ABSTRACT
The ability of atmospheric particles to absorb water has extensive climate, atmospheric chemistry, and health implications, and considerable effort has gone into determining relationships between particle composition and hygroscopicity. Parallel techniques, in which co-located composition and hygroscopicity measurements are combined to infer composition-hygroscopicity relationships, may not detect the influence of external mixtures. Previous in-line measurements have been limited to single-particle composition or a limited analyte range, and are often non-quantitative and/or offline. Here, we present for the first time in-series, online, quantitative hygroscopicity-composition measurements using a Brechtel Manufacturing, Inc. Hybrid Tandem Differential Mobility Analyzer and an Aerodyne High-Resolution Time-of-Flight Aerosol Mass Spectrometer. This technique is first verified using laboratory-generated external particle mixtures, then extended to ambient measurements at a seaside sampling side at the Hong Kong University of Science and Technology. The technique successfully separated laboratory-generated particles of differing hygroscopicities and showed promise for atmospheric particles, though high mass attenuation endemic to the HTDMA dual size selection limits application to environments with at least ∼14–41 μg/m3 of particulate mass, depending on composition.
Copyright © 2017 American Association for Aerosol Research
EDITOR:
Introduction
Interactions between atmospheric water and particles are various, with far-reaching climate, atmospheric chemistry, and health implications (IPCC Citation2014). The ability of particles to absorb water, which varies between particle types (McMurry et al. Citation1996), can affect the cloud albedo, extent, and lifetime, which can in turn influence atmospheric radiative balance, precipitation, and surface insolation (IPCC Citation2014). Heterogenous chemistry in droplets and wetted particles can form new aerosol mass, light-absorbing brown carbon compounds, and acidic atmospheric waters (Lim et al. Citation2010); these products can also contribute to nutrient deposition, visibility degradation, and other environmental effects (Levin et al. Citation2009; Benedict et al. Citation2013). Thus, a sophisticated understanding of particle hygroscopicity is relevant to a number of important scientific questions. The work presented herein seeks to directly measure the chemical composition of ambient particles with varying hygroscopic growth factors using a hybrid tandem differential mobility analyzer (HTDMA) in-line before an aerodyne high-resolution time-of-flight aerosol mass spectrometer (AMS).
Particle hygroscopicity can be expressed via particle growth factor (GF), which is the ratio of the particle diameter at a given RH to its dry diameter. The growth factor of a particle population can be measured using an HTDMA: a relatively monodisperse, dry particle population is selected with a first differential mobility analyzer, then humidified; the resultant humidified size distribution is characterized using a humidified differential mobility analyzer and condensation particle counter (CPC). This technique has been used to quantify the hygroscopicity of a variety of particles, including single- and multi-component laboratory-generated particles (McMurry et al. Citation1996; Chan and Chan Citation2005).
Ambient aerosols have also been characterized via HTDMA, which is often paired in the field with parallel bulk chemical composition measurements to explore relationships between composition and hygroscopicity; instruments used in parallel include aerosol mass spectrometers (Gysel et al. Citation2007), impactors (Pitchford and McMurry Citation1994), and filters (Malm et al. Citation2005). However, parallel (and especially bulk) composition measurements inherently fail to separate the hygroscopic contributions of externally and internally mixed populations, with added convolution from possible differences in size selection specificity and sampling artifacts between the HTDMA and the parallel instrument. Coupling an in-series electron microscope to an HTDMA solved this issue and provided hygroscopicity, composition, and morphology, but was offline and time consuming (McMurry et al. Citation1996). Recent advances include combinations of an HTDMA with an in-series aerosol time-of-flight mass spectrometer (AToFMS), which provides single-particle composition but is not quantitative in regard to component proportions or the ambient aerosol population (Herich et al. 2008; Wang et al. 2014). Other instruments used in series include the Single-Particle Laser Ablation ToF MS (SPLAT), which is sensitive but also qualitative (Zelenyuk et al. 2008), and the Single-Particle Soot Photometer (SP2), which illuminates the particle mixing state, but is limited in analyte range to black carbon (McMeeking et al. 2011). These measurements have been very useful in conjunction with the Zdanovskii–Stokes–Robinson (ZSR) method, allowing fair predictions of total particle hygroscopicity (GFm) as the sum of the hygroscopicities of each particle component (k) via the equation: , where
is the volume fraction (Zdanovskii Citation1948; Stokes and Robinson Citation1966; Kanakidou et al. Citation2005); this method assumes particle sphericity, lack of solute-solute interactions in the aerosol water, and ideal mixing behavior.
However, between limitations in the specificity and range of composition measurements and test-particle/dataset representativeness (e.g., laboratory generated particles, which do not replicate the complex ambient matrix especially in terms of organics), and often low quantitativeness and time resolution, it would be ideal to develop an integrated hygroscopicity-composition measurement method that is online, quantitative, and valid for a wider range of particle compositions. The Aerodyne High Resolution Time-of-Flight Aerosol Mass Spectrometer (HR-ToF-AMS) provides online measurements of the size and non-refractory composition of submicron particles with mass resolution capable of identifying organic fragment empirical formulas (though not organic parent molecules, due to fragmentation in the vaporization/ionization step; Decarlo et al. Citation2006). This work describes the development and testing of a new method of in-series HTDMA-AMS measurement capable of determining the hygroscopicity and non-refractory composition of submicron particles.
Methods
Externally mixed particle populations were generated using two atomizers (a TSI 3076 and an Cole-Palmer 74900 syringe pump into a Brechtel Manufacturing, Inc. [BMI] 9200 atomizer), each followed by a 24-inch stainless steel-housed diffusion dryer; RH after the dryers measured ≤10%. After drying, particle populations were mixed in a stainless steel BMI chamber (19″w × 26″l x ×8″h) before introduction to the analytical system (). The atomizers and mixing chamber were cleaned thoroughly whenever a new solution was used. Aqueous solutions of ammonium nitrate (AN), ammonium sulfate (AS), humic acid (to represent “Low Volatility-Oxygenated Organic Aerosol,” or LV-OOA), glyoxylic acid (as “Semi Volatile-OOA,” or SV-OOA), and polystyrene latex spheres (PSL) were used singly and in various internally and externally mixed combinations as described later. Constant mass flow of each particle type was ensured by using metering valves on the atomizer output; intermittent direct AMS sampling of the mixed particle populations (no intervening HTDMA) showed largely stable particle output, and scans with flow irregularities were omitted from analysis. During some experiments, fresh mosquito coil smoke (Dragon Brand, made primarily of wood pulp, as a proxy for BBOA) was introduced to the mixing chamber from an outdoor, semi-enclosed burn chamber, through which flow was maintained at ∼15 LPM. The experiments were conducted at the HKUST Air Quality Research Supersite (Li et al. Citation2015). During ambient-aerosol experiments, the ¼-inch stainless steel inlet protruded 10 cm from the laboratory side wall ∼1 m above the roof decking; a 24-inch Nafion dryer (PermaPure MD-110-24S4) followed immediately inside the wall, with all instruments inlets within ∼0.5 m of the dryer outlet and utilizing copper or stainless steel tubing.
Figure 1. Schematic of the particle generation and combined HTDMA-AMS systems. Dashed lines indicate alternate plumbing pathways for sampling of various particle populations as described in the text.
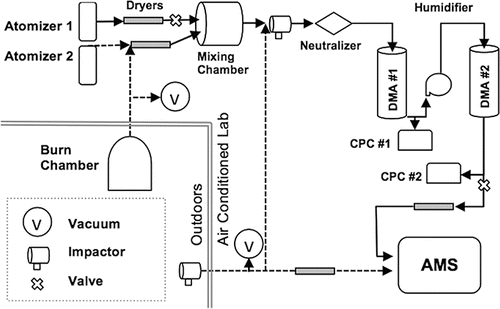
Briefly, the BMI Hybrid Tandem Differential Mobility Analyzer (HTDMA) dries and neutralizes the particle stream (using 85Kr), selects a subpopulation of mean geometric diameter D0 by electric mobility in the first DMA (DMA1), humidifies this subpopulation to a user-controlled RH (set at 90% for all experiments herein; Yeung et al. Citation2014), and then scans through electric mobility diameters again in a second, humidified DMA (DMA2). A butanol condensation particle counter (CPC) counts the particles during scanning, producing a number distribution of the humidified D0 population. The hygroscopic growth factor (GF), a common measure of hygroscopicity, can then be determined via GF = Dhumid/D0. This GF scan is reported as particle counts within a given particle-diameter bin (which, through the equation above, is also a growth-factor bin). The duration of sampling for each bin ranged from 20 to 120 s and the AMS run length was adjusted to match the sampling-bin length, giving a mass spectrum showing the average composition for each humidified size bin. This yields the composition and hygroscopicity of particles in each size bin. Details of the BMI HTDMA can be found in Lopez-Yglesias et al. (Citation2014). A bypass to the AMS was installed in the DMA2 sample excess line after the laminar flow element (LFE) and controlled by a two-way valve (); the BMI software adjusts the DMA2 sample excess valve to maintain a constant flow value through this LFE (with an ∼1-min stabilization period) whether the AMS bypass valve is open or closed. This AMS bypass included a 12-inch stainless steel Nafion dryer immediately after the bypass valve.
The HTDMA underwent extensive calibration, including DMA voltage calibrations, AS and PSL size distribution, humidified AS growth factor verifications, etc., as described in the manual (v2.6). Plumbing residence times within the HTDMA and between DMA2 and the AMS were measured using 250 nm AS after any changing plumbing or flow parameters.
Initial exploratory measurements of ambient aerosol using the tandem HTDMA-AMS yielded very low DMA2 particles counts (∼8/cc at the size distribution apex) and not enough mass to detect via AMS; given the dual size selection and line length through the HTDMA, significant mass loss through the system would be expected. Reductions in systemic mass loss involved a number of approaches. First, sample lines (outside the HTDMA) were shortened and sharp bends that might cause impaction were eliminated where possible. Line, mixing chamber, and calibration equipment materials were optimized for reduced static losses, using as much stainless steel and copper tubing as possible; polypropylene Nafion dryer sheaths were also replaced with stainless steel where possible.
HTDMA settings were also modified to reduce particle loss. Usually, DMA sample:sheath flow ratios are ‘‘tuned” to increase monodispersity; thus it stands to reason that ‘‘de-tuning” might broaden the selected size distribution and increasing the transmitted mass, albeit at the cost of size-selection precision. These relationships were explored by varying the sample:sheath flow ratio and observing the size distributions produced for ammonium sulfate, a well-constrained non-volatile species (). HTDMA size distribution width parameter (i.e., polydispersity) and total mass transmission increase with increasing DMA sample:sheath flow ratio. A sample:sheath flow ratio of 1 LPM:10 LPM was selected to balance increased particle transmission with size-selection precision.
Figure 2. Size distribution parameters (top) amplitude in units of dN/dlogDp and (bottom) geometric standard deviation of 150 nm ammonium sulfate particles using a 10 LPM sheath flow rate and varying sample flow rates. Data points represent the lognormal fit of a three-sample average, while error bars are the standard deviation of the lognormal fit.
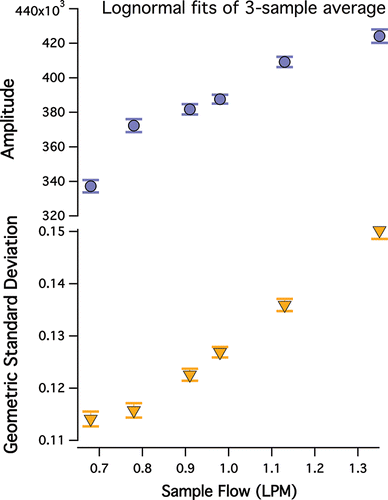
Results
Laboratory-generated standards
A variety of externally mixed particle populations of known composition and hygroscopicity were analyzed to validate this technique. It will be shown below that the chemical and hygroscopic properties of externally mixed particles are retained during the measurement process, with the exception of the nitrate and ammonium volatilization common to HTDMA measurements and discussed in more depth later.
The first external mixture involved polystyrene latex spheres vs. ammonium sulfate, as both are non-volatile and well-characterized in the literature. shows clear distinction in the hygroscopicity of these two particle types at 90% RH, with an organic mode from the PSLs at GF = 1.07 ± 0.00; PSLs are non-hygroscopic and should therefore have a growth factor of 1. For all replicates, the PSL modes produce mass spectra dominated by organic fragment m/z 104 (C8H8+, the polystyrene monomer) with successive mass spectral ‘‘peaks” separated by 13–14 m/zs reminiscent of methylene bridge subtraction during fragmentation (Figure S1). In , the second mode at GF = 1.72 ± 0.02 (D0 = 200 nm) is composed of ammonium and sulfate and is consistent with the spread in the literature, which includes multiple measurements of ∼1.7 at 90% RH (Wise et al. Citation2003; Lopez-Yglesias et al. Citation2014). The small contribution of organics in the AS mode may arise from organic contamination of the ammonium sulfate solution arising from the DI water generation system, which after much investigation was traced to the laboratory water supply, and comprises 12.1 ± 1.1% of mass. In the organic mass spectrum coinciding with the AS mode, the predominance of m/z 44 (CO2+) indicates a high degree of oxidation characteristic of low-volatility organics and yields oxidation-level indicator f44 = 0.20 (f44 = [mz44 mass]/[total organic mass]; Figure S2; Lanz et al. Citation2007; Ulbrich et al. Citation2009). According to a recent parameterization of f44 vs. growth factor, an organic with f44 = 0.20 could possess a GF > 1.7 at a water activity ≈ 0.95 (Duplissy et al. Citation2011); though mixing of this oxidized organic with the ammonium sulfate could contribute to the somewhat increased growth factor of the AS mode in comparison to many of the literature values stated above, the measured AS mode is within the 5% uncertainty commonly reported for HTDMA GF measurements (Swietlicki et al. Citation2008).
Figure 3. Number size distributions (dashed line, left axis) and mass concentration of total organics, sulfate, nitrate, and ammonium (solid colored lines, right axis) vs. hygroscopic growth factor for external mixtures of (a) 200 nm polystyrene latex spheres (PSL) vs. ammonium sulfate (D0 = 200 nm), and (b) an ammonium sulfate-humic acid mixture (see the text) vs. ammonium nitrate (D0 = 250 nm), all humidified to 90% RH.
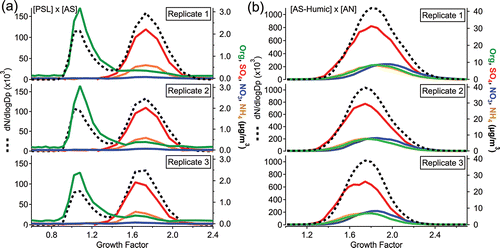
shows AS-humic acid particles (averaging 17.9% organic mass) externally mixed with AN in which both particle types have similar hygroscopicities, leading to an apparently unimodal growth factor distribution (Chan and Chan Citation2003). However, the composition data clearly indicate that two different particle populations contribute to the growth factor distributions, as the concentration distributions of AS-Organics and AN are shifted laterally from one another. As determined from the composition distributions, the AS-humic acid mixture has a growth factor mode of 1.74 ± 0.05 (determined using lognormal fits, since the number of bins limits data resolution in the x-dimension), while AN has a growth factor mode of 1.87 ± 0.06 (at 90% RH; standard deviations reflect variation between replicates). Ammonium nitrate growth factor measurements are highly complicated by the temperature-dependent effects of volatilization (Gysel et al. Citation2007); the literature indicates AN growth factors between ∼1.6 at 90% RH at 24.85 ± 2°C (Mikhailov et al. Citation2004) and ∼1.8 at 86% RH (D0 ≈ 180 nm; Hu et al. Citation2011).
Chamber burn experiments
The next group of experiments mixed mosquito-coil smoke from the outdoor burn chamber with internally mixed AN-AS-humic acid (13.0% organic, 66.4% sulfate, 4.8% nitrate by mass) particles meant to simulate aged SOA (); the varying relative magnitudes of the two modes may arise from dilution variation in the burn chamber due to wind, from which the chamber was sheltered but not sealed (there being small vents at the chamber bottom). Because the mosquito coils are comprised mostly of wood pulp, particles emitted by burning would be expected to display BBOA-like properties (Li et al. Citation2012); thus, these experiments would mimic a mixture of fresh BBOA and aged SOA, as often observed in ambient settings. Indeed, as shown in , the mosquito-coil smoke mode contains mass-spectral markers common to BBOA at m/zs 55, 57, 60, and 73 (Ng et al. Citation2011). Mosquito-coil smoke has a growth factor of 1.06 ± 0.04 at 90% RH, which is consistent with the literature, in which the hygroscopicity of fresh, burning-influenced particles ranges from GF = 1.07–1.09 for “[fresh] urban emission” (Aklilu et al. Citation2006) to GF = 1.22–1.30 for BBOA-influenced particles at Yosemite National Park (Carrico et al. Citation2005), and GF = 1.10 to 1.47 in Tanzania (Boreddy et al. Citation2014), all adjusted to 90% RH.
Figure 4. (left panel) Number size distributions (dashed line, left axis) and mass concentration of total organics, sulfate, nitrate, and ammonium (solid colored lines, right axis) vs. hygroscopic growth factor for external mixtures of mosquito-coil smoke vs. AN-AS-humic acid, and (right panel) mass spectra of each mode (averaged over the mode bin).
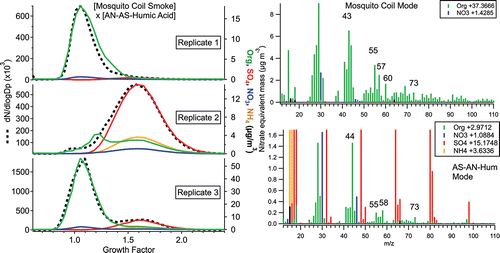
The second hygroscopic mode, comprised of AN-AS-humic acid particles, has a growth factor of 1.58 ± 0.03. The mode mass spectrum is dominated by ammonium, nitrate, sulfate, and organic fragments at m/zs 44, 55, and 58; m/z 44 is comprised of C2H4O+ (carbonyl) and/or CO2+ (carboxylic acid) fragments consistent with the highly oxygenated nature of humic acid (Lanz et al. Citation2007; Ulbrich et al. Citation2009). The organic mass spectrum of the AN-AS-humic acid mode differs from that of pure humic acid in this instrument in that it contains more mass at m/zs 55, 58, 69, etc. (Figure S3), but is also distinct from that of mosquito-coil smoke (lacking m/z 60), arguing against internal mixing of the two particle types; the enhanced complexity of the organic mass spectrum as compared with pure humic acid could arise from dark reaction with sulfate during particle generation, which may form organosulfate products (Hatch et al. Citation2011).
Lastly, mosquito-coil smoke was mixed externally with AN-glyoxylic acid particles (AN-Gly: 48.6% organic, 41.3% nitrate by mass; ). Mosquito-coil smoke mass spectra are fairly consistent between . The less-lognormal peak shapes—and slight increase in relative m/z 44—could arise from some internal mixing or coating between the smoke and AN-glyoxylic acid particles in the mixing chamber; this might also explain the slight increase in the growth factor of the mosquito-coil smoke to 1.14 ± 0.02, from the GF = 1.06 ± 0.04 measured in the mosquito-coil/AN-AS-humic acid experiments discussed previously. The second hygroscopic mode, comprised of AN-glyoxylic acid particles, has a growth factor of 1.60 ± 0.07, and a mass spectrum featuring prominent m/z 44, reflecting the carboxylic acid functionalization of glyoxylic acid and identical to the mass spectrum of the pure AN-glyoxylic acid particles (Figure S4). This is broadly consistent with the literature, as multifunctional organic acids have produced growth factors of 1.0−1.53 (Peng et al. Citation2001; Zamora et al. Citation2011), to which is added the enhanced hygroscopicity of ammonium nitrate.
Figure 5. (left panel) Number size distributions (dashed line, left axis) and mass concentration of total organics, sulfate, nitrate, and ammonium (solid colored lines, right axis) vs. hygroscopic growth factor for external mixtures of mosquito-coil smoke vs. AN-glyoxylic acid, and (right panel) mass spectra of each mode (averaged over the mode bin).
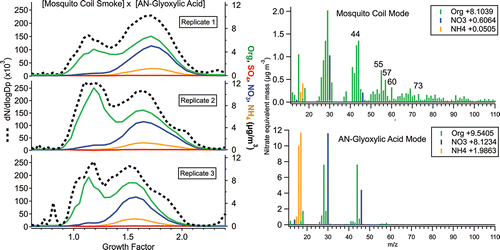
Ambient measurements
After successful characterization of the laboratory-generated particles described above, this technique was applied to ambient particles. To counter the high particle losses observed in the HTDMA using laboratory-generated particles (for which total mass transmission measured <1% for ammonium sulfate and humic acid particles, for example), we selected high-concentration ambient particle episodes (generally when PM2.5> ∼30 μg/m3 as measured by a co-located ThermoFisher Scientific TEOM Monitor). The PM1 ambient size distribution was determined using the HR-ToF-AMS (Aerodyne Research, Inc., Billerica, MA, USA), and the ambient mode was selected as the D0 in DMA1 of the HTDMA to maximize mass retention. The AMS-averaging and HTDMA bin time was increased to 60 s to reduced noise in the mass spectra with the possible caveat of changes in the ambient aerosol population over the relatively protracted scan time, there being 30–40 bins per scan.
shows scans from ambient aerosol events on 30 September and 14 October 2015 with growth factor modes of GF = 1.57 (D0 = 300 nm), 1.54 (D0 = 400 nm), and 1.42 (D0 = 400 nm) from top to bottom. Organics, sulfate, and, for the 14 October samples, nitrate exceed their respective AMS detection limits (dashed lines). Total mass transmission with the HTDMA in-line measured 1.8 ± 0.5% of the ambient concentration measured by in-series dryer, 85Kr neutralizer, and AMS, highlighting the severe mass loss in the HTDMA. Ammonium, which was more abundant by mass than nitrate in the ambient aerosol but also has a detection limit ten times higher, was not detected using this technique. Volatile loss of ammonium is also probable: given the mass transmission above for the bulk particle, no volatile loss, and an ammonium detection limit of 0.038 μg/m3 (Decarlo et al. Citation2006), ambient ammonium levels above 1.67 should be detectable; however, no ammonium was detected though ambient concentrations were 3.23, 2.14, and 3.28 μg/m3 for the ambient samples (in chronological order, ). Further measurement of ammonium transmission with ammonium sulfate particles showed 1.09 ± 0.2% transmission (consistent with sulfate at 0.8 ± 0.1%), equivalent to a minimum ambient level of 2.75 μg/m3, to which our measured ambient concentrations are quite close. Additionally, HTDMA volatile loss can be even higher in common ambient species such as ammonium nitrate. This highlights the limitations to ambient application, especially where ammonium is an important constituent. While it is common to estimate ammonium concentrations to be the amount needed to neutralize sulfate and nitrate, this approach disregards the possibility of both non-neutralized and amine-containing particles, both of which could contribute to the hygroscopicity of the particle (Duplissy et al. Citation2011).
Figure 6. (left panel) Number size distributions (dashed line, left axis) and mass concentration of total organics, sulfate, nitrate, and ammonium (solid colored lines, right axis) vs. hygroscopic growth factor for ambient particles. Colored dashed lines show the detection limit for the HR-ToF-AMS (Decarlo et al. Citation2006). There are 30 bins per scan for the 30 September sample and 40 bins for the 14 October samples. (right panel) Mass spectra for the organic mode bin of each sample; legends show total nitrate-equivalent mass contributed by fragments of the indicated species.
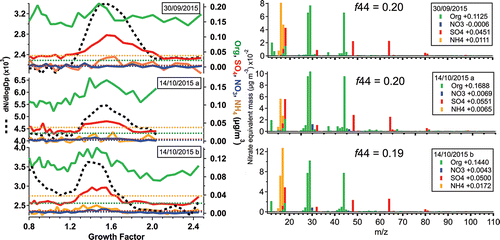
For the 30 September sample, both the non-lognormal shape of the growth factor distribution (mode GF = 1.57) and the x-dimension offset between the organic and sulfate modes suggest that an externally mixed particle population may be at play, highlighting the usefulness of this in-series measurement approach. The organic mode falls at GF = 1.48, much like the other two samples, while the sulfate mode falls at GF = 1.60. For all samples, the organic mode mass spectra are remarkably similar, with predominant organic mass at m/z 44 and f44 ≈ 0.20 indicating high levels of oxidation.
While this technique has been shown to directly and robustly relate hygroscopicity and composition in high-concentration laboratory-generated particles, the severe mass loss observed in the HTDMA limits its practicality for ambient applications. This mass attenuation is native to the HTDMA technique as constraint of the wetted size requires both initial size selection and subsequent scanning size selection, each of which reduces mass transmission. This loss measures ∼73% of mode particle number between DMA1 and DMA2, in which a lognormal distribution is selected, via DMA2, from the lognormal distribution produced by DMA1 (Lopez-Yglesias et al. Citation2014). Given that the ambient size distribution is usually broader and non-lognormal, we could assume that mode count attenuation in DMA1 is ≥73%, giving a maximum estimated transmission of ∼7.3% by count, not accounting for loss during plumbing to the AMS. In the experiments presented herein, great attention was paid to minimizing line losses before the HTDMA and between the HTDMA and AMS; it is therefore unlikely that mass transmission can be increased without a more linear redesign of the HTDMA (which still does not ameliorate loss due to the double size selection). Thus, ambient application of this technique appears to be limited to ambient conditions with >∼20 μg/m3 total PM2.5 (as measured by the AMS), and preferably higher. For instance, with the empirically determined average mass transmission for our HTDMA-AMS system, ∼2.75 μg/m3 of ammonium would be needed in the ambient aerosol for the ammonium detection limit to be reached; with ammonium typically averaging between 6.7% and 19% of ambient particulate mass (Zhang et al. Citation2007), ∼41 and 14 μg/m3 total PM2.5 would be required, respectively, and at minimum, for characterization of the basic particulate composition, charge balance, etc., in the mode bin. Sufficient concentrations are often reported in cities in China, which might be an ideal deployment location for this technique (see Figure 2 in Li et al. Citation2015).
Conclusions
In response to the need for real-time, direct relationship of hygroscopic growth factor and particle composition for a broader range of ambient particles, a hybrid tandem differential mobility analyzer (HTDMA) was placed in series before an aerodyne high-resolution aerosol mass spectrometer (AMS). In short, particles of an initial size D0 are selected in a first DMA, humidified, and then sampled through a second, scanning humidified DMA such that the hygroscopic growth factor (GF = Dhumid/D0) is determined for each scanned bin; the sample stream from the second DMA is routed to a condensation particle counter (giving the growth factor measurements) and the AMS (giving quantitative composition measurements). This double size selection leads to significant mass loss, with an average of 1.8% of ambient particulate mass transmitted through the system. To increase mass transmission through the HTDMA, the DMA1 sample:sheath flow ratio was tuned to increase the amplitude and width of the selected particle size distribution, with the necessary caveat of slightly degraded size selection precision. Method evaluation began with external mixtures of polystyrene latex spheres (PSLs) and ammonium sulfate, both of which are nominally non-volatile, humidified to 90% RH. Non-hygroscopic PSLs should and did show a growth factor near 1, while hygroscopic ammonium sulfate grew to GF = 1.72 ± 0.02, consistent with the literature, producing a bimodal growth factor curve accompanied by the expected changes in composition. Another experiment involving ammonium sulfate-humic acid particles externally mixed with ammonium nitrate particles showed this technique capable of distinguishing between particle populations with similar growth factors but different compositions, even when one of the particle types is in relatively small ratio. Next, fresh mosquito-coil smoke, which has clear BBOA mass spectral markers and a GF near 1, was mixed externally with AN-AS-humic acid or AN-glyoxylic acid particles to simulate the mixing of ‘‘fresh” emissions with the typically secondary, oxidized particles found in urban outflows. Here, too, the system performed well, separating the two hygroscopic modes and producing composition measurements (mass spectra) like those of the unmixed particle type. Finally, ambient particles were analyzed. Composition and corresponding growth factors were broadly consistent with the literature, but due to the high mass loss in the system, ammonium was not detected. We approximate that, in order to characterize the basic particulate composition (organics, NH4, NO3, and SO4), charge balance, etc., in the mode bin, ambient PM2.5 concentrations would need to be at least ∼14 μg/m3 (for particles 19% NH4 by mass) to 41 μg/m3 (for particles 6.7% NH4 by mass). In summary, this novel, online technique is capable of directly measuring particle hygroscopicity and non-refractory composition and may be readily applied in the laboratory, but is limited in ambient application to fairly high-concentration particle episodes.
UAST_1309350_Supplemental_File.zip
Download Zip (264.3 KB)Funding
This work was funded by the University Grants Committee (General Research Fund 600413) and the Environment and Conservation Fund (ECWW09EG04) of Hong Kong.
References
- Aklilu, Y., Mozurkewich, M., Prenni, A. J., Kreidenweis, S. M., Alfarra, M. R., Allan, J. D., Anlauf, K., Brook, J., Leaitch, W. R., Sharma, S., Boudries, H., and Worsnop, D. R. (2006). Hygroscopicity of Particles at Two Rural, Urban Influenced Sites During Pacific 2001: Comparison with Estimates of Water Uptake from Particle Composition. Atmos. Environ., 40(15):2650–2661, doi:10.1016/j.atmosenv.2005.11.063.
- Benedict, K. B., Carrico, C. M., Kreidenweis, S. M., Schichtel, B., Malm, W. C., and Collett, J. L. (2013). A Seasonal Nitrogen Deposition Budget for Rocky Mountain National Park. Ecol. Appl., 23(5):1156–1169, doi:10.1890/12-1624.1.
- Boreddy, S. K. R., Kawamura, K., Mkoma, S., and Fu, P. (2014). Hygroscopic Behavior of Water-Soluble Matter Extracted from Biomass Burning Aerosols Collected at a Rural Site in Tanzania, East Africa. J. Geophys. Res. Atmos., 119:12,233–12,245, doi:10.1002/2014JD021546.
- Carrico, C. M., Kreidenweis, S. M., Malm, W. C., Day, D. E., Lee, T., Carrillo, J., McMeeking, G. R., and Collett, J. L. (2005). Hygroscopic Growth Behavior of a Carbon-Dominated Aerosol in Yosemite National Park. Atmos. Environ., 39(8):1393–1404, doi:10.1016/j.atmosenv.2004.11.029.
- Chan, M. N., and Chan, C. K. (2003). Hygroscopic Properties of Two Model Humic-Like Substances and Their Mixtures with Inorganics of Atmospheric Importance. Environ. Sci. Technol., 37:5109–5115.
- Chan, M. N., and Chan, C. K. (2005). Mass Transfer Effects in Hygroscopic Measurements of Aerosol Particles. Atmos. Chem. Phys., 5(10):2703–2712, doi:10.5194/acp-5-2703-2005.
- Decarlo, P. F., Kimmel, J. R., Trimborn, A., Northway, M. J., Jayne, J. T., Aiken, A. C., Gonin, M., Fuhrer, K., Horvath, T., Docherty, K. S., Worsnop, D. R., and Jimenez, J. L. (2006). Field-Deployable, High-Resolution, Time-of-Flight Aerosol Mass Spectrometer. Anal. Chem., 78(24):8281–8289, doi:10.1021/ac061249n.
- Duplissy, J., DeCarlo, P. F., Dommen, J., Alfarra, M. R., Metzger, A., Barmpadimos, I., Prevot, A. S. H., Weingartner, E., Tritscher, T., Gysel, M., Aiken, A. C., Jimenez, J. L., Canagaratna, M. R., Worsnop, D. R., Collins, D. R., Tomlinson, J., and Baltensperger, U. (2011). Relating Hygroscopicity and Composition of Organic Aerosol Particulate Matter. Atmos. Chem. Phys., 11(3):1155–1165, doi:10.5194/acp-11-1155-2011.
- Gysel, M., Crosier, J., Topping, D. O., Whitehead, J. D., Bower, K. N., Cubison, M. J., Williams, P. I., Flynn, M. J., McFiggans, G. B., and Coe, H. (2007). Closure Study Between Chemical Composition and Hygroscopic Growth of Aerosol Particles During TORCH2. Atmos. Chem. Phys., 7(24):6131–6144, doi:10.5194/acp-7-6131-2007.
- Hatch, L. E., Creamean, J. M., Ault, A. P., Surratt, J. D., Chan, M. N., Seinfeld, J. H., Edgerton, E. S., Su, Y., and Prather, K. A. (2011). Measurements of Isoprene-Derived Organosulfates in Ambient Aerosols by Aerosol Time-of-Flight Mass Spectrometry—Part 2: Temporal Variability and Formation Mechanisms. Environ. Sci. Technol., 45(20):8648–8655, doi:10.1021/es2011836.
- Herich, H., Kammermann, L., Gysel, M., Weingartner, E., Baltensperger, U., Lohmann, U., and Cziczo, D. J. (2008). In Situ Determination of Atmospheric Aerosol Composition as a Function of Hygroscopic Growth, J. Geophys. Res., 113, D16213, doi:10.1029/2008JD009954.
- Hu, D., Chen, J., Ye, X., Li, L., and Yang, X. (2011). Hygroscopicity and Evaporation of Ammonium Chloride and Ammonium Nitrate: Relative Humidity and Size Effects on the Growth Factor. Atmos. Environ., 45(14):2349–2355, doi:10.1016/j.atmosenv.2011.02.024.
- IPCC. (2014). IPCC, 2014: Climate Change 2014: Synthesis Report, in Contribution of Working Groups I, II and III to the Fifth Assessment Report of the Intergovernmental Panel on Climate Change, R. K. Pachauri, and L. A. Meyer, eds., IPCC, Geneva, Switzerland, 151 pp.
- Kanakidou, M., Seinfeld, J. H., Pandis, S. N., Barnes, I., Dentener, F. J., Facchini, M. C., Van Dingenen, R., Ervens, B., Nenes, A., Nielsen, C. J., Swietlicki, E., Putaud, J. P., Balkanski, Y., Fuzzi, S., Horth, J., Moortgat, G. K., Winterhalter, R., Myhre, C. E. L., Tsigaridis, K., Vignati, E., Stephanou, E. G., and Wilson, J. (2005). Organic Aerosol and Global Climate Modelling: A Review. Atmos. Chem. Phys., 5(4):1053–1123, doi:10.5194/acp-5-1053-2005.
- Lanz, V. A., Alfarra, M. R., Baltensperger, U., Buchmann, B., Hueglin, C., and Pr, A. S. H. (2007). Source Apportionment of Submicron Organic Aerosols at an Urban Site by Factor Analytical Modelling of Aerosol Mass Spectra. Atmos. Chem. Phys., 2004:1503–1522.
- Levin, E. J. T., Kreidenweis, S. M., McMeeking, G. R., Carrico, C. M., Collett Jr., J. L., and Malm, W. C. (2009). Aerosol Physical, Chemical and Optical Properties During the Rocky Mountain Airborne Nitrogen and Sulfur study. Atmos. Environ., 43(11):1932–1939, doi:10.1016/j.atmosenv.2008.12.042.
- Li, Y. J., Lee, B. P., Su, L., Fung, J. C. H., and Chan, C. K. (2015). Seasonal Characteristics of Fine Particulate Matter (PM) Based on High-Resolution Time-of-Flight Aerosol Mass Spectrometric (HR-ToF-AMS) Measurements at the HKUST Supersite in Hong Kong. Atmos. Chem. Phys., 15(1):37–53, doi:10.5194/acp-15-37-2015.
- Li, Y. J., Yeung, J. W. T., Leung, T. P. I., Lau, A. P. S., and Chan, C. K. (2012). Characterization of Organic Particles from Incense Burning Using an Aerodyne High-Resolution Time-of-Flight Aerosol Mass Spectrometer. Aerosol Sci. Technol., 46:654–665.
- Lim, Y. B., Tan, Y., Perri, M. J., Seitzinger, S. P., and Turpin, B. J. (2010). Aqueous Chemistry and Its Role in Secondary Organic Aerosol (SOA) Formation. Atmos. Chem. Phys., 10(21):10521–10539, doi:10.5194/acp-10-10521-2010.
- Lopez-Yglesias, X. F., Yeung, M. C., Dey, S. E., Brechtel, F. J., and Chan, C. K. (2014). Performance Evaluation of the Brechtel Mfg. Humidified Tandem Differential Mobility Analyzer (BMI HTDMA) for Studying Hygroscopic Properties of Aerosol Particles. Aerosol Sci. Technol., 48(9):969–980.
- Malm, W. C., Day, D. E., Kreidenweis, S. M., Collett, J. L., Carrico, C. M., McMeeking, G. R., and Lee, T. (2005). Hygroscopic Properties of an Organic-Laden Aerosol. Atmos. Environ., 39(27):4969–4982.
- McMeeking, G. R., Good, N., Petters, M. D., McFiggans, G., and Coe, H. (2011). Influences on the Fraction of Hydrophobic and Hydrophilic Black Carbon in the Atmosphere, Atmos. Chem. Phys., 11(10):5099–5112, doi:10.5194/acp-11-5099-2011.
- McMurry, P. H., Litchy, M., Huang, P.-F., Cai, X., Turpin, B. J., Dick, W. D., and Hanson, A. (1996). Elemental Composition and Morphology of Individual Particles Separated by Size and Hygroscopicity with the TDMA. Atmos. Environ., 30(1):101–108, doi:10.1016/1352-2310(95)00235-Q.
- Mikhailov, E., Vlasenko, S., Niessner, R., and Pöschl, U. (2004). Interaction of Aerosol Particles Composed of Protein and Salts with Water Vapor: Hygroscopic Growth and Microstructural Rearrangement. Atmos. Chem. Phys., 4(2):323–350, doi:10.5194/acp-4-323-2004.
- Ng, N. L., Canagaratna, M. R., Jimenez, J. L., Zhang, Q., Ulbrich, I. M., and Worsnop, D. R. (2011). Real-Time Methods for Estimating Organic Component Mass Concentrations from Aerosol Mass Spectrometer Data. Environ. Sci. Technol., 45(3):910–916, doi:10.1021/es102951k.
- Peng, C., Chan, M. N., and Chan, C. K. (2001). The Hygroscopic Properties of Dicarboxylic and Multifunctional Acids: Measurements and UNIFAC Predictions. Environ. Sci. Technol., 35(22):4495–4501.
- Pitchford, M. L., and McMurry, P. H. (1994). Relationship Between Measured Water Vapor Growth and Chemistry of Atmospheric Aerosol for Grand Canyon, Arizona, in Winter 1990. Atmos. Environ., 28(5):827–839, doi:10.1016/1352-2310(94)90242-9.
- Stokes, R. H., and Robinson, R. A. (1966). Interactions in Aqueous Nonelectrolyte Solutions. I: Solute-Solvent Equilibria. J. Phys. Chem.-US., 70:2126–2130.
- Swietlicki, E., Hansson, H.-C., Hameri, K., Svenningsson, B., Massling, A., McFiggans, G., McMurry, P. H., Petaja, T., Tunved, P., Gysel, M., Topping, D., Weingartner, E., Baltensperger, U., Rissler, J., Wiedensohler, A., and Kulmala, M. (2008). Hygroscopic Properties of Submicrometer Atmospheric Aerosol Particles Measured with H-TDMA Instruments in Various Environments—A Review. Tellus B, 60B:432–469.
- Ulbrich, I. M., Canagaratna, M. R., Zhang, Q., Worsnop, D. R., and Jimenez, J. L. (2009). Interpretation of Organic Components from Positive Matrix Factorization of Aerosol Mass Spectrometric Data. Atmos. Chem. Phys., 9(9):2891–2918, doi:10.5194/acp-9-2891-2009.
- Wang, X., Ye, X., Chen, H., Chen, J., Yang, X., and Gross, D. S. (2014). Online Hygroscopicity and Chemical Measurement of Urban Aerosol in Shanghai, China, Atmos. Environ., 95: 318–326, doi:10.1016/j.atmosenv.2014.06.051.
- Wise, M. E., Surratt, J. D., Curtis, D. B., Shilling, J. E., and Tolbert, M. A. (2003). Hygroscopic Growth of Ammonium Sulfate/Dicarboxylic Acids. J. Geophys. Res., 108(D20):4638, doi:10.1029/2003JD003775.
- Yeung, M. C., Lee, B. P., Li, Y. J., and Chan, C. K. (2014). Simultaneous HTDMA and HR-ToF-AMS measurements at the HKUST Supersite in Hong Kong in 2011. J. Geophys. Res. Atmos., 119(16):9864–9883, doi:10.1002/2013JD021146.
- Zamora, I. R., Tabazadeh, A., Golden, D. M., and Jacobson, M. Z. (2011). Hygroscopic Growth of Common Organic Aerosol Solutes, Including Humic Substances, as Derived from Water Activity Measurements. J. Geophys. Res. Atmos., 116(D23):n/a–n/a, doi:10.1029/2011JD016067.
- Zdanovskii, A. (1948). New Methods for Calculating Solubilities of Electrolytes in Multicomponent Systems. Zhur. Fiz. Khim., 22:1475–1485.
- Zelenyuk, A., Imre, D., Han, J.-H., and Oatis, S. (2008). Simultaneous Measurements of Individual Ambient Particle Size, Composition, Effective Density, and Hygroscopicity., Anal. Chem., 80(5):1401–7, doi:10.1021/ac701723v.
- Zhang, Q., Jimenez, J. L., Canagaratna, M. R., Allan, J. D., Coe, H., Ulbrich, I. M., Alfarra, M. R., Takami, A., Middlebrook, A. M., Sun, Y. L., Dzepina, K., Dunlea, E., Docherty, K., Decarlo, P. F., Salcedo, D., Onasch, T., Jayne, J. T., Miyoshi, T., Shimono, A., Hatakeyama, S., Takegawa, N., Kondo, Y., Schneider, J., Drewnick, F., Borrmann, S., Weimer, S., Demerjian, K., Williams, P., Bower, K., Bahreini, R., Cottrell, L., Griffin, R. J., Rautiainen, J., Sun, J. Y., Zhang, Y. M., and Worsnop, D. R. (2007). Ubiquity and Dominance of Oxygenated Species in Organic Aerosols in Anthropogenically-Influenced Northern Hemisphere Midlatitudes. Geophys. Res. Lett., 34(13):1–6, doi:10.1029/2007GL029979.