ABSTRACT
Operational parameters for a single particle soot photometer (SP2) and a CETAC Marin-5 nebulizer were optimized for detection of particulates aerosolized from liquid samples. The sensitivity of nebulization efficiency on nebulizer input gas pressure, liquid sample flow rate, and alcohol doping of the sample were explored. The nebulization efficiency of the Marin-5 was found to be roughly independent of applied gas pressure once above a minimum pressure. The nebulization efficiency changed by ∼50% for an order of magnitude change in liquid sample flow. Doping the sample with isopropyl alcohol at a 1:1 ratio results in a ∼50% relative increase in nebulization efficiency over a broad range of liquid flows. These results should apply to all particulate materials in the size range studied. SP2 operational parameters including sheath and sample flow were explored to optimize detection of refractory black carbon (rBC) specifically via coupling to the nebulizer. The SP2 tested samples up to 5 cc s−1 with 100% detection of rBC in its size range of detection, with increased sample jet spread and corresponding lack of detected rBC in the air at higher flows, leading to a total undetected rBC mass fraction of ∼15% at 16 cc s−1. Varying sheath flow does not improve this result, which is significant because under reasonable Marin-5 operating conditions, the SP2 only samples a fraction of the total air flow out of the nebulizer. Recommended operational parameters for cases of sample with low rBC loadings are presented: first, when very little liquid sample is available; second, when considerable sample is available.
© 2017 American Association for Aerosol Research
EDITOR:
1. Introduction
Since 2007, measurements of black carbon (BC) particulate from snow, ice, and rainwater (McConnell et al. Citation2007; Kaspari et al. Citation2011; Ohata et al. Citation2011; Bisiaux et al. Citation2011) have been carried out in the aerosol phase after nebulization of water-based (or melted) samples. In these experiments, water samples containing refractory black carbon (rBC, using nomenclature as described in Petzold et al. Citation2013) are typically transported into a nebulizer via a peristaltic pump, aerosolized then passed to a single particle soot photometer (SP2, Droplet Measurement Technologies, Boulder, CO, USA; Schwarz et al. Citation2006) to measure rBC mass mixing ratio (MMR). More recently, there has been interest in measuring size distribution of the rBC as well (Schwarz et al. Citation2012, Citation2013; Mori et al. Citation2016). Different nebulizers have been tested to evaluate the size-dependent efficiency with which they aerosolize particulate (i.e., the fraction of particles transported into the nebulizer via liquid that are transported out in aerosol phase), which is critical to understanding the liquid-phase concentration of the different sized particles. Two nebulizers used in the early work (the CETAC U5000 AT+ ultrasonic nebulizer and a Collison type nebulizer) have each proven to have substantial limitations. The U5000 fails to nebulize particles larger than ∼700 nm with reasonable efficiency and exhibits an undesirable nebulization size-dependence, while the Collison has low enough overall efficiency to make measurements of low concentration (1 ng g−1 BC, fairly typical for Artic snow samples) samples challenging (Schwarz et al. Citation2012). Concentric pneumatic nebulizers (CPNs) are now emerging as the preferred equipment to study rBC in water via aerosolization. For instance, Wendl et al. (Citation2014) conclude that the Apex Q (High Sensitivity Sample Introduction System, Elemental Scientific Inc., Omaha, NE, USA), a CPN, demonstrates a lower size-dependence of nebulization efficiency compared to Collison or U5000 nebulizers between 100 and 1000 nm, and Mori et al. (Citation2016) report the CETAC Marin-5 (Teledyne CETAC Technologies, Omaha, NE, USA) nebulization efficiency to be very good (∼50%) and size independent over the range of particulate diameters 200–2000 nm.
Here, we explore the operation of the CETAC Marin-5 concentric pneumatic nebulizer to optimize its ability to efficiently aerosolize particulate from liquid. Further, coupling of the Marin-5 to the SP2 is explored to optimize detection of the aerosol for determinations of BC MMR and size distributions specifically. Three different issues are central to the task, and were tested independently. First is the nebulization efficiency of the Marin-5, defined as the ratio of particle number that emerges from the nebulizer in aerosol form to the number initially pumped into the nebulizer. We consider a number of parameters that can affect this efficiency, including the nebulizer's rate of sample uptake, nebulizer gas flow, and the liquid in which particulate is suspended. Second is the fraction of aerosol generated by the nebulizer that is sampled by the SP2, which can vary dramatically depending on SP2 gas flow parameters (namely, sample and sheath flow). Finally, the detection efficiency of the SP2 as a function of its sample flow is explored. These factors can be combined to provide the total efficiency of detection of available particulate in a sample. This is especially of interest for cases where there is either very little sample or very little total particulate available.
In Section 2, we present details of the Marin-5 and the SP2 relevant to this work. Section 3 presents the sensitivity studies carried out and their results. In Section 4, the results are assimilated into recommendations for optimal operating parameters for the Marin-5 and SP2 based on rBC sample loadings and availability. Again, note that the evaluations of nebulization efficiency are relevant to all particulates, not only rBC, but the net detection for the SP2 specifically is focused on rBC.
2. Instrumentation
The experimental setup used is shown schematically in . A test liquid sample, generally of less than a few milliliters in volume, is peristaltically pumped from a glass vial to the Marin-5 nebulizer system. Here, the sample is broken into small droplets on the order of 10 μm (Mori et al. Citation2016) by an expansion of ultra-pure gas concentrically located around a thin stream of the liquid. These droplets enter a heated chamber where much of the liquid evaporates, leaving behind particulates in the air stream. The Marin-5 removes the remaining water components by use of a chiller. Hence, dry aerosol is generated, which can be transported to the SP2 (or any other aerosol instrumentation). For these studies, electrically conductive tubing with no bends is used to minimize particle losses. The aerosol is then detected by the SP2, which permits calculation of aerosol sample concentration and, when appropriate, rBC size distributions.
Figure 1. A schematic of the experimental setup, including the peristaltic pump, nebulizer with N2 tank, and single particle soot photometer.
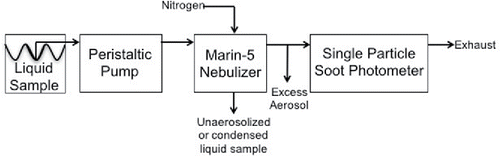
We begin our discussion in Section 1 by addressing the creation of the concentration standards used for testing. Section 2 covers the nebulization instrumentation, including the peristaltic pump that is used to drive liquid sample to the nebulizer at various rates. Section 3 provides a brief account of the basic operational principles of the SP2. Detailed explanations of SP2 performance and operation are available in other publications (Schwarz et al. Citation2006; Laborde et al. Citation2012). Here, the focus is on SP2 sensitivities relevant to sampling aerosol from the Marin-5.
2.1. Concentration and size distribution standards
Solutions of known concentration of rBC and monodisperse polystyrene latex spheres (PSLs) are essential for characterizing the nebulizer's performance on particulate. We use both gravimetric standards of refractory rBC and of several different size PSLs suspended in water. Fullerene soot (Alpha Asar, lot # F12S011) is used as a laboratory proxy for atmospheric rBC (Baumgardner et al. Citation2012). Three different dilutions (MMRs: 12, 14, 16 +/− 10% ng g−1) of well-characterized rBC gravimetric standard (Schwarz et al. Citation2010) were used. The lognormal mass size distribution of the rBC in this solution was centered on 190 nm and contains negligible mass outside the range of 80–500 nm volume equivalent diameter, assuming 1.8 g cc−1 void free density of the rBC. This size distribution closely mirrors that of rBC in typical ambient air, whereas the size distribution in snow and ice can tend toward larger sized particles (Schwarz et al. Citation2012, Citation2013).
Throughout all of these studies, fullerene soot standard aerosol size distributions were monitored for comparison to the size collected at typical running conditions. This ensured that any possible changes in size-dependent nebulization would be detected. No statistically significant shifts in size distribution were noticed after inspection of both the distribution's lognormal shape and mass-median diameter. We have also extended the study by Mori et al. (Citation2016) on the effects of coagulation of fullerene soot during nebulization with increasing concentrations, in the size range of 80–300 nm. Mori et al. show that for fullerene soot in samples with MMRs as high as 137 ng g−1, size distributions remain consistent at the 20% level. We found agreement with this when using fullerene soot standards with MMRs of 14, 53, and 96 ng g−1, then further extend the study to MMRs of 210 and 483 ng g−1, and still remained within 20% agreement.
PSL concentrations were determined by the manufacturer-provided solid fraction weight. This method has been previously checked against laser transmission spectroscopy (Schwarz et al. Citation2012), showing particle concentration agreement within 7%.
2.2. Nebulization system
A liquid solution is pumped to the Marin-5 nebulizer via a peristaltic system (Ismatec sa, model 7331-00) at a minimum fluid rate of 7 × 10−4 cc s−1 when using 0.030″ I.D. PVC tubing (Reinhard Glass Products, Golden CO, USA). Variability of this rate was measured over several-minute time scales 5 times over 6 months; the standard deviation in sample pump rate was 8% (likely dominated by differences in peristaltic tubing). Over time scales of 1 day, much smaller (i.e., negligible) changes were observed. Note that calibrations (either with PSL or BC gravimetric standards) should be run multiple times a day to ensure that any possible drift in nebulizer efficiency are sufficiently resolved. The peristaltic pump's rate can be incremented in discrete steps of this minimum (i.e., 7 × 10−4 cc s−1 × N, for N = 1,2,3…), where N is the number of revolutions per minute of the pump's rollers. The pump speed was measured at settings of N = 1, 5, and 10 rpm over which the resulting liquid flow rate showed good linearity.
From the peristaltic pump tube, the liquid sample enters the glass CPN. This consists of a narrow capillary tip concentrically surrounded by a sheath flow of pressurized gas supplied through the back of the Marin-5. The two meet at a critical orifice and the resulting gas expansion breaks the liquid stream into a fairly narrow droplet size distribution. In our setup, the pressurized gas stream to the nebulizer was supplied from a cylinder of nitrogen. Gas flow was controlled with a pressure regulator over the range 10–50 PSI corresponding to 10.4–31.4 cc s−1 volumetric flow (all gas flows in this article are volumetric) through the critical orifice formed by the CPN. Throughout most of these studies, gas flow rate was typically set to ∼16 cc s−1 volumetric flow at ambient pressure.
The Marin-5 was tested with two different glass CPNs of different critical orifice diameters (“MicroMist 500 μm” and “MicroMist 250 μm”). Most of our measurements were made with a 500 μm nozzle, but some testing was done with a 250 μm nozzle to evaluate any influences of nozzle size on nebulization.
The CPN is coupled into the Marin-5's heated chamber by an adapter that determines the angle between the droplet spray and the heated chamber. Most testing here is done with a 5° adapter that allows the droplets to remain as far from the heater walls as possible, but a 15° adapter was also briefly tested.
The droplets produced by the nozzle are dried at a user-defined temperature (here, 110°C) in the heated chamber, with water vapor subsequently removed from the aerosol by a chiller held at 5°C. This conditioned aerosol, which contains primarily non-soluble particulate from the liquid, typically had relative humidity <30% at laboratory temperature (23°C). The aerosol was transported to the SP2 via conductive silicon tubing, with the nebulizer and SP2 arranged to minimize bends in the transport line. Excess aerosol was vented to the room.
To establish a background contamination level of the system, distilled water was sampled frequently. To reduce contamination, distilled water was passed at high (for cleaning), then low (at typical running conditions) speed through the peristaltic pump to the nebulizer after every rBC-containing sample. The SP2 continuously monitored the resulting aerosol from this water. Background minimums were usually due to rBC in the blanks—typically the distilled water that we used contained rBC concentrations at or below the 0.5 ng g−1 level. We consider this sufficient to allow 1 ng g−1 samples to be quantified with ∼50% uncertainty. Based on observations with exceptionally clean blanks (an on occasion, very clean ambient samples), we are confident that the fundamental limit on obtaining low background is set by rBC contained in the blanks, and not from other contaminations in the system.
Distilled water sampled immediately following relatively high-rBC concentration samples (∼tens of ng g−1 rBC MMR) exhibited noticeably higher particle contamination. Restoring background levels to 0.5 ng g−1 could take half an hour or more of steady pumping, indicating that residual rBC had to be cleaned from the lines. One effective method used to clean the lines involved turning our peristaltic pump to high speeds (20 revolutions per minute), then introducing air bubbles in the lines to “scrub” them clean. During this time, larger contaminate (i.e., visible to the naked eye) could occasionally be seen in the tubing being carried away at each new air–water interface (i.e., at the trailing edge of each bubble).
The free end of the peristaltic pump line was frequently submerged in dirty samples in order to deliver the sample to the nebulizer. This served as another means to transfer contamination that has accumulated on the peristaltic pump line into background water sample. To avoid this, the peristaltic pump line was frequently rinsed with distilled water, and water samples and glassware were regularly refreshed with clean ones.
The studies presented in this article made use of suspensions with relatively moderate rBC MMRs (∼10 ng g−1), so background levels generally restored to the 0.5 ng g−1 level after less than a minute of implementing the mentioned cleaning techniques. However, if one can anticipate relative rBC concentrations in samples, we recommend running them from cleanest to dirtiest so that background contamination remains a small fraction of sample concentration. Note that contamination size distributions can differ from that of the sample, in particular causing problems at larger sizes. We assume that any non-BC particulate of interest will act similarly.
Marin-5 nebulization stability was tested using a fullerene soot liquid-standard. We sampled continuously over 5 h, and divided data into 12-min bins. The standard deviation of these efficiency values was less than 1% and no trends in efficiency were observed.
2.3. SP2 aerosol instrument
To detect rBC specifically, we employ a single particle soot photometer (SP2, Droplet Measurement Technology, Inc., Boulder, CO). The SP2's operation and performance is described in Schwarz et al. (Citation2010). The SP2, as operated here, was capable of detecting individual rBC aerosol particles over a size range between 0.8 fg and 7000 fg, and allowed quantification of both rBC mass content and size distribution.
The SP2 detects individual particles drawn through a capillary (here, 0.026″ but typically 0.017”) that injects the sample through a 0.028” nozzle that is surrounded by concentric sheath flow. It is passed to a continuous inter-cavity 1064 nm Nd:YAG laser inside of a chamber held near ambient pressure (in Boulder, CO ∼830 mb). Laser intensity is sufficiently high such that rBC particles more massive than 0.8 fg are heated to their vaporization temperature. The incandescing particles are surrounded by four channels for light detection, two of which are sensitive to different wavelengths of light incandesced from rBC. The remaining two channels detect scattered laser light from both refractory and non-refractory particles, and can be used to verify the presence of sufficient laser intensity when sampling monodisperse aerosol.
Once calibrated, the maximum peak height of an incandescent signal can be converted to the mass of the refractory particle. Here, we calibrate the SP2 using a differential mobility analyzer (DMA) to size select fullerene soot over a mobility diameter range of 125 nm to 350 nm (corresponding to rBC mass of ∼1 fg to 25 fg). Mobility diameter-to-BC mass conversions are published by Moteki and Kondo (Citation2010) and Gysel et al. (Citation2011). The incandescent signal is linearly proportional to particle mass for these small particles, whereas the relationship for larger particles is made via extrapolation using an empirical power law, also presented in Moteki and Kondo (Citation2010) as well as Schwarz et al. (Citation2012). The measurements presented here focus only on rBC morphology in accumulation mode mass range (∼1–400 fg).
3. Results
The data used for these studies were acquired in a laboratory at NOAA, Boulder, CO between July 2015 and June 2016. All data were obtained using the fullerene soot (mass) or PSL (number) concentration standards discussed above. These were sonicated for several minutes before use to ensure uniform distribution of particulate in the liquid. SP2 laser intensity was varied depending on the study, but in all cases, was above the minimum required levels for detection of rBC masses >0.5 fg described by Schwarz et al. (Citation2010). This was verified by measuring scatter signals from PSL standards.
We explore the dependence of nebulizer efficiency on Marin-5/peristaltic pump operational parameters, including gas flow, peristaltic pump speed, alcohol doping, and nebulizer adapter angle. These results, which are independent of detection instrumentation, are presented in Sections 3.1, 3.2, and 3.3, respectively. The temperature of the heated-chamber was also tested, with efficiency effectively constant between 100°C and 150°C. Section 3.4 presents SP2-specific detection efficiency dependencies on SP2 flow parameters.
We define the Marin-5 nebulization efficiency as the mass of rBC pumped into the nebulizer per unit time divided by the mass of rBC coming out of the nebulizer in aerosol form per unit time. In practice, this efficiency is expressed as[1] where [A] is the concentration of the aerosol generated by the Marin-5, [S] is the known concentration of the prepared sample, Rneb is the rate of gas flow into the Marin-5, and RP.P. is the rate the sample is pumped into the Marin-5 via a peristaltic pump. SP2 sampling detection efficiency, ϵSP2, is similarly defined as the fraction of a known number of particles introduced to the SP2 inlet line that are subsequently detected by the SP2, again corrected for system flows. In Sections 3.1–3.3, we use SP2 operating parameters such that ϵSP2 = 1.
Also of interest is how these efficiencies affect both the amount of time necessary to make a measurement of particle size distribution and the minimum sample size needed for the measurement. The number of collected particles needed to achieve a statistically significant size distribution will vary depending on the particulate being investigated. We have empirically determined this number to be ∼40,000 particles for typical ambient snow samples. However, this number will be driven by the existence of extremely rare particles large enough to contain a considerable fraction of the total BC mass. To the extent that such particles are present, it may be necessary to acquire substantially more than 40,000 particles to adequately represent the size distribution.
The duration for which a sample must be analyzed to obtain the size distribution will depend on all of four variables: the number concentration of particulate in the sample ([S]), the rate at which sample is fed to the nebulizer (R p.p.), the aerosol flow rate out of the nebulizer (which is the same as the rate of flow in, Rneb), and the SP2 sampling rate (RSP2). Three of these, the peristaltic pump rate, nebulizer air flow, and SP2 sample flow, introduce efficiency dependencies, ϵneb and ϵSP2. The efficiency ϵneb is a function of alcohol doping ratio, η (discussed in detail in Section 3.3). The theoretical time to measure a size distribution, (tS.D.), comprised of N particles can be written as[2] and the total efficiency of use of particulate in the sample is ϵuse = ϵSP2 * ϵneb*RSP2/Rneb. The minimum volume of liquid sample needed to obtain the desired number of particles is a function of [S], with the lower limit of SP2 MMR sensitivity being on the order of 1 ng g−1.
For these studies, we use both fullerene soot and PSLs. Marin-5 nebulization efficiency has already been shown to be independent of PSL size between 200 and 2000 nm (Mori et al. Citation2016), and this was verified in our lab for sizes between 220 and 3000 nm (PSL diameters 220, 356, 510, 1025, 1550, and 3000 nm, with 3000 nm corresponding to 14,000 fg on a mass basis) with 20% standard deviation. Fullerene soot and PSL (356 nm, 3μm) nebulization efficiencies were found to agree within uncertainties of 15%.
3.1. Marin-5 nebulization efficiency dependence on air flow
The absolute efficiency of nebulization of water-borne fullerene soot for the Marin-5 vs. the input gas flow (in our case, supplied by nitrogen gas) was measured over a range of ∼10 to 31 cc s−1 corresponding to N2 supply pressure from 10–50 PSI at ∼ 2 PSI intervals using the both the MicroMist 500 and 250 tip. Below this range, nebulization did not occur for the 500 tip, while above this range the system became prone to leaks at the nebulizer's gas-input connection.
Absolute nebulization efficiency is presented in for both tips. Two measurements on the 500 tip are shown to provide an estimate of measurement precision and stability. The figure shows a steady increase in absolute nebulization efficiency from just above 20% to just under 60% between 10.4 and 14.6 cc s−1. Beyond 14.6 cc s−1, the efficiency remains independent of gas pressure. The 250 tip shows slightly better performance at higher pressures, but we do not believe this is a significant result given the variability in measurements.
Figure 2. Absolute nebulization efficiency of the Marin-5 for flow rates (top axis) and pressures (bottom axis) applied to the gas inlet line. Data were acquired using a prepared 16 ng g−1 rBC sample, peristaltic pump rate of cc s−1, and an SP2 flow rate between 2 and 2.3 cc s−1. MicroMist 500 tip is shown in both open (2015) and closed circles (2016). MicroMist 250 tip is shown in stars.
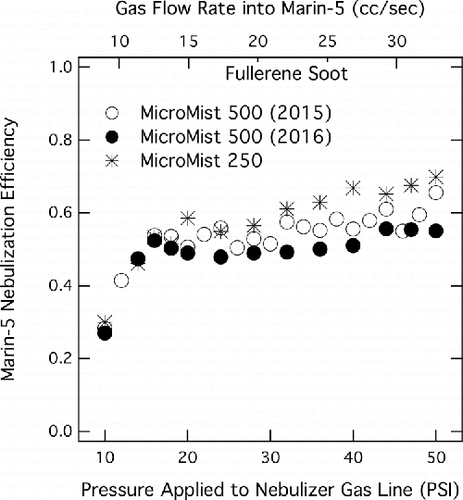
For subsequent studies discussed in this article, an input N2 flow of 16 cc s−1 is used, which is conservatively in the nebulizer efficiency plateau while still avoiding undue additional airflow and dilution of aerosol.
3.2. Nebulization efficiency vs. peristaltic pump speed
Absolute efficiency of nebulization vs. the peristaltic pump speed was measured over the range of 7 × 10−4 cc s−1 to 7 × 10−3 cc s−1 (1–10 RPM on our peristaltic pump). shows both absolute rBC nebulization efficiencies of the Marin-5 as well as the normalized aerosol concentration measured using the MicroMist 500.
Figure 3. Absolute nebulization efficiency of the Marin-5 (with MicroMist 500) for different peristaltic pump speeds, using samples of both pure and isopropyl-doped rBC (ratio of original sample to alcohol shown in legend). Open shapes are efficiencies, and closed shapes represent the normalized signal-per-second seen by the SP2. Each curve of signal per unit time is normalized to the signal rate measured by the SP2 for the specified doping ratio at a peristaltic pump rate of cc s−1.
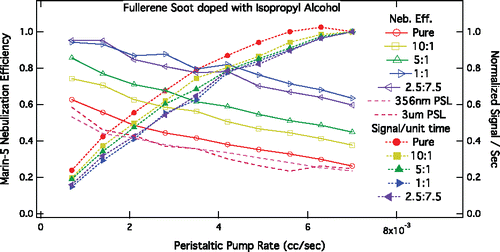
In all cases, there exists an immediate decrease in efficiency from 7 × 10−4 cc s−1 to 1.4 × 10−3 cc s−1, with a near-linear trend in drop off to 7 × 10−3 cc s−1. Our pure-sample data are consistent to within 5% of the measurements by Mori et al. (Citation2016), who measure a 50% nebulization efficiency at pump rate 3 × 10−3 cc s−1 and input gas flow rate of 16 cc s−1. Obviously, the aerosol concentration exhibits an opposing dependence on pump rate, and one may choose to sacrifice efficiency to achieve higher aerosol concentrations, depending on the particular measurement being made and sample availability.
Also shown in is absolute nebulization efficiency vs. pump rate for 356 nm and 3000 nm PSLs. There is good agreement between these two diameters, evidencing little or no size dependency for nebulization between this range. Further, these measurements agree with our pure rBC data at the 15% level.
3.3. Doping with alcohol
Water is a particularly difficult liquid to nebulize because of its high surface tension (∼72 dyn −1 at 25°C). Generally, alcohol or acetone is a preferred solution for nebulization due to its lower surface tension and boiling point compared to water. Both of these properties lead to a more efficient removal of liquid from particulate in the heated chamber. Hence, the effect of alcohol doping of water-based rBC samples on nebulization efficiency was performed. Samples were prepared by diluting water-based rBC suspension of known MMR with a measured mass of either isopropyl (surface tension 21.79 dyn cm−1, boiling point 82.6°C) or methyl (surface tension 22.32 dyn cm−1, boiling point 78.4°C) alcohol. To account for this doping in the efficiency calculations in the case of isopropyl alcohol, the original sample with mass mixing ratio MMR0 is corrected as[3] where V0 and malc are the volume of the original sample and mass of doping alcohol, respectively, and the density, ρ, of isopropyl (ethyl) alcohol is 0.786 (0.789) g cm−3.
shows nebulization efficiency for the isopropyl alcohol-doped samples at several different doping ratios using the MicroMist 500 at 5°. Ethyl alcohol data are not shown, as there was no significant difference between isopropyl and ethyl alcohol doping. A steady increase in absolute nebulization efficiency is exhibited as more alcohol is added to the sample until a doping ratio of 1:1 is reached, at which point no further increase in nebulization efficiency was observed. When this ratio is combined with low peristaltic pump speeds, we see near-100% nebulization efficiency.
This same experiment was performed using the MicroMist 250 tip. A quasi-efficient peristaltic pump rate of 3.5 × 10−3 cc s−1 was chosen so that relative gains or losses in efficiencies of the two tips would be evident. At times, the 250 tip exhibited as much as a 20% relative increase in nebulization efficiency compared to the 500 tip. However, this was on the same order as variability in the 250 tip measurements, and thus we cannot confidently conclude that it performs systematically better than the 500 tip.
Note that the increase in nebulization efficiency here comes at the cost of diluting the sample, which will increase total sample volume by more than a factor of two for a 1:1 doping ratio. Hence, while more rBC will make it from the liquid sample into the aerosol, aerosol concentrations will actually be lower, requiring more time to acquire the desired number of particles compared to using pure sample. Thus, alcohol doping is likely most useful when only very limited sample volumes are available.
3.4. SP2 detection efficiency vs. SP2 sample and sheath flow
Thus far we have investigated parameters that are related solely to the Marin-5′s ability to transfer particulate from a liquid suspension to an aerosol form. Optimizing nebulization of the liquid sample gives one the ability to minimize both the amount of time required to make a measurement, as well as the percentage of particulate wasted in the process. Both of these variables can also be affected by choice of SP2 operating parameters, namely, the sample flow rate.
At typical SP2 aerosol sample flow rates (∼1–4 cc s−1), it has been shown that every particle within the SP2 size range of sensitivity is detected (Schwarz et al. Citation2006, Citation2010). However, the largest potential loss of aerosolized particles from the nebulizer, if an SP2 is used as the sole detector, occurs because the SP2's typical sample flow is only a small fraction of the nebulizer flow output. Here, we examine the consequences of increasing the SP2 sampling rate beyond this typical range to reduce particle loss. To this end, the SP2's detection efficiency of rBC was measured over a wide range of sample flow rates. A low sample flow of 2 cc s−1 was used as a unitary-detection efficiency reference point. Our upper sample flow rate of 16 cc s−1 matched the total flow provided from the Marin-5 at its (conservatively) lowest highly efficiency backing pressure. We also recorded laser-light scattering data for both 220 and 505 nm PSLs at different flows. Using these monodisperse aerosols allows one to easily infer information about spreading of the sample jet in the laser beam because the light intensity varies across the laser beam in a well-understood manner.
shows the SP2's rBC detection efficiency (ϵSP2, defined at the beginning of Section 3) as a function of sample flow; there is a slow near-linear decrease in efficiency beginning at sample rates as low as 5 cc s−1. SP2 detection efficiency does however remain above 80%, even when the entirety of nebulized aerosol is sampled by the SP2, making these extreme upper sample flows potentially useful in some scenarios.
Figure 4. Measured rBC mass detection efficiency for several different SP2 sample flows and currents. Data are normalized to the average of measured MMR at 2 cc s−1 sample flow at three different laser currents.
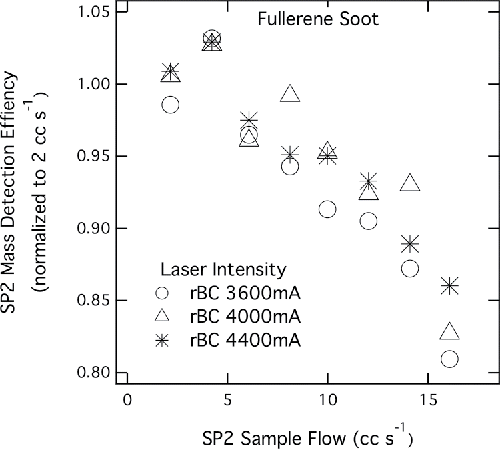
shows the probability distribution of scattering peak amplitudes from PSLs over the range of sample flows tested above. As sample flow increases, the aerosol jet can both increase in diameter and shift in position. This can result in particles experiencing a wider/different range of laser intensity than under lower flow conditions. We refer to this effect as jet spreading. We find that higher sample flows result in significant jet spreading at as low as 6 cc s−1 for both diameter PSLs tested. This effect worsens as sample flow increases. However, the effect is noticeably enhanced for 505 nm particles compared to 220 nm. This may indicate that even larger particles would experience still more dramatic spreading at elevated SP2 sample rates.
Figure 5. PSL scatter amplitudes for 220 nm (left data set) and 505 nm (right data set) at various SP2 sample flows. As SP2 sample flow increases, PSL peaks broaden. This effect appears to be enhanced for larger particles (i.e., 505 nm vs. 220 nm).
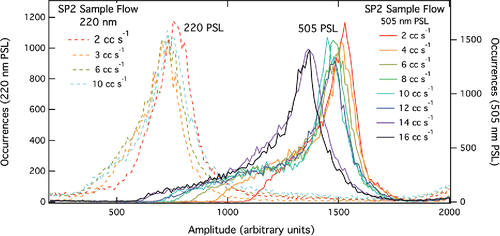
The SP2 uses clean sheath flow to constrain the sample flow near the center of the laser beam (held constant at 10 cc s−1 in previous studies). shows distributions of the detected scatter signal peak height from 505 nm PSLs measured over a range of sheath flows at ∼16 cc s−1 SP2 sample rate. Included is distribution obtained at standard conditions (2 cc s−1 SP2 sample flow and 10 cc s−1 sheath flow). We see that at 16 cc s−1 SP2 sample flow, the PSL peak broadens even more with decreasing sheath flow. We thus tested whether SP2 detection efficiency showed a dependence on sheath flow, potentially offering an opportunity to counter the jet spread by coaxing the particles on the fringe of the laser back toward the center. Further, we collected this data at several laser intensities to see if increasing fringe laser intensity recovered any losses. Sheath flow was varied over 3.7 to 13.5 cc s−1 at the upper sample flow limit of 16 cc s−1. The data in show no dependence of SP2 detection efficiency on sheath flow. They were also consistent with data from sample flow studies, collected 3 days prior, lending confidence to measurement reproducibility. Higher laser intensities did not appear to recover any losses in efficiencies.
4. Recommended running conditions
We have presented various dependencies for detection of water-based particulates in the aerosol form. How one chooses optimal operating conditions will depend on availability of sample, the amount of time one is willing to commit to a measurement, and the performance of the specific instruments in use. We encourage each user to independently measure the efficiencies associated with their particular setup, as they will certainly vary from the measured efficiencies of our specific setup.
Optimal settings will also depend on whether one wishes to measure MMR, size distribution, or both. Ideally, an MMR measurement would coincide with a size distribution measurement. However, especially for samples of very low rBC concentration, this requires a much larger time commitment to ensure a good statistical measurement over the entire size range of interest. In these situations, one may choose to make only a short measurement of MMR without a size distribution measurement (thus embracing the assumption that no rare, large particles exist in the sample). While the uncertainty in this measurement will be large—Schwarz et al. (Citation2012) estimate a 60% total uncertainty on MMR in snow samples between ∼100 and 2000 nm—it allows one to assess an MMR level with reasonable certainty in only a few minutes. For this reason, we separate the different statistical requirements to assess MMR at this level (roughly a factor of 2) as opposed to constraining the size distribution.
The issue of optimizing parameters to measure a size distribution can usually be reduced to one of three circumstances:
One wishes to maximize the percentage of particulate in the liquid sample that is detected
One wishes to minimize the time in which a measurement is made
One wishes to reduce signal to avoid multiple particles per trigger (particulate number is overwhelming)
presents several possible system operating conditions and their associated tS.D. for the NOAA Marin-5+SP2 setup for these different scenarios. This shows that under typical running conditions of 2 cc s−1 SP2 sample rate and the most efficient peristaltic pump rate, 0.2 ml of a low-concentration sample (∼1 ng g−1 rBC) is too small to permit acquisition of a statistically significant size distribution, as the SP2 will only detect well under 10% of the BC particles introduced to the nebulizer. However, if one dopes the sample with alcohol at a ratio of 1:1 and matches the SP2 sample flow to the nebulizer aerosol output rate, 0.2 ml of original sample is sufficient to collect 40,000 BC particles, even when running at a high (and thus less efficient) peristaltic pump rate. Under these circumstances, a size distribution could be acquired in as little as 1 min, while detecting better than 50% of the BC pumped to the nebulizer. Thus, we recommend a set of parameters similar to these latter conditions when available BC signal is low.
Table 1. Some examples of possible running conditions and the time needed to acquire a statistically significant size distribution, given very limited and very abundant availability of clean samples, or limited availability of dirty samples. Average particle size of 140 nm is used for calculations, with 1 ng g−1 used for clean samples and 10 ng g−1 for dirty samples. ϵuse is the fraction of total particulate in the sample that is detected by the SP2.
In the case where a relatively large amount of sample is available (e.g., 2 ml or more), one is free to be more liberal in wasting particulate signal in favor of minimizing the amount of time required to make the desired measurement. again shows the two extremes of operational parameters. Under typical running conditions of 2 cc s−1 SP2 sample rate and a low rate of peristaltic pumping of pure sample, nearly 20 min are needed to measures a size distribution. This time can be dramatically reduced by increasing the peristaltic pump speed, matching SP2 sample rate to nebulizer output flow rate and doping the sample with alcohol at a 5:1 ratio. A measurement of size distribution can then be made in about 1 min.
Finally, in the case where BC signal is overwhelming, one may wish to reduce the signal per unit time detected by the SP2 as much as possible. This is achieved by dropping both the peristaltic pump rate as well as the SP2 sample rate to very low settings. A size distribution for a liquid sample containing BC at the 50 ng g−1 level can still be achieved in roughly a couple of minutes. It can also be desirable to dilute the sample in these cases (simply with pure water).
For instances, where one only cares to make an MMR measurement, the cases are quite similar. If one has very little sample, it is advised to pump the liquid to the nebulizer at as slow of a rate as possible to extend the length of the measurement. Doping the sample will also increase the fraction of particulate seen. Conversely, if one has a large amount of sample but signal is low, it is best to increase sample pump rate to the nebulizer (sacrificing efficiency, but increasing signal per unit time) and do not dilute the sample in any way. Finally, if there is plenty of sample and signal above background, one may run at the lowest pump rate with water-diluted sample to avoid either clogging the sample line or multiple events per trigger. Unlike size-distribution measurements, one must wait for the signal rate to stabilize before collecting data to measure an MMR. For our system, this usually takes less than 1 min. In the system as we have tested it (down to 1 ng g−1 concentration rBC), particle statistics did not contribute strongly to the total uncertainties in MMR. Instead, the amount of time required to make a measurement of a liquid-based sample's MMR was primarily dependent on variability in the sample and the stability of the specific system being used, and thus will vary from setup to setup and for different samples.
Being a bit more general, we can say that alcohol doping is recommended at some level when one wishes to detect as large a fraction of the particulate in the sample as possible. If, however, time is a factor, it may be illogical to dilute the sample if it is already of reasonably low concentration, necessarily decreasing the rate of BC per unit time seen by the SP2. This should not be confused with the situation where one has an excessively dirty sample, in which case dilution (either with alcohol or water) may actually decrease the required time to make successive measurements by minimizing the time to clean the system between samples.
Only under circumstances where aerosol concentration is too high for convenient sampling does it make sense to drastically reduce the ratio of SP2 sample rate to nebulizer aerosol-out flow. In all other cases, the increase in SP2 detection efficiency gained at low flows is heavily outweighed by the amount of wasted BC being exhausted from the system before reaching the SP2.
5. Conclusions
Optimized aerosol-phase detection of particulates in liquid supports timely measurements of low-concentration and adequate measurements of low-volume samples. The optimizations carried out here have improved performance of the Marin-5 by a factor of 2 over previously published results. To clarify the various issues affecting net detection and sampling time requirements, we investigate both the efficiency of nebulization of the particulate-containing liquid as well as the sensitivity of SP2 particle detection to various flow parameters.
We have measured the efficiency of the Marin-5 nebulizer while varying input gas flow rate, liquid uptake rate, and amount of alcohol doping. Nebulization efficiency of water-based samples was found to be roughly independent of input gas rate once a minimum flow rate of 15 cc s−1 was achieved; increasing flow then merely diluted the resulting aerosol concentration. Increasing the rate at which sample was introduced to the Marin-5 at the uptake line by an order of magnitude, from 7 × 10−4 cc s−1 to 7 × 10−3 cc s−1 resulted in a <50% relative drop in nebulization efficiency, with maximum aerosol concentration observed at a flow rate of ∼5.6 × −3 cc s−1. Conversely, we observed a steady rise in nebulization efficiency with increasing alcohol doping ratio. Once the sample had sufficient alcohol content (1:1 or higher), nebulization efficiency was maximized at all liquid flow rates. At pump rates of less than 1.4 × 10−3 cc s−1, nebulization efficiencies near 100% were achieved, with higher pump rates being anti-proportional to nebulization efficiency. Alcohol doping increases efficiency, and thus the fraction of sample aerosolized, but does not increase aerosol concentration.
We also investigated how choosing SP2 sample and sheath flow rates can influence the range of laser intensities experienced by the aerosol. PSLs were used to map the aerosol jet in the laser, and rBC detection efficiency was measured to estimate the significance of the spread to rBC. The SP2, as configured here, can sample up to 4 cc s−1 with 100% detection of rBC in its size range of detection. However, above this rate the sample jet spreads enough that detection efficiency begins to drop, and at 16 cc s−1 ∼15% of rBC in the air is undetected by the SP2. Detection efficiency was found to be independent of sheath flow over a range of 3.7 to 13.5 cc s−1.
Funding
NOAA SP2 participation was supported by the NOAA Atmospheric Composition and Climate Program, the NASA Radiation Sciences Program, and the NASA Upper Atmosphere Research Program.
References
- Baumgardner, D., Popovicheva, O., Allan, J., Bernardoni, V., Cao, J., Cavalli, F., Cozic, J., Diapouli, E., Eleftheriadis, K., Genberg, P. J., Gonzalez, C., Gysel, M., John, A., Kirchstetter, T. W., Kuhlbusch, T. A. J., Laborde, M., Lack, D., Müller, T., Niessner, R., Petzold, A., Piazzalunga, A., Putaud, J. P., Schwarz, J., Sheridan, P., Subramanian, R., Swietlicki, E., Valli, G., Vecchi, R., and Viana, M. (2012). Soot Reference Materials for Instrument Calibration and Intercomparisons: A Workshop Summary with Recommendations. Atmos. Meas. Tech., 5:1869–1887. doi:10.5194/amt-5-1869-2012
- Bisiaux, M. M., Edwards, R., Heyvaert, A. C., Thomas, J. M., Fitzgerald, B., Susfalk, R. B., Schladow, S. G., and Thaw, M. (2011). Stormwater and Fire as Sources of Black Carbon Nanoparticles to Lake Tahoe. Environ. Sci. Technol., 45:2065–2071. doi:10.1021/es103819v
- Gysel, M., Laborde, M., Olfert, J. S., Subramanian, R., and Gröhn, A. J. (2011). Effective Density of Aquadag and Fullerene Soot Black Carbon Reference Materials used for SP2 Calibration. Atmos. Meas. Tech., 4:2851–2858.
- Kaspari, S. D., Schwikowski, M., Gysel, M., Flanner, M. G., Kang, S., Hou, S., and Mayewski, P. A. (2011). Recent Increase in Black Carbon Concentrations from a Mt. Everest Ice Core Spanning, 1860–2000 AD. Geophys. Res. Lett., 38:L04703. doi:10.1029/2010gl046096
- Laborde, M., Schnaiter, M., Linke, C., Saathoff, H., Naumann, K.-H., Möhler, O., Berlenz, S., Wagner, U., Taylor, J. W., Liu, D., Flynn, M., Allan, J. D., Coe, H., Heimerl, K., Dahlkötter, F., Weinzierl, B., Wollney, A. G., Zanatta, M., Cozic, J., Laj, P., Hitzenberger, R., Schwarz, J. P., and Gysel, M. (2012). Single Particle Soot Photometer Intercomparison at the AIDA Chamber. Atmos. Meas. Tech., 5:3077–3097. doi:10.5194/amt-5-3077-2012
- McConnell, J. R., Edwards, R., Kok, G. L., Flanner, M. G., Zender, C. S., Saltzman, E. S., Banta, J. R., Pasteris, D. R., Carter, M. M., and Kahl, J. D. W. (2007). 20th-Century Industrial Black Carbon Emissions Altered Arctic Climate Forcing. Science, 317:1381–1384. doi:10.1126/science.1144856
- Mori, T., Moteki, N., Ohata, S., Koike, M., Goto-Azuma, K., Miyazaki, Y., and Kondo, Y. (2016). Improved Technique for Measuring the Size Distribution of Black Carbon Particles in Liquid Water. Aerosol Sci. Technol., 50(3):242–254. doi:10.1080/02786826.2016.1147644
- Moteki, N., and Kondo, Y. (2010). Dependence of Laser-Induced Incandescence on Physical Properties of Black Carbon Aerosols: Measurements and Theoretical Interpretation. Aerosol Sci. and Techno., 44:663–675. doi:10.1080/02786826.2010.484450
- Ohata, S., Moteki, N., and Kondo, Y. (2011). Evaluation of a Method for Measurement of the Concentration and Size Distribution of Black Carbon Particles Suspended in Rainwater. Aerosol Sci. Technol., 45(11):1326–1336. http://dx.doi.org/10.1080/02786826.2013.824067
- Petzold, A., Ogren, J. A., Fiebig, M., Laj, P., Li, S.-M., Baltensperger, U., Holzer-Popp, T., Kinne, S., Pappalardo, G., Sugimoto, N., Wehrli, C., Wiedensohler, A., and Zhang, X.-Y. (2013). Recommendations for Reporting “Black Carbon” Measurements. Atmos. Chem. Phys., 13:8365–8379.
- Schwarz, J. P., Doherty, S. J., Li, F., Ruggiero, S. T., Tanner, C. E., Perring, A. E., Gao, R. S., and Fahey, D. W. (2012). Assessing Recent Measurement Techniques for Quantifying Black Carbon Concentration in Snow. Atmos. Meas. Tech., 5:2581–2592. doi:10.5194/amt-5-2581-2012
- Schwarz, J. P., Gao, R. S., Fahey, D. W., Thomson, D. S., Watts, L. A., Wilson, J. C., Reeves, J. M., Darbeheshti, M., Baumgardner, D. G., Kok, G. L., Chung, S. H., Schulz, M., Hendricks, J., Lauer, A., Kärcher, B., Slowik, J. G., Rosenlof, K. H., Thompson, T. L., Langford, A. O., Loewenstein, M., and Aikin, K. C. (2006). Single-Particle Measurements of Midlatitude Black Carbon and Light-Scattering Aerosols from the Boundary Layer to the Lower Stratosphere. J. Geophys. Res., 110:D16207. doi: 10.1029/2006JD007076.
- Schwarz, J. P., Gao, R. S., Perring, A. E., Spackman, J. R., and Fahey, D. W. (2013). Black Carbon Aerosol Size in Snow. Sci. Rep., 3:1356–1360. doi:10.1038/srep01356.
- Schwarz, J. P., Spackman, J. R., Gao, R. S., Perring, A. E., Cross, E., Onasch, T. B., Ahern, A., Wrobel, W., Davidovits, P., Olfert, J., Dubey, M. K., Mazzoleni, C., and Fahey, D. W. (2010). The Detection Efficiency of the Single Particle Soot Photometer. Aerosol Sci. Technol., 44:612–628. doi: 10.1080/02786826.2010.481298
- Wendl, I. A., Menking, J. A., Färber, R., Gysel, M., Kaspari, S. D., Laborde, M. J. G., and Schwikowski, M. (2014). Optimized Method for Black Carbon Analysis in Ice and Snow Using the Single Particle Soot Photometer. Atmos. Meas. Tech., 7:2667–2681. doi:10.5194/amt-7-2667-2014