Our largest pulsed UVC dose, for 30 s of pulsing, exceeded the threshold for eradication of influenza virus (UVC dose > 40 J/m2; Kowalski and Bahnfleth Citation2004). The susceptibility factor in for the fast-decay regime of aerosolized Φ6 bacteriophage (Z = 0.24 m2/J) was slightly less but generally concurred with those reported by a modern study of influenza A virus aerosols at similar RH (estimated Z value of 0.27 m2/J at RH of 50% to 54%; McDevitt et al. Citation2012) and were less than the value reported for Φ6 exposed to continuous UVGI (0.43 m2/J; Tseng and Li Citation2005). The susceptibility factor for Φ6 in the slow-decay regime was an order of magnitude less than that in the fast-decay regime. In determining and reporting Z-value, it is important to specify the spectral content of the irradiation dose. The susceptibility factor for the fast-decay regime was 0.11 m2/J when the cumulative dose was calculated based on considering the irradiation as Pulsed White Light (200 nm to 892 nm; Figure S5 in the SI), i.e., less than half the value found from considering only the UVC contribution.
Spatial distribution of recovered bacteriophage
Higher Φ6 concentrations were recovered on PTFE filters, after exposure to 10 s of PUVGI, at air sampling locations closer to the chamber floor (Figure S6 in the SI). Overall, the concentrations of recovered Φ6 in these trials were low. However, these results are indicative of the gravitational settling of the infectious aerosolized droplets. More generally, the basic premise of germicidal irradiation is to arrange the intersection of contaminated air and the effective range of the irradiation source. In the case of contaminated air introduced into a larger enclosed volume of calm air (e.g., a cough in an indoor room), strong variation of pathogen distribution across space and time, together with the steady spatial variation of the germicidal irradiation field, presents a design challenge for innovative disinfection tools, which may be mobile and programmed to sweep a chosen irradiation path through contaminated indoor air.
Ozone generated by the lamp
The UVGI system in the current study produced enough ozone, in close proximity to the lamp bulb and immediately after lamp pulsing, to be detectable by the human nose but not enough to produce pulmonary responses in healthy young adult lab workers. Thus, the maximum ozone concentration in the present experiments was estimated to have exceeded 0.02 ppm but to have remained below 0.08 ppm (Lippmann Citation1989; International Ozone Association from http://www.ozoneservices.com/articles/007.htm). Since (i) our estimated maximum ozone concentration was at least eightfold less than in Tseng and Li (Citation2006), (ii) our test chamber volume was 250 times that of Tseng and Li (Citation2006) and allowed for significant dilution of the ozone detected near the UVGI bulb, and (iii) 25 complete air changes were induced by our vacuum system between each trial, it was unlikely that ozone was more significant than PUVGI for the inactivation of Φ6.
Study limitations
An inherent challenge of the infectious bioaerosol research problem is that dose and pathogen concentration can strongly vary with spatial location and time. The difficulty and cost of collecting each data point are not trivial. In this study, measurement locations were chosen that were likely to be representative and of practical interest (e.g., 1.0 m from the bioaerosol source). The spatial resolution of the measurements was chosen to allow for a feasible experiment. Refinement of the spatial resolution of these measurements is a logical extension upon this study.
These results indicated that measurements of absolute spectral irradiance are sensitive to the precise alignment of the receiving face of the spectrometer to the emitting face of the UV lamp. While these measurements were completed as carefully as possible, a focused study on alignment effects would be prudent.
Ozone is a natural by-product of operating germicidal pulsed xenon lamps. It is possible that ozone had a minor germicidal effect in these experiments and ozone levels can be quantified by direct measurement. In practice, the critical information about a disinfection system is the overall germicidal effect and this study shows how the system effectiveness varies for different settings within a given airspace. Whether disinfection is from ultraviolet irradiation alone, or a combination of UV and ozone, is not a major issue in the application of these systems.
Conclusions and future work
Pulsed UVGI exposure of 10–30 s resulted in a two-log reduction in viable recovered pathogen with standard recovery techniques in calm enclosed air. For moderate relative humidity (around 50% RH), the susceptibility factor for the fast-decay regime of aerosolized Φ6 bacteriophage (Z = 0.24 m2/J) was similar to values reported for influenza A virus aerosols. Studies of smaller doses are needed for complete identification of susceptibility factors and shoulder effects in the dose–response of Φ6 and other pathogens. The small differences in Φ6 survival over a threefold increase in PUVGI dose supported previous findings indicating that eradication is difficult. The range of pulsing durations in our experiments corresponded to cumulative doses of 14 to 43 J/m2 within the UVC spectral band. The spectral range of reported UVGI doses needs to be carefully reported since pulsed irradiation sources tend to be broadband and portions of the electromagnetic spectrum outside of the UVC range may also be germicidal. Based on the guidance available from expert specialist knowledge on ozone levels, our ozone concentrations were relatively low and our relatively large chamber volume allowed for significant dilution. The germicidal effect of ozone warrants consideration and more data on this aspect of UVGI systems are needed. Future work directly comparing the performance of continuous and pulsed UVGI systems against infectious bioaerosols, with a single test facility, would be useful.
UAST_1280128_Supplemenatl_File.zip
Download Zip (1.5 MB)Acknowledgments
Mr. Vinod Kumar and Mr. Valentino Ramanand of TBM Service Group offered guidance on using the PUVGI system. Ms. Ushma Naik performed the assays. Dr. William Lindsley shared instructions for using the NIOSH air samplers. Dr. Daniel Verreault advised about the nebulizer.
Funding
This work was supported by an Engage grant from The Natural Sciences and Engineering Research Council of Canada.
References
- Blachere, F. M., Lindsley, W. G., Pearce, T. A., Anderson, S. E., Fisher, M., Khakoo, R., Meade, B. J., Lander, O., Davis, S., Thewlis, R. E., Celik, I., Chen, B. T., and Beezhold, D. H. (2009). Measurement of Airborne Influenza in a Hospital Emergency Department. Clin. Infect. Dis., 48(4):438–440.
- Bohrerova, Z., Shemer, H., Lantis, R., Impellitteri, C. A., and Linden, K. G. (2008). Comparative Disinfection Efficiency of Pulsed and Continuous-Wave UV Irradiation Technologies. Water Res., 42(12):2975–2982.
- Bunsen, R. W., and Roscoe, H. E. (1862). Photochemical Researches — Part V. On the Measurement of the Chemical Action of Direct and Diffuse Sunlight. Proc. R. Soc. Lond., 12:306–312.
- Cao, G., Noti, J. D., Blachere, F. M., Lindsley, W. G., and Beezhold, D. H. (2011). Development of an Improved Methodology to Detect Infectious Airborne Influenza Virus Using the NIOSH Bioaerosol Sampler. J. Environ. Monit., 13(12):3321–3328.
- Chapman, S. (1930). A Theory of Upper-Atmospheric Ozone. Mem. R. Meteorol. Soc., 3(26):103–125.
- Gates, F. L. (1929). A Study of the Bactericidal Action of Ultra Violet Light. J. Gen. Physiol., 13(2):231–260.
- Jensen, M. M. (1964). Inactivation of Airborne Viruses by Ultraviolet Irradiation. Appl. Environ. Microbiol., 12(5):418–420.
- Kowalski, W. J. (2009). Ultraviolet Germicidal Irradiation Handbook. Springer-Verlag, Berlin.
- Kowalski, W. J., and Bahnfleth, W. (2000a). UVGI Design Basics for Air and Surface Disinfection. HPAC Eng., 72(1):100–110.
- Kowalski, W. J., and Bahnfleth, W. (2000b). Effective UVGI System Design Through Improved Modeling. ASHRAE Tran., 106(2):721–730.
- Kowalski, W. J., and Bahnfleth, W. P. (2004). Proposed Standards and Guidelines for UVGI Air Disinfection. IUVA News, 6(1):20–25.
- Kowalski, W. J., Bahnfleth, W. P., and Rosenberger, J. L. (2003). Dimensional Analysis of UVGI Air Disinfection Systems. Int. J. HVAC&R Res., 9(3):347–364.
- Kowalski, W. J., Bahnfleth, W., Witham, D. L., Severin, B. F., and Whittam, T. S. (2000). Mathematical Modeling of Ultraviolet Germicidal Irradiation for Air Disinfection. Quant. Microbiol., 2:249–270.
- Krishnamurthy, K., Tewari, J. C., Irudayaraj, J., and Demirci, A. (2010). Microscopic and Spectroscopic Evaluation of Inactivation of Staphylococcus Aureus by Pulsed UV Light and Infrared Heating. Food Bioprocess Technol., 3:93–104.
- Lin, C.-Y., and Li, C.-S. (2002). Control Effectiveness of Ultraviolet Germicidal Irradiation on Bioaerosols. Aerosol Sci. Technol., 36(4):474–478.
- Lippmann, M. (1989). Health Effects of Ozone. A Critical Review. JAPCA, 39(5):672–695.
- McDevitt, J. J., Lai, K. M., Rudnick, S. N., Houseman, E. A., First, M. W., and Milton, D. K. (2007). Characterization of UVC Light Sensitivity of Vaccinia Virus. Appl. Environ. Microbiol., 73(18):5760–5766.
- McDevitt, J. J., Rudnick, S. N., and Radonovich, L. J. (2012). Aerosol Susceptibility of Influenza Virus to UV-C Light. Appl. Environ. Microbiol., 78(6):1666–1669.
- Mitscherlich, E., and Marth, E. H. (1984). Microbial Survival in the Environment. Springer-Verlag, Berlin.
- Peccia, J., Milton, D. K., Reponen, T., and Hill, J. (2008). A Role for Environmental Engineering and Science in Preventing Bioaerosol-Related Disease. Environ. Sci. Technol., 42(13):4631–4637.
- Perkins, J. E., Bahlke, A. M., and Silverman, H. F. (1947). Effect of Ultra-Violet Irradiation of Classrooms on Spread of Measles in Large Rural Central Schools. Am. J. Public Health Nations Health, 37(5):529–537.
- Savory, E., Lin, W. E., Blackman, K., Roberto, M. C., Cuthbertson, L. R., Scott, J. A., and Mubareka, S. (2014). Western Cold and Flu (WeCoF) Aerosol Study — Preliminary Results. BMC Res. Notes, 7:563, doi: 10.1186/1756-0500-7-563.
- Sharp, D. G. (1938). A Quantitative Method of Determining the Lethal Effect of Ultraviolet Light on Bacteria Suspended in Air. J. Bacteriol., 35(6):589–599.
- Sharp, D. G. (1940). The Effects of Ultraviolet Light on Bacteria Suspended in Air. J. Bacteriol., 39(5):535–547.
- Soo, J.-C., Monaghan, K., Lee, T., Kashon, M., and Harper, M. (2016). Air Sampling Filtration Media: Collection Efficiency for Respirable Size-Selective Sampling. Aerosol Sci. Technol., 50(1):76–87.
- Tseng, C.-C., and Li, C.-S. (2005). Inactivation of Virus-Containing Aerosols by Ultraviolet Germicidal Irradiation. Aerosol Sci. Technol., 39(12):1136–1142.
- Tseng, C.-C., and Li, C.-S. (2006). Ozone for Inactivation of Aerosolized Bacteriophages. Aerosol Sci. Technol., 40(9):683–689.
- Turgeon, N., Toulouse, M.-J., Martel, B., Moineau, S., and Duchaine, C. (2014). Comparison of Five Bacteriophages as Models for Viral Aerosol Studies. Appl. Environ. Microbiol., 80(14):4242–4250.
- Walker, C. M., and Ko, G. (2007). Effect of Ultraviolet Germicidal Irradiation on Viral Aerosols. Environ. Sci. Technol., 41(15):5460–5465.
- Wekhof, A. (2000). Disinfection with Flash Lamps. PDA J. Pharm. Sci. Technol., 54(3):264–276.
- Wells, W. F., and Brown, H. W. (1936). Recovery of Influenza Virus Suspended in Air and its Destruction by Ultraviolet Radiation. Am. J. Hyg., 24:407–413.
- Wells, W. F., Wells, M. W., and Wilder, T. S. (1942). The Environmental Control of Epidemic Contagion. I. An Epidemiologic Study of Radiant Disinfection of Air in Day Schools. Am. J. Hyg., 35(1):97–121.