ABSTRACT
Sampling for culturable (e.g., viable) aerosolized microbes (bioaerosols) is a useful means to provide information for public health monitoring and studies. However, it is challenging to maintain microbe culturability when sampling at high flow rates (>12 L/min) and extended periods of time (≥4 h). We developed a first-generation, viable bioaerosol collection system (VBCS) utilizing temperature (T) and relative humidity (RH)–conditioned filtration at a flow rate of 25 L/min. A two-stage system of tube-in-shell Nafion™ exchange units provides cooling to ≤10°C and RH conditioning to 80–95%. Aerosol particles are collected on a polyurethane nanofiber filter providing a physical collection efficiency of >95% for sizes 0.06–10 µm. The T and RH conditions at the collection filter are maintained, despite changes to ambient conditions. The initial testing of the VBCS was done under indoor, laboratory conditions with aerosolized, vegetative E. coli. A scenario of a 30-min challenge of bioaerosol followed by continued sampling of clean air for various times was used to judge culturability maintenance under extended-term sampling. An initial loss of culturability upon collection onto the filter was observed; 23 ± 13% relative to 4-mm all-glass impinger. However once collected, 98% of culturability was maintained for an additional 4.5 h of sampling. An exponential decay in culturability was observed from 8 h to 15 h of sampling. Also, 24-h cold storage of the filters collected was studied. The VBCS is based on the use of dry filter cassettes, needs minimal maintenance, and preserves culturability of vegetative bacteria for >4 h.
© 2017 RTI International and Aerosol Dynamics Inc.
EDITOR:
1. Introduction
Airborne microorganisms (e.g., bacteria, viruses, fungal spores) are ubiquitous in indoor and outdoor environments (Bowers et al. Citation2011; Burge Citation1995; Caruana Citation2011; Despres et al. Citation2012; Ghosh et al. Citation2015; Nazaroff Citation2016; Walser et al. Citation2015; Xu et al. Citation2011), and they can have a significant impact on human health (Griffiths and Decosemo Citation1994; Douwes et al. Citation2003; La Rosa et al. Citation2013; Morawska et al. Citation2013; Walser et al. Citation2015) and the environment (Bowers et al. Citation2011; Burrows et al. Citation2009; Despres et al. Citation2012; Lighthart Citation1997). Although advancements in DNA-based (i.e., PCR) detection provides for rapid identification of collected microbes, there is value in the collection of culturable, or more broadly viable, microbes. Samples with viable microbes enable a more detailed assessment of infectivity and health risks. Yet, currently used methods for viable collection (e.g., impingers, filters, agar-base impactors) are not operable for extended periods. Additionally, there are challenges to maintaining viability of microorganisms because the structure of some are hardier (e.g., spores) than others (e.g., viruses and vegetative bacteria). For systematic public health monitoring and large, extended-term research studies, there is a need for methods capable of long-term (e.g., 4 h to 12 h, to days) viable bioaerosol sampling that are simple to perform, provide samples that are easy to handle, have low operational cost, and preserve microbial viability.
A critical factor in long-term viable sampling of bioaerosols, especially for vegetative bacteria, is desiccation. Several researchers showed that wet collection typically provides better viability maintenance (King and McFarland Citation2012; Li et al. Citation1999; Wang et al. Citation2001). Examples of methods for wet collection include impingers, such as the all-glass impinger (e.g., AGI or SKC BioSampler), cyclone or wet-walled cyclone (e.g., Coriolis sampler of Bertin Instruments), and, more recently, a viable condensation growth tube collector (Lednicky et al. Citation2016; Walls et al. Citation2016). Although each method prevents desiccation, there are still challenges that make them less than ideal for long-term sampling. Traditional impingers are limited to sampling times of less than 30 minutes (Lin et al. Citation1997). Impingers, impactors, and cyclones have poor physical collection efficiencies for sizes below approximately 0.3–0.7 µm (Kesavan and Sagripanti Citation2015; Nevalainen et al. Citation1993). Loading of the collection liquid with particles and evaporation of fluid (unless refreshed) are common problems for most liquid-based samplers. In some cases, low flow rates are used to help mitigate evaporation, but then the low flow rate may under-sample the air.
Filter collection is simpler for in-field servicing, sample handling, and transport, and it typically provides better than 85% physical collection efficiency for particle sizes from viruses (sub-0.3 µm) to clusters of spores (>7 µm), but current filtration methods do not maintain culturability. Sampling times must be short (e.g., 20 min) or even shorter for some vegetative bacteria that are sensitive to stress. However, Karwowska et al. (Citation2003) found that maintaining filter humidity greater than 50% improved culturable bioaerosol sampling, thus suggesting a potential solution for the challenge of long-term filter sampling. As with wet collection, there is the potential for growth of the microorganisms sitting in a high-humidity environment at ambient temperature for extended periods of time. This problem might be overcome by refrigeration, which has been shown to retard the growth and death of microbes (Kango Citation2010). The combination of controlled filter humidity and refrigerated temperature (≤10°C) should provide viability maintenance during longer-term sampling.
Our hypothesis is that a sampling system that provides temperature- and humidity-controlled filtration collection of aerosolized microbes could provide longerterm, viable bioaerosol sampling than classical sampling methods while keeping much of the simplicity of filter collection. To test our hypothesis, we engineered and evaluated a first-generation humidity- and temperature-controlled filtration system that we call the viable bioaerosol collection system (VBCS). Specifically, we aimed to condition the sampled air stream without substantial microorganism loss (i.e., less than 1 log loss) to near refrigerator temperatures while controlling the relative humidity (RH) to avoid condensation or desiccation, followed by collection onto a cooled nanofiber filter with high collection efficiency over the range of likely microorganism sizes. Our objective was to evaluate this approach for an easily handled and well-characterized vegetative bacterial aerosol, E. coli. For ease of experimentation, we assessed culturability as a simple method for measuring viability, although it is understood that not all viable bacteria are culturable (Barer and Harwood Citation1999; Kell et al. Citation1998). Additionally, we examined the effects of sample storage after collection. Reported here are our initial efforts conducted under controlled laboratory conditions of mild temperature and humidity changes and relatively clean air (compared to the complexity of outdoor air).
2. The viable bioaerosol collection system (VBCS)
The first-generation VBCS is a humidity- and temperature-controlled, nanofiber filter collection system intended for fixed site monitoring (as opposed to a personal sampler), as illustrated in . Ambient air is drawn into a two-stage humidity and temperature conditioning system that cools the air to approximately 7°C and adjusts the RH to a range between 80 and 90%. The two-stage approach enables control of the RH during the cooling process, so as to avoid both condensation and desiccation, and it enables operation over relatively wide swings in ambient conditions. The aerosol is collected from this conditioned, approximately 7°C, 80–90% RH air stream, using a polyurethane (PU) nanofiber filter housed inside the cooled chamber. The VBCS samples at a flow rate of 25 L/min. We selected a flow rate of 25 L/min to provide a balance of high enough flow rate to detect a short-term incident of bioaerosol while allowing the design of equipment to minimize stress that the microbes may experience from high-volume sampling (e.g., 500 L/min; Kesavan and Sagripanti Citation2015; Li et al. Citation1999). The aerosol particles, including bioaerosols, are collected onto a 25 mm PU nanofiber filter held in a standard air sampling cassette. After collection, the filter is removed and placed in cool storage. Analyses are conducted in the laboratory by using a simple extraction buffer that is compatible with plating for culture to estimate viability (i.e., viable counting as estimated from culturability) or other microbiology analytical techniques.
The sampler was custom built by the research team by using a combination of commercial off-the-shelf components, custom-ordered parts designed by the team, and in-house custom-made parts. Because of the complexity of the instrument and the extensive details for fabrication, only the processes directly associated with the sampled air stream are discussed here. Other key features, components, and processes are described in the online supplemental information [SI].
The inlet for the sampled air used in this study was a custom-made isokinetic nozzle for 25 L/min that faced into a test rig flow of approximately 8,000 L/min. (Note that an omnidirectional nozzle could be developed for more general monitoring applications.) After the nozzle, the sampled air is drawn into the tube side of a Nafion™ tube-in-shell humidity exchange unit designed by the research team and custom fabricated by Perma Pure (PH150-11060T-0301; Lakewood, NJ, USA). This Nafion exchange unit is composed of 50 TT-110 Nafion tubes, 2.2 mm inner diameter, 25 cm long, and housed in an acrylonitrile butadiene styrene (ABS) shell. This configuration is similar to a tube and shell heat exchanger (Incropera and DeWitt Citation1990). Using 50 tubes results in a reduction of the flow rate to approximately 0.5 L/min per tube, which should minimize particle losses in the tubes. The Nafion membrane readily allows water moisture transport based on the gradient in absolute water content of the sample air (tube side) and the conditioning air (shell side). However, contaminates such as microbes and particles do not cross the membrane barrier, maintaining the integrity of the sample. Heat exchange also occurs, providing for modification of the temperature.
The partially conditioned sample stream then transitions from the first-stage Nafion exchange unit into a refrigerated space that contains the second-stage Nafion exchange unit and the sampling filter. This second-stage Nafion unit is identical to the first stage, allowing for further cooling and humidity adjustments of the sampled air. Finally, the sampled air, at the target temperature and humidity conditions, flows into a filter cassette where the aerosol particles, including microbes, are collected on the PU nanofiber filter. This two-stage process with refrigerated space achieves a temperature at the sampling filter that ranges between approximately 7 and 10°C and an RH that ranges between approximately 80–95%, depending upon the temperature and absolute moisture content of the ambient air that is sampled. For control purposes, the filter temperature and RH are measured directly downstream of the filter because this ensures non-desiccating conditions at the filter. The VBCS is controlled through a combination of manual operation for setting flows and conditions to achieve the targeted 7°C and 85% RH. A computer automated readout and recording of the conditions (i.e., temperature and RH) are made at various locations throughout the instrument.
3. Methods
3.1. Polyurethane (PU) nanofiber filter media
3.1.1. Rationale and preparation
PU foams have been shown to provide favorable surfaces for collecting or maintaining microbes compared with Teflon™ (PTFE) and other filters (Crook et al. Citation1997; Flemming et al. Citation2000; Sharma et al. Citation2015; van Groenestijn and Liu Citation2002). A correctly designed nanofiber filter offers high collection efficiency, low pressure drops, and robustness (Bao et al. Citation2016; Barhate and Ramakrishna Citation2007; Choi et al. Citation2014; Ensor et al. Citation2010; Leung et al. Citation2010; Wang et al. Citation2008). We selected a PU nanofiber filter to provide a favorable surface for viability maintenance along with high collection efficiency and low-pressure drop.
We prepared the PU nanofiber filter media by electrospinning (Barhate and Ramakrishna Citation2007; Bhardwaj and Kundu Citation2010; Filatov et al. Citation2007; Persano et al. Citation2013; Reneker et al. Citation2007; Teo and Ramakrishna Citation2006). We used an in-house, custom-built apparatus to electrospin Pellethane 2103–90 AE Nat polyurethane (Lubrizol; Wickliffe, OH, USA) onto a microfiber nonwoven substrate. The nonwoven substrate provides a support to aid in handling and robustness. Details of the nanofiber media preparation are provided in the SI. Beaded fibers were generated with the average fiber diameter of 126 ± 42 nm and bead diameters of 2.3 ± 0.4 µm (see Figure S1 in the SI).
To mitigate concerns for filter contamination prior to use, filters were prepared in a clean laboratory by using tools and equipment sterilized with 70% isopropyl alcohol. Batch verification of finished filter products was performed by culturing filter extracts on trypticase soy agar (TSA) plates for 24 h.
3.1.2. Filter cassette
The electrospun, several-micron-thick layer of PU nanofibers on the nonwoven substrate were converted into 25 mm diameter filters by using a hammer-driven hole punch (McMaster-Carr; Chicago, IL, USA). The filters were placed into a 25-mm, asbestos PCM air sampling cassette (Zefon; Ocala, FL, USA) with a thin, paper gasket to ensure a tight seal (see Figure S2 in the SI). The support pad made from thick paper that is typically used with the PCM air sampling cassette was omitted in our filter cassettes. The ends of the cassette were plugged to ensure clean storage before use. During collection, the cassette was used in the open-face mode with the end caps removed.
3.1.3. Filter extraction to recover collected microbes
For filter extraction to recover collected microbes, we used a solution of FTA hemagglutination buffer (FTAB) with Tween-80 buffer. We combined 4.5 g of FTAB (BD Difco; Franklin Lakes, NJ, USA) and 0.5 mL Tween-80 (J.T. Baker; Center Valley, PA, USA) in 500 mL of deionized water and mixed well. This solution was autoclaved and allowed to cool. The solution was kept at 3°C and was used cold when extracting the filters. During a previous study (data not shown), we determined that this extraction buffer had minimal interference while conducting PCR. Although PCR was not a part of this present study, one objective of the VBCS is a system that does not preclude the use of any analytical techniques that might be needed to study the collected microbes.
We used sterilized tweezers and aseptic procedures to carefully extract the PU nanofiber filter from the cassette. To extract the microbes, we then place the filter into a 15-mL conical tube with 10 mL of extraction buffer. The capped conical tube was vortexed for 60 s while ensuring that the filter was immersed the entire time. The extraction liquid was then pipetted onto culture plates for bacteria colony counting.
3.2. Physical collection efficiency testing
The efficiency for particle collection by the nanofiber filters was determined by using aerosolized ammonium fluorescein. Particle-size distributions were measured both upstream and downstream of the filters, from which we inferred the size-dependent particle collection. Measurements were made by using an aerodynamic particle sizer (APS, TSI Model 3321; Shoreview, MN, USA) to span the particle size range from 0.7 to 10 µm. A high sensitivity optical spectrometer (Model UHAS, Droplet Measurement Technologies Inc.; Boulder, CO, USA) was also used for particles ranging from 0.06 to 1.0 µm. Filtration efficiency was measured by using filters mounted within their 25-mm cassettes. Additionally, the filters were assayed, and the collection was compared to a parallel collection with a commercial membrane filter (MiliporeIsopore, 0.8 µm ATTP). APS data were collected from two experiments with eight measurements upstream and eight measurements downstream of the filter cassette. The Model UHAS optical spectrometer data were collected from one experiment with two measurements upstream and three measurements downstream. The upper and lower confidence limits were determined via Poisson statistics by using IES-RP-CC007.1 (IES Citation1997).
The efficiencies for the particle transport from the inlet, through the temperature and RH conditioning systems, and to the filter cassette were measured with a polydisperse potassium chloride (KCl) aerosol (dried), the Climet particle counter, and the bio-test rig described in Section 3.4 of this manuscript. The aerosol concentration in the test rig (i.e., upstream of the VBCS) and immediately downstream of the filter cassette with no collection filter installed, provided an estimate of the particle losses in the VBCS. A single experiment was conducted for each of the conditions (i.e., no filter in cassette and collection filter in cassette). The total particle concentration in the test rig varied by less than 5%. The upstream concentrations were measured (5 times), and then the downstream concentrations were measured (7 and 12 times, respectively). An additional three measurements upstream were made to verify that no change in the aerosol challenge concentration occurred. Upper and lower confidence limits were determined via Poisson statistics by using IES-RP-CC007.1 (IES Citation1997).
3.3. Test microbe and preparation of bioaerosol
We selected Escherichia coli (ATCC 15597; Manassas, VA, USA) as the test microbe for many reasons. E. coli is a vegetative bacterium that is readily aerosolized, it easy to work with (biosafety level [BSL]−1 for the strain selected and culturable counts are obtained with overnight growth), it is well studied as a bioaerosol (Cox Citation1968; Crook et al. Citation1997; King and McFarland Citation2012; Li et al. Citation1999; Wathes et al. Citation1986; Zhen et al. Citation2014), and it is more vulnerable to inactivation in an aerosolized state than a bacterial spore (e.g., Bacillus) or fungal spores.
E. coli was used to inoculate 50 mL of nutrient broth (BD Difco; Franklin Lakes, NJ, USA) and was incubated overnight at 37°C in a shaking incubator (200 rpms). For Collison preparations, 20 mL of the overnight culture was added to 30 mL of sterile distilled water in a six-jet Collison nebulizer (BGI by Mesa Labs; Butler, NJ, USA). This preparation resulted in a nebulizing solution of 40% nutrient broth and a Collison concentration between 4 × 108 and 8 × 108 colony forming units (CFU)/mL of culturable E. coli. The broth was included to provide a small amount of protective factors to the E. coli during the stress of aerosolization and drying to particles.
3.4. Test rig
For this indoor air study, a single-pass test duct was used that provided aerosol drying and steady-state airflow with sampling ports for bioaerosol collection. We selected a controlled test duct for this study to provide careful characterization of the VBCS rather than convolute a study of room air dynamics and characterization of the VBCS. The test duct is compliant with ASHRAE 52.2 and 185.1 test standards (ASHRAE Citation2012; Citation2015). A schematic diagram of the test duct is shown in , with a cross-section of 0.61 m × 0.61 m. Uniformly mixed bioaerosol is provided to the test section of the duct. The test duct uniformity and the rapid increase and then decrease of introduced aerosol in the test rig were validated using an array of six AGI-4-mm (AGI-4; Ace Glass; Vineland, NJ, USA) samplers and a Climet CI-500 (Climet Instruments; Redlands, CA, USA) particle counter.
At the aerosol generator site, the Collison nebulizer containing E. coli was attached to the test duct, and aerosol was generated by using 15 psi of air. This aerosol was mixed with 85 L/min of clean, dry air in a drying tower. The aerosol was then passed through a 10 mCi Kr85 charge neutralizer before mixing with the test duct air, providing an airflow in the test duct of 8,000 L/min and a culturable bioaerosol concentration of 4 ± 2 × 103 CFUs/L of air.
3.5. Test protocol and reference sampler
The VBCS was tested with aerosolized E. coli by using the protocol shown in . The time between stopping of the bioaerosol challenge and removal of the filter was used to simulate the situation where an incident occurs early in the VBCS sampling cycle. An AGI-4 was selected as a reference sampler and operated only when the bioaerosol challenge was released into the testing apparatus to provide a count of the total concentration of culturable bioaerosol present. For sampling times of 30 min and less, the AGI-4 has high physical collection efficiency for 0.3–5 µm–sized particles (Lin et al. Citation1997), high-culturability collection efficiency, and does not require an extraction step before plating for culturability (Lehtimaki and Willeke Citation1993). We used a bioaerosol challenge time and AGI-4 sampling time of 30 min to minimize transient effects of startup and shutdown of the aerosol challenge and reference sample and to provide sufficient culturable E. coli. By using the phrase “sufficient culturable bacteria,” we mean, provide sufficient signal (i.e., culturable counts) to effectively measure relative impacts of each process step compared to experimental variability (i.e., noise). To study the VBCS's effect on bacteria culturability, the sampling time of the system was varied, and cool storage for 24 h (laboratory refrigerator at approximately 3°C) was included in some of the tests.
Prior to beginning an aerosol test, the VBCS was allowed to come to steady-state filter temperature and RH, with the test duct running and no bioaerosol present. To begin an experiment, the Collison nebulizer and the AGI-4 pump (12.5 L/min) were started. After 30 min of the bioaerosol challenge, both the AGI-4 and Collison were turned off, removed from the test duct, and placed on ice for plating to obtain culturable counts later that same day. The VBCS was either turned off at the 30-min mark or was allowed to continue sampling after the bioaerosol was turned off. For each experimental run, one AGI sample and one VBCS filter were collected. For each experimental condition, three to eight experiments were conducted.
3.6. Culturable bacteria counting and analysis of data
Viability of the aerosolized E. coli was determined by and restricted to culturable counts as assayed via standard microbiology techniques. Collected AGI-4 samples were measured to determine final volume of impinger fluid, and then were serially diluted before plating. PU nanofiber filter samplers were extracted. The extraction liquid volume was measured and, if needed, was serially diluted before plating. The AGI-4 impinger fluid or filter extract was plated in triplicate onto TSA (BD Difco; Franklin Lakes, NJ, USA) plates by using 0.1 mL of fluid for each sample. The TSA plates were incubated at 37°C for 24 h and counted for CFUs.
Calculations to determine culturable bioaerosol recovered were performed as follows: the CFUs/mL were calculated by using the sum of the triplicate plates, the total volume plated (0.3 mL), and any corrections for dilution. The total CFU collected by the sampler (i.e., AGI-4 or VBCS) was determined from the CFUs/mL multiplied by the total liquid collection volume (i.e., total volume of impinger fluid or total volume of extraction liquid). From the measure of CFUs/mL in the collected liquid sample, the airborne concentration of culturable microbes in the test duct measured by each sampler was then calculated. The air sampling flow rate (Q, L/min) and the bioaerosol challenge time (t, 30 min) were used. Only the bioaerosol release time (i.e., when bioaerosol was in the test duct) is used for both samplers, although the VBCS may have been operated for much longer times when the bioaerosol challenge was no longer present in the test duct. The CFUs/volume of air sampled was thus obtained with Equation (Equation1[1] ).
[1]
This calculated CFU/L air for each sampler is the observed culturable bioaerosol concentration for that sample. As previously mentioned, we assumed that the AGI-4 is a good reference and provides an accurate estimate of the culturable, aerosolized E. coli in the test duct. Results for the VBCS are reported as a percentage relative to the AGI-4 as given by Equation (Equation2[2] ).
[2]
The VBCS observed CFU/L air is a convolution of the culturable collection efficiency, culturability maintenance during sampling after the aerosol was turned off (if applicable to the specific test), the culturability maintenance during storage (if applicable to the specific test), and the extraction efficiency of culturable bacteria. This ratio of the VBCS to AGI provides an estimate of the total efficiency of the VBCS to collect and maintain the culturability of the bacteria.
4. Results and discussion
4.1. PU nanofiber filter
The low-pressure drop of our nanofiber filter media is demonstrated in and compared with two common filter materials used in air sampling, Teflon and gelatin. Teflon filters are good for the collection of inert particles, whereas gelatin filters are commonly used in short-term filtration sampling of bioaerosols. During our studies, we found that a decrease in RH occurs across the fibrous filter (i.e., upstream RH compared to downstream RH) due to the pressure drop (see the SI). This finding is because the temperature is constant (i.e., a thermodynamically open system occurs instead of adiabatic process), which holds the saturation pressure constant while the partial pressure of water decreases, hence the RH drops (Felder and Rousseau Citation1986). Potentially very high-pressure gradients could result in desiccating conditions for the collected microbes. (We did not investigate this possibility of high-pressure drop desiccating the bacteria.) Although the nanofiber filter has a low initial pressure drop, it was demonstrated to be very robust withstanding loading with Arizona Test Dust and ambient aerosol to pressure drops greater than 25 kPa. For a clean PU nanofiber filter in the 25-mm filter cassette with a flow rate of 25 L/min, the face velocity was approximately 5,100 cm/min, and the pressure drop was approximately 7 kPa.
Figure 3. Pressure drop as a function of filter face velocity for two common sampling filters and the RTI International's PU nanofiber filter in a 25-mm sampling cassette. Data points are an average of three measurements with the standard deviation in the measurements being the size of the symbols shown on the graph. Lines are to guide the eyes and are not fits or models.
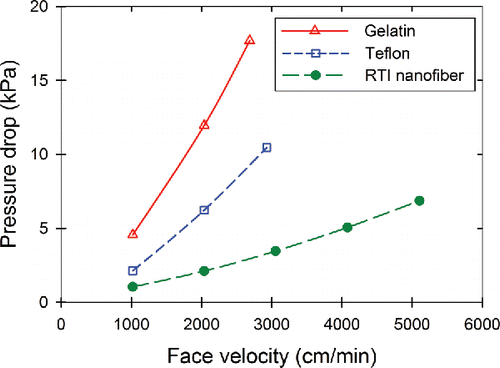
The physical collection efficiency (i.e., the particle collection without regard to culturability) was determined by using inert particles. Inferred size-dependent collection efficiency for 25 L/min flow passing through the 25-mm holder with the nanofiber filter is shown in . These data show 90% or higher collection efficiency over the entire tested size range (i.e., 0.06–9 µm) and better than 95% for the range of 0.1–9 µm. Direct assay of the filters confirmed the collection, with the nanofiber filters yielding slightly higher fluorescence readings than the parallel reference filter. Insufficient counts in the larger particle sizes provide poor count statistics above approximately 5 µm.
4.2. Particle transport
The total physical efficiency of particle transport through the VBCS was measured and is illustrated in . For the size range of 0.3 µm to 5.5 µm (i.e., the size range for statistically significant counts obtained in the test rig for the KCl aerosol used in this experiment), the losses were less than 10% below 3 µm and less than 20% below 6 µm. The losses were most likely due to the transition from the first-stage Nafion exchange unit to the second-stage Nafion exchange unit. During particle transport tests with a single Nafion unit, losses of much less than 5% were observed for particles less than 6 µm (Figure S4). The large particles were likely being lost due to the individual tubes at the exit of the first unit not being aligned with the inlet of the tubes in the second unit. A different design that takes this issue into consideration could potentially reduce the large particle losses.
Figure 5. Particle transport. Observed particle losses and collection by system (measurement of aerosol concentration in the test rig compared with downstream of the filter holder with and without the collection filter installed). Data shown are the mean of particle counts downstream to the mean of counts upstream, with upper and lower confidence limits calculated via Poisson statistics.
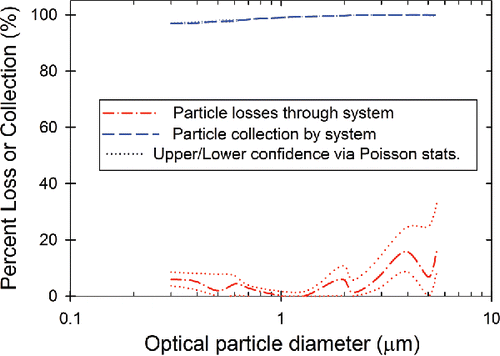
4.3. Two-stage Nafion system for cool and humid collection conditions
We used an environmental chamber to test the ability of the VBCS to hold the sampling filter at stable, cool, and humid conditions, despite fluctuating environmental conditions. The VBCS was allowed to come to a steady state with a target of approximately 7°C and 85% RH. The conditions in the environmental chamber were then varied to provide transient inlet conditions while monitoring changes in conditions at the filter. shows the sampler inlet and filter conditions for this test of response to transient conditions. The sampler inlet temperature and RH indicate the conditions of the ambient air being sampled. The filter T and RH were held stable within a window appropriate for maintaining microbe culturability. A wider range of steady-state inlet conditions and resulting conditions at the filter were tested (see for a summary). Although the inlet conditions changed conditions at the filter, the filter conditions were typically in our target range of T ≤ 10°C and an RH between 80% and 95%. A hot and dry inlet condition made it difficult to achieve an RH of 80% at the filter. Modification of the pressure and flow in the second-stage Nafion jacket could have remedied this problem. These stable T and RH results demonstrate that the VBCS can tolerate changes in the environment while effectively maintaining conditions at the filter favorable for microbe culturability maintenance.
Figure 6. Readout of filter and inlet environmental conditions for experiment of varying inlet conditions demonstrating the stability of conditions at the filter for transient inlet conditions. Data are from a single continuous experiment. Other experiments showed similar results. Due to the transient nature of the experiment, experimental uncertainty was not determined.
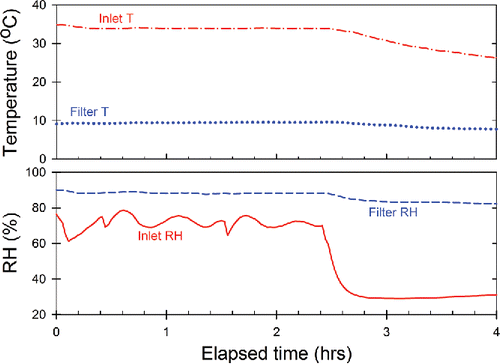
Table 1. Resulting filter conditions for different, steady-state inlet conditions for the VBCS. Data are from single experiments where values reported are the results after at least 30 min of consistent readings (coefficient of variation <1%) at each test condition.
A test of instrument operational stability and simplicity of startup was conducted (more details are presented in the SI). The VBCS was demonstrated to run for at least 2 weeks (the test was stopped for time constraints, not due to issues) while maintaining target conditions at the filter. The only maintenance needed was the changing of the sampling filters.
4.4. Indoor culturable bioaerosol sampling
The results of testing the VBCS with aerosolized E. coli under indoor conditions are shown in . The graph reports the culturable bacteria recovered from the nanofiber filter relative to the AGI-measured culturable bacteria. In all cases, the aerosolized E. coli occurred during the first 30 min of sampling. For the samples collected on the VBCS filter that were retrieved immediately after exposure to the E. coli, the recovery compared to the AGI was 23 ± 13%. However, when sampling continued for an additional 4.5 h after the bioaerosol exposure, with clean, T- and RH-conditioned air passing through the VBCS filter, the relative recovery of E. coli did not change (22 ± 18%). An exponential decay in culturability was observed from approximately 8 h to 15 h of sampling time. Some culturable bacteria were still present after 15 h of sampling. We did not test sampling times longer than 15 h.
Figure 7. Sampling of aerosolized E. coli with the VBCS. Data points are an average of 3 to 8 experiments, and error bars are the standard deviation. Bioaerosol was supplied during only the first 30 min of sampling. The relative culturability recovery is with respect to the AGI-measured, total aerosolized culturable bacteria present in the first 30 min.
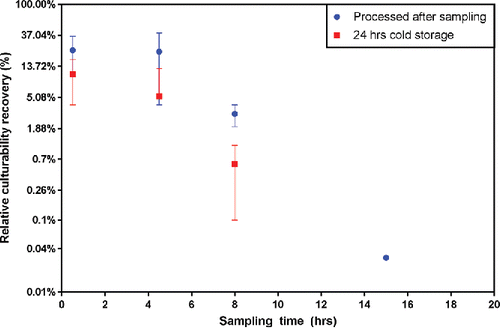
The source of the losses during the initial collection relative to the AGI were not determined. However, potential sources of bacteria loss include the following: physical losses in the T- and RH-conditioning process, unexpected stresses (e.g., desiccation) placed on the microbes during the conditioning process, the stress of the collection mechanisms of interception and impaction onto the filter fibers, and/or problems with extraction from the nanofiber filter. An intriguing possibility is the known losses of particles greater than 4 µm (). Although the majority of the particles were below 2 µm (Figure S5), any larger particles will actually contain a large number of bacteria, so, although by physical count, most of the particles were below 2 µm, the majority of culturable bacteria were likely in the very few particles that were greater than 2 µm. A detailed study of bacteria culturability count by particle size fraction would be needed to determine whether the low, initial collection in culturable bacteria was due to the physical losses of the larger particles in the transition from the first-stage exchange unit to the second stage. Re-engineering of the system to minimize particle losses would reduce this problem.
Although recovery of culturable E. coli from the initial collection was low, the important result is that after collection onto the filter, the culturability of the vegetative bacterium was maintained. Using the culturable E. coli recovered on the filter samples challenged for 30 min as the reference, that culturability maintenance of the collected bacteria was 98% at 4.5 h. The expectation with a convective airflow would be considerable desiccation of the bacteria, but this was not observed within the first 4.5 h of air sampling. The cause of the exponential decay in culturability after 4.5 h was not determined, but could include desiccation or death of the bacteria resulting from attempted growth in nutrient poor conditions. Detailed molecular and bacteria morphology studies would be needed to understand the details. However, the potential of the VBCS is demonstrated in the fact that a vegetative bacterium was maintained on a dry filter while being stressed by a convective airflow for several hours.
Cold storage of filters after removal from the VBCS reduced culturability, but still provided meaningful, countable colonies when sample collection was 5 h or less. Substantial decay of culturability with storage was observed for 8-h samples. The 15-h samples were not subjected to cold storage. Ideally, an instrument could be developed with an automatic filter changer that would start a new filter approximately every 5 h, thus providing long-term samples with preserved, culturable bacteria present.
The VBCS was tested with a vegetative bacterium. Hardier microbes (e.g., spores) that are more commonly found in an aerosolized state could potentially last 12–24 h on the sampler. Further testing will be needed to prove culturability maintenance of hardier microbes.
5. Conclusions
The purpose of this study was to determine whether temperature- and RH-controlled filtration can preserve vegetative bacteria culturability during long sampling times (4 h and longer). We describe the VBCS, a 25 L/min filter sampling system based on a PU nanofiber filter and conditioning of the sampled air by using a system of two-stage Nafion exchange units. The sampled air was conditioned to a temperature ≤10°C and an RH in the range of 80–95%. The VBCS was tested with aerosolized E. coli under indoor conditions. An AGI-4 was used as a reference sampler, and the VBCS total observed culturable collection efficiency (convolution of sampling, culturability maintenance during continued air sampling, culturability maintenance during cold storage, and extraction efficiency) was judged against the reference sampler.
Key elements in the design and the process of sampling for culturable bioaerosol along with results for the VBCS include the following:
Efficient particle transport to the collection point
Particle losses were <20% below 6 µm and <10% below 3 µm.
Efficient particle collection
Particle collection was >95% for sizes 0.1 µm to 10 µm, when considering those particles that make it to the filter, and >95% for sizes 0.1 µm to 3 µm, when considering the particles drawn into the inlet of the VBCS.
Maintain bioaerosol culturability during collection
During the first 5 hours of sampling, culturability was fairly independent of sampling time.
Maintain culturability during sample storage
For samples collected during the first 5 h, cold storage decreased bacteria culturability, but still provided significant counts.
Stable conditions at the collection point
Despite variations in inlet conditions, T and RH at the collection filter were maintained; stable operation can be maintained with minimal maintenance for weeks.
The VBCS suffered an initial loss of culturable microbes compared to the AGI sampler. For initial processing of filter samples, only 23% of the culturable microbes were recovered; however, once collected, the culturability of 98% of collected microbes was maintained for up to 4.5 h. It is postulated that the low, initial culturable collection efficiency was partially due to physical losses of large particles, which contained a larger fraction of the culturable bacteria, at the transition from the first- to the second-stage Nafion humidity exchange units. Using continuous Nafion tubes, or aligning inlets and outlets of the individual tubes, to transition from the first-stage to the second-stage air conditioning could minimize these particle losses and improve culturability collection.
The VBCS provides longer-term (≤5 h) culturable sampling of vegetative E. coli than is possible with traditional dry filter sampling. Because culturability was the method used for these initial studies, only a subset of viable bacteria was measured in the filter collection potentially underrepresenting the total number of viable E. coli present. Therefore, the overall performance of the VBCS may be better than what was represented by the culturable counts. To fully determine the performance of the samplers, an assessment of the total viability, including the viable non-culturable and the active but non-culturable E. coli, could be measured by using alternative methods such as live/dead staining, viable PCR, or qRT-PCR (quantitative reverse transcriptase PCR) of the messenger RNA (Keer and Birch Citation2003; Kell et al. Citation1998). Additionally, the sampling of hardier aerosolized microorganisms would show even better results compared to E. coli, which is a vulnerable, vegetative bacterium.
Because the VBCS can operate for several hours, the system provides an alternative to traditional liquid-based samples (e.g., AGI) when longer-term sampling is needed. The VBCS provides a 25 L/min flow rate, thus providing a larger sample of the air than low-flow rate (e.g., 1 L/min) liquid-based samples designed to run for longer than 1 hour. From a convenience perspective, the VBCS provides a dry filter in a standard air sampling cassette. Use of a dry filter cassette provides a ready means to transport and store samples without the need to handle special liquids in the field. This filter is easily extracted with a simple buffer, which is compatible with live culture and other analytical techniques such as PCR. The only consumables for the VBCS used at the sampling location are the sampling cassettes and purified water (standard bottled water is sufficient). The VBCS shows promise as a bioaerosol sampler whose ability to preserve bacterial culturability under a range of environmental conditions would appreciably improve public health monitoring.
UAST_1286290_Supplemental_File.zip
Download Zip (607.2 KB)Acknowledgments
The authors greatly appreciate the contributions of Jeffery Portzer, J. Randle Newsome, Roger H. Pope, Sarah D. Shepherd, and Adam Rieth for fabricating the VBCS. We also appreciate the assistance of C. Spencer West, Amy Evans, and Karin Foarde with the bioaerosol experiments.
The authors thank Dr. Christina Murata and Mr. James Hanley for the fruitful conversations and editorial comments in preparing this manuscript.
Funding
This work was supported by the U.S. Department of Homeland Security, Science and Technology Directorate under contract HSHQDC-09-C-00154.
References
- ASHRAE. (2012). Standard 52.2: Method of Testing General Ventilation Air-Cleaning Devices for Removal Efficiency by Particle Size American Society of Heating. Refrigerating, and Air-Conditioning Engineers, Atlanta, GA.
- ASHRAE. (2015). Standard 185.1: Method of Testing UVC Lights for Use in Air Handling Units or Air Ducts to Inactivate Airborne Microorganisms, American Society of Heating. Refrigerating, and Air-Conditioning Engineers, Atlanta, GA.
- Bao, L., Seki, K., Niinuma, H., Otani, Y., Balgis, R., Ogi, T., Gradon, L., and Okuyama, K. (2016). Verification of Slip Flow in Nanofiber Filter Media Through Pressure Drop Measurement at Low-Pressure Conditions. Sep. Purif. Technol., 159:100–107.
- Barer, M. R., and Harwood, C. R. (1999). Bacterial Viability and Culturability. Adv. Microb. Physiol., 41:93–137.
- Barhate, R. S., and Ramakrishna, S. (2007). NanofibrousFiltering Media: Filtration Problems and Solutions From Tiny Materials. J. Membr. Sci., 296:1–8.
- Bhardwaj, N., and Kundu, S. C. (2010). Electrospinning: A Fascinating Fiber Fabrication Technique. Biotechnol. Adv., 28:325–347.
- Bowers, R. M., McLetchie, S., Knight, R., and Fierer, N. (2011). Spatial Variability in Airborne Bacterial Communities Across Land-Use Types and Their Relationship to The Bacterial Communities of Potential Source Environments. ISME J., 5:601–612.
- Burge, H. A., ed. (1995). Bioaerosols. Lewis Publishers, Boca Raton, FL.
- Burrows, S. M., Elbert, W., Lawrence, M. G., and Poeschl, U. (2009). Bacteria in the Global Atmosphere—Part 1: Review and Synthesis of Literature Data for Different Ecosystems. Atmos. Chem. Phys., 9:9263–9280.
- Caruana, D. J. (2011). Detection and Analysis of Airborne Particles of Biological Origin: Present and Future. Analyst, 136:4641–4652.
- Choi, H. J., Kim, S. B., Kim, S. H., and Lee, M. H. (2014). Preparation of Electrospun Polyurethane Filter Media and Their Collection Mechanisms for Ultrafine Particles. J. Air Waste Manage. Assoc., 64:322–329.
- Cox, C. S. (1968). Aerosol Survival and Cause of Death of Escherichia coli K12. J. Gen. Microbiol., 54:169–175.
- Crook, B., Kenny, L. C., Stagg, S., Stancliffe, J. D., Futter, S. J., Griffiths, W. D., and Stewart, I. W. (1997). Assessment of the Suitability of Different Substrate Materials for Bioaerosol Sampling. Ann. Occup. Hyg., 41:647–652.
- Despres, V. R., Huffman, J. A., Burrows, S. M., Hoose, C., Safatov, A. S., Buryak, G., Froehlich-Nowoisky, J., Elbert, W., Andreae, M. O., Poeschl, U., and Jaenicke, R. (2012). Primary Biological Aerosol Particles in the Atmosphere: A Review. Tellus: Series B—Chem. Phys.Meteorol., 64: 15598.
- Douwes, J., Thorne, P., Pearce, N., and Heederik, D. (2003). Bioaerosol Health Effects and Exposure Assessment: Progress and Prospects. Ann. Occup. Hyg., 47:187–200.
- Ensor, D. S., Walls, H. J., Andrady, A., and Walker, T. A. (2010). Particle filter system incorporating nanofibers. U.S. Patent No. 7,789,930.
- Felder, R. M., and Rousseau, R. W. (1986). Elementary Principles of Chemical Processes. John Wiley and Sons, New York. Chapters 6 and 7.
- Filatov, Y., Budyka, A., and Kirichenko, V. (2007). Electrospinning of Micro- and Nanofibers: Fundamental and Applications in Separation and Filtration Processes. Begell House, Inc., Redding, CT.
- Flemming, R. G., Capelli, C. C., Cooper, S. L., and Proctor, R. A. (2000). Bacterial Colonization of Functionalized Polyurethanes. Biomaterials, 21:273–281.
- Ghosh, B., Lal, H., and Srivastava, A. (2015). Review of Bioaerosolsin Indoor Environment with Special Reference to Sampling, Analysis and Control Mechanisms. Environ. Int., 85:254–272.
- Griffiths, W. D., and Decosemo, G. A. L. (1994). The Assessment of Bioaerosols—A Critical Review. J. Aerosol Sci., 25:1425–1458.
- IES. (1997). IES-RP-CC007.1: Testing of ULPA Filters. Institute of Environmental Sciences and Technology, Arlington Heights. pp.23–24.
- Incropera, F. P., and DeWitt, D. P. (1990). Chapter 11, Heat Exchangers, in Fundamental of Heat and Mass Transfer. John Wiley and Sons, New York. pp. 640–693.
- Kango, N. (2010). Textbook of Microbiology. I. K. International Pvt. Ltd., New Delhi.
- Karwowska, E., Sztompka, E., Lebkowska, M., and Miaskiewicz-Peska, E. (2003). Viability of Bacteria in Fiber Filters as A Result of Filter Humidity. Polish J. Environ. Stud., 12:57–61.
- Keer, J. T., and Birch, L. (2003). Molecular Methods for the Assessment of Bacterial Viability. J. Microbiol. Meth., 53:175–183.
- Kell, D. B., Kaprelyants, A. S., Weichart, D. H., Harwood, C. R., and Barer, M. R. (1998). Viability and Activity in Readily Culturable Bacteria: A Review and Discussion of the Practical Issues. Antonie Van Leeuwenhoek, 73:169–187.
- Kesavan, J., and Sagripanti, J. L. (2015). Evaluation Criteria for Bioaerosol Samplers. Environ. Sci.—Processes Impacts, 17:638–645.
- King, M. D., and McFarland, A. R. (2012). Bioaerosol Sampling with a Wetted Wall Cyclone: Cell Culturability and DNA Integrity of Escherichia coli Bacteria. Aerosol Sci. Technol., 46:82–93.
- La Rosa, G., Fratini, M., Della Libera, S., Iaconelli, M., and Muscillo, M. (2013). Viral Infections Acquired Indoors Through Airborne, Droplet or Contact Transmission. Annalidell'IstitutoSuperiore de Sanita, 49:124–132.
- Lednicky, J., Pan, M. H., Loeb, J., Hsieh, H., Eiguren-Fernandez, A., Hering, S., Fan, Z. H., and Wu, C. Y. (2016). Highly Efficient Collection of Infectious Pandemic Influenza H1N1 virus (2009) Through Laminar-Flow Water Based Condensation. Aerosol Sci. Technol., 50:I–IV.
- Lehtimaki, M., and Willeke, K. (1993). Measurment Methods, in Aerosol Measurement, K. Willeke and P. A. Baron, eds. Van Norstrand Reinhold, New York. pp. 112–129.
- Leung, W. W. F., Hung, C. H., and Yuen, P. T. (2010). Effect of Face Velocity, Nanofiber Packing Density and Thickness on Filtration Performance of Filters with Nanofibers Coated on a Substrate. Sep. Purif. Technol., 71:30–37.
- Li, C. S., Hao, M. L., Lin, W. H., Chang, C. W., and Wang, C. S. (1999). Evaluation of Microbial Samplers for Bacterial Microorganisms. Aerosol Sci. Technol., 30:100–108.
- Lighthart, B. (1997). The Ecology of Bacteria in the Alfresco Atmosphere. FEMS Microbiol. Ecol., 23:263–274.
- Lin, X. J., Willeke, K., Ulevicius, V., and Grinshpun, S. A. (1997). Effect of Sampling Time on the Collection Efficiency of All-Glass Impingers. Am. Indust. Hyg. Assoc. J., 58:480–488.
- Morawska, L., Afshari, A., Bae, G. N., Buonanno, G., Chao, C. Y. H., Hanninen, O., Hofmann, W., Isaxon, C., Jayaratne, E. R., Pasanen, P., Salthammer, T., Waring, M., and Wierzbicka, A. (2013). Indoor Aerosols: From Personal Exposure to Risk Assessment. Indoor Air, 23:462–487.
- Nazaroff, W. W. (2016). Indoor Bioaerosol Dynamics. Indoor Air, 26:61–78.
- Nevalainen, A., Willeke, K., Liebhaber, F., Pastuszka, J., Burge, H. A., and Henningson, E. W. (1993). Bioaerosol Sampling, in Aerosol Measurement, K. Wileke and P. A. Baron, eds. Van Nostrand Reinhold, New York. pp. 471–492.
- Persano, L., Camposeo, A., Tekmen, C., and Pisignano, D. (2013). Industrial Upscaling of Electrospinning and Applications of Polymer Nanofibers: A Review. Macromol. Mater. Eng., 298:504–520.
- Reneker, D. H., Yarin, A. L., Zussman, E., and Xu, H. (2007). Electrospinning of Nanofibersfrom Polymer Solutions and Melts, in Advances in Applied Mechanics, vol. 41, Elsevier Academic Press Inc., San Diego, CA. pp. 43–195.
- Sharma, A., Clark, E., McGlothlin, J. D., and Mittal, S. K. (2015). Efficiency of Airborne Sample Analysis Platform (ASAP) Bioaerosol Sampler for Pathogen Detection. Frontiers Microbiol., 6:512.
- Teo, W. E., and Ramakrishna, S. (2006). A Review on Electrospinning Design and Nanofibre Assemblies. Nanotechnology, 17:R89–R106.
- van Groenestijn, J. W., and Liu, J. X. (2002). Removal of Alpha-PineneFrom Gases Using Biofilters Containing Fungi. Atmos. Environ., 36:5501–5508.
- Walls, H. J., Ensor, D. S., Harvey, L. A., Kim, J. H., Chartier, R. T., Hering, S. V., Spielman, S. R., and Lewis, G. S. (2016). Generation and Sampling of Nanoscale Infectious Viral Aerosols. Aerosol Sci. Technol., 50:802–811.
- Walser, S. M., Gerstner, D. G., Brenner, B., Bunger, J., Eikmann, T., Janssen, B., Kolb, S., Kolk, A., Nowak, D., Raulf, M., Sagunski, H., Sedlmaier, N., Suchenwirth, R., Wiesmuller, G., Wollin, K. M., Tesseraux, I., and Herr, C. E. W. (2015). Evaluation of Exposure-Response Relationships for Health Effects of Microbial Bioaerosols—A Systematic Review. Int. J. Hyg. Environ. Health, 218:577–589.
- Wang, J., Kim, S. C., and Pui, D. Y. (2008). Investigation of the Figure of Merit for Filters with a Single Nanofiber Layer on a Substrate. J. Aerosol Sci., 39:323–334.
- Wang, Z., Reponen, T., Grinshpun, S. A., Gorny, R. L., and Willeke, K. (2001). Effect of Sampling Time and Air Humidity on the Bioefficiencyof Filter Samplers for Bioaerosol Collection. J. Aerosol Sci., 32:661–674.
- Wathes, C. M., Howard, K., and Webster, A. J. F. (1986). The Survival of Escherichia coli in an Aerosol at Air Temperatures of 15 and 30 Degrees C and a Range of Humidities. J. Hyg., 97:489–496.
- Xu, Z., Wu, Y., Shen, F., Chen, Q., Tan, M., and Yao, M. (2011). Bioaerosol Science, Technology, and Engineering: Past, Present, and Future. Aerosol Sci. Technol., 45:1337–1349.
- Zhen, H. J., Han, T. W., Fennell, D. E., and Mainelis, G. (2014). A Systematic Comparison of Four Bioaerosol Generators: Affect on Culturability and Cell Membrane Integrity when Aerosolizing Escherichia coli Bacteria. J. Aerosol Sci., 70:67–79.