ABSTRACT
Here, we present a concept of a personal electrostatic bioaerosol sampler (PEBS), which is an open channel collector consisting of a novel wire-to-wire particle charger and a collection section housing a double-sided and removable metal collection plate and two quarter-cylinder ground electrodes. The charger consists of a tungsten wire (25.4 mm long and 0.076 mm in diameter) connected to high voltage and positioned in the center of the charging section (a cylinder 50.8 mm long and 25.4 mm in diameter); a ring of stainless steel wire 0.381 mm in diameter surrounds the hot electrode at its midpoint and is grounded. The newly designed wire-to-wire charger produces lower ozone concentrations compared to traditional wire-to-plate or wire-to-cylinder charger designs. The particles captured on the collection plate are easily eluted using water or other fluids. The sampler was iteratively optimized for optimum charging and collection voltages, and collection electrode geometry. When tested with polystyrene latex particles ranging from 0.026 µm to 3.1 µm in diameter and 10 L/min collection flow rate, the sampler's collection efficiency was approximately 70%–80% at charging and collection voltages of +5.5 kV and −7 kV, respectively. The PEBS showed this collection efficiency at sampling times ranging from 10 min to 4 h. Preliminary tests with Bacillus atrophaeus bacterial cells and fungal spores of Penicillium chrysogenum showed similar collection efficiency. The use of a unique wire-to-wire charger resulted in ozone production below 10 ppb. Due to low ozone emissions, this sampler will allow maintaining desirable physiological characteristics of the collected bioaerosols, leading to a more accurate sample analysis.
© 2017 American Association for Aerosol Research
EDITOR:
Introduction
Over the last few decades, a substantial number of studies investigating exposures to bioaerosols (e.g., bacteria, fungi, viruses, allergens, endotoxins, and mycotoxins) and health effects (e.g., infectious diseases, acute toxic effects, and allergies) due to such exposures have been published (Schachter et al. Citation1984; Kenny et al. Citation1999; Douwes et al. Citation2003; Fung and Hughson Citation2003; Asefa et al. Citation2009; Persoons et al. Citation2010; Duquenne et al. Citation2013; Eduard et al. Citation2012; Madsen et al. Citation2012b; Napoli et al. Citation2012). Exposures to bioaerosols occur mainly via inhalation (Pearson et al. Citation2015; Nazaroff Citation2016), and the potential health effects depend on intensity, frequency, and duration of exposure (Cookingham and Solomon Citation1995; Dosemeci et al. Citation2002; Preller et al. Citation2004). Thus, to accurately assess exposures to bioaerosols, efficient and reliable air sampling tools capable of obtaining representative bioaerosol samples are needed (Madsen et al. Citation2006, Citation2012a).
Many stationary and portable bioaerosol samplers have been developed and used to assess exposures to bioaerosols (Mandal and Brandl Citation2011). Among those, inertia-based particle collection devices, e.g., impingers and impactors, remain the most popular bioaerosol sampling tools (Macher Citation1997; Kesavan and Sagripanti Citation2015). Over the past years, several existing personal samplers for particulate matter were adapted and used for personal and area sampling of bioaerosols, including application of Button Aerosol Sampler and IOM cassette with regular or gelatin filters (Aizenberg et al. Citation2000; Yao and Mainelis Citation2007; Wu et al. Citation2010; Chang and Hung Citation2012). Several new personal bioaerosol sampler concepts, such as rotating cup (Gorner et al. Citation2006) and microcentrifuge tube (Lindsley et al. Citation2006; Macher et al. Citation2008; Su et al. Citation2012) have been proposed. In addition to samplers based on inertia and filtration, several electrostatics-based bioaerosol samplers, including those designed for area and even personal sampling, have been developed and applied (Mainelis et al. Citation1999, Citation2002a, Citation2002b; Yao and Mainelis Citation2006; Han and Mainelis Citation2008; Madsen and Sharma Citation2008; Tan et al. Citation2011; Park et al. Citation2015; Roux et al. Citation2016). Compared to inertial sampling techniques, electrostatic samplers have a number of advantages: lower power requirements, lower pressure drop, and lower impaction stress on the organisms (Mainelis et al. Citation1999; Tan et al. Citation2011). In electrostatic collectors, incoming particles are electrically charged (Hewitt Citation1957; Awad and Castle Citation1975) and then deposited on/into collection substrate by the action of electrostatic forces. The charging of particles is achieved either via diffusion or field-charging mechanism (Liu and Yeh Citation1968). For the latter, wire-plate (Xiangrong et al. Citation2002) or wire-cylinder (Niewulis et al. Citation2014) designs are most commonly used. While the field-charging mechanism is an efficient charging process, a number of issues related to its application for bioaerosols still remain: ozone emission during charging process (Chang et al. Citation1991), charger degradation over time (Koutsoubis and MacGregor Citation2000), and difficulty in charging smaller particles (Tsai et al. Citation2010). Among these concerns, ozone emission is probably the biggest issue not only due to its effect on the collected microorganisms (Kammer Citation2005) but also because ozone is an irritant to the lungs (Francis et al. Citation2017). While the current U.S. National Ambient Air Quality Standard for ozone is 70 ppb (50CFR65292), there are currently limited regulations governing ozone emissions from personal-use devices. For example, the Food and Drug Administration (FDA) standard limits ozone output of indoor medical devices to 50 ppb (21CFR801.415); this level is stricter than 100 ppb standard for 8-h exposures (not emissions) in occupational environments set by Occupational Safety and Health Administration (54CFR23332). Thus, at the very least, a personal sampling device should satisfy FDA requirements and, even better, have much lower ozone emissions. Overall, ozone emissions depend on the sampling flow rate, operational voltage and its polarity, current level, electric field strength, relative humidity, and size and material of a charging electrode (Castle et al. Citation1969; Goheen et al. Citation1984; Boelter and Davidson Citation1997; Kulkarni et al. Citation2002; Plank et al. Citation2014). An earlier study showed that when carbon fibers are used in particle chargers instead of the often-used tungsten wire, ozone production is lower when other operational characteristics are the same (Han et al. Citation2009). However, our own investigation showed that charging efficiency of carbon fibers is not stable over time resulting in widely fluctuating ozone emissions because the carbon fibers become contaminated by dust and particles after use (Han and Mainelis, unpublished data). It is known that residues on ionizer electrodes compromise their performance by reducing ion density and by generating particles (Murray et al. Citation1992).
In this article, we present a concept and performance of a novel personal electrostatic bioaerosol sampler (PEBS) that eliminates or minimizes some of the issues above, including ozone production. The presented sampler has the following features: (Equation1[1] ) a two-stage electrostatic precipitator design featuring open channel geometry, (Equation2
[2] ) a wire-to-wire charger design resulting in low ozone production, (Equation3
[3] ) a relatively high sampling flow rate, (Equation4
[4] ) an easily accessible collection plate, from which the collected particles can be washed off with a nominal amount of liquid, and (5) use of liquid as elution fluid allowing sample analysis by multiple techniques. These sampler's characteristics, especially high sampling flow rate and low amount of elution liquid result in high sample concentration rate (Han and Mainelis Citation2008) that would allow measuring exposures even to low microorganism concentrations—a feature lacking in current personal bioaerosol samplers—thus substantially improving the ability to identify exposure risks and protect affected populations. Because of low power requirements, the presented sampler concept can be developed into a self-contained personal sampler.
Thus, the main goal of this study was to introduce the PEBS concept and to investigate its feasibility for achieving high collection efficiency and high sample concentration rate while maintaining low ozone production. As part of this process, the collection efficiency was examined as a function of operating voltage, sampler's geometric parameters, sampling flow rate, and operating time. The experiments in this stage of the development have been performed with polystyrene latex (PSL) particles ranging from nanosized (26 nm) to super micron (3.1 µm) in size and two microorganism species (i.e., Bacillus atrophaeus bacterial cells and Penicillium chrysogenum fungal spores).
Materials and methods
General design principles
A number of factors affect ESP design and performance, including particle terminal drift velocity, which is determined by the operational voltage(s) and particle electrical mobility, sampler's geometrical parameters, and volumetric air flow rate. The performance of traditional wire-to-plate ESPs could be typically described by Deutsch–Andersen equation (Nóbrega et al. Citation2001) or its modified version (Lin et al. Citation2012). However, in most cases, this equation serves only as guidance because the actual collection efficiency is considerably affected by air-ion mixing, non-ideal collection patterns, and particle re-entrainment (Yang et al. Citation2009).
Since our goal was to achieve good collection efficiency while maintaining low ozone production, we departed from the traditional wire-to-plate design and used the wire-to-wire approach as described below. Because of the new design and our previous experience in ESP design (Han and Mainelis Citation2008; Han et al. Citation2015a), it seemed more prudent to apply general ESP design principles and develop the sampler by iteration: optimize one design parameter to achieve a collection efficiency of 70% or better while others remain fixed. When deciding on these parameters, we were cognizant that our goal is to design a personal sampler, i.e., the sampler had to be compact. Also, for improved user experience, the collection plate had to fit easily into a standard 50-mL disposable and sterile centrifuge tube for a convenient way to remove, handle, and store the collected particles.
Ozone production is an inescapable consideration when designing an ESP, especially one to be used as a personal bioaerosol sampler. To minimize ozone production, we undertook the following steps: the sampler was designed as a two-stage system (separate charging and collecting sections) for better control of charging process, wire-to-wire charger design, use of positive corona discharge to minimize ozone production (Chen and Davidson Citation2003), use of lowest possible corona current, and elimination of any sharp edges within the sampler to minimize strong, local electrical fields. The text below describes these iteration steps in detail.
Design features of the personal electrostatic bioaerosol sampler (PEBS) with a wire-to-wire charger
The PEBS is comprised of a static “air blender,” a wire-to-wire charger, and a collection chamber (). The entire PEBS has a shape of a cylinder of 2.54 cm (1 inch) in diameter, ∼14 cm (5.5 inches) in length, and is made of a static dissipative material (homopolymer acetal, or Delrin; Professional Plastics Inc., Fullerton, NY, USA). The static blender, which is positioned at the sampler's inlet, has been designed to improve mixing of the incoming aerosol particles with the produced ions. The blender has the shape of a disk 2.54 cm (1 inch) in diameter and 0.56 cm (0.22 inches) in height; it features 6 blades in the inner circle (1.45 cm in diameter) and 15 blades in the outer circle and was printed using 3D printing technology.
Figure 1. Schematic diagram of the personal electrostatic bioaerosol sampler (PEBS) with a wire-to-wire charger. The sampler incorporates a novel particle charger with a 25.4 mm (1 inch) long tungsten wire 0.076 mm (0.003 inches) in diameter positioned in the center of the charging chamber (a cylinder 25.4 mm or 1 inch in diameter) and connected to high voltage; a ring of stainless steel wire (0.381 mm [0.015 inches] in diameter) is surrounding the hot electrode at its midpoint and is grounded.
![Figure 1. Schematic diagram of the personal electrostatic bioaerosol sampler (PEBS) with a wire-to-wire charger. The sampler incorporates a novel particle charger with a 25.4 mm (1 inch) long tungsten wire 0.076 mm (0.003 inches) in diameter positioned in the center of the charging chamber (a cylinder 25.4 mm or 1 inch in diameter) and connected to high voltage; a ring of stainless steel wire (0.381 mm [0.015 inches] in diameter) is surrounding the hot electrode at its midpoint and is grounded.](/cms/asset/51c5cb0a-cca5-41f2-8829-d8faa2169a59/uast_a_1329516_f0001_b.gif)
To achieve high collection efficiency with low ozone production, the sampler features a novel wire-to-wire charger, where a tungsten wire 2.54 cm (1 inch) in length and 0.076 mm (0.003 inches) in diameter (W91, Scientific Instrument Inc., Ringoes, NJ, USA) is positioned at a distance of 1.27 cm (0.5 inches) downstream of the inlet and in the center of the charging chamber (i.e., 1-inch diameter cylinder); it is connected to DC high voltage. A ring of stainless steel wire 0.381 mm (0.015 inches) in diameter is installed on the inside of the cylinder at the middle point and at 90° angle to tungsten wire and grounded. The tungsten wire is supported by ceramic mini posts of 1.575 mm (0.062 inches) outer diameter and 0.787 mm (0.031 inches) inner diameter, which provide insulation for a conduit to the wire. Since the tungsten wire is connected to the positive voltage and the stainless steel wire electrode is grounded, this wire-to-wire configuration creates sufficient ions, which charge the incoming particles while producing low ozone emissions during the charging process. Positive charging is the preferred approach for biological particles as the production of ozone can be up to one order of magnitude lower than the production of ozone in the negative corona (Chen and Davidson Citation2003).
Although many studies have examined how the ozone production is affected by various ESP design parameters (e.g., current level, ionizer wire diameter, wire material, and electric field strength (Castle et al. Citation1969; Awad and Castle Citation1975; Nashimoto 1988; Ohkubo et al. Citation1990; Viner et al. Citation1992; Plank et al. Citation2014), we did not see studies on the physical dimensions of the ground electrode and ozone production. In our design, the area of the ground electrode is minimized, and it should lead to a lower deposition of particles and ions on the ground electrode in the charging section. This, in turn, should result in a lower corona current and, since the ozone production is proportional to the current level (Castle et al. Citation1969; Viner et al. Citation1992), lower ozone production.
The collection section consists of two grounded stainless steel plates having the shapes of the quarter cylinder and a stainless steel collection plate, which is connected to collection voltage. The collection plate divides the collection section into two half-cylinder collection chambers. The collection plate automatically connects to the collection voltage once it is slid into the grooves in the inner wall of the chamber with an electric connection. After completing the sampling, the collection plate is removed from the collector for sample elution and analysis. Because the design of the collection section is symmetrical and the particles are collected on both sides of the plate, particles from each side could be eluted separately thus allowing to have two identical samples (). The two samples could be analyzed separately for different purposes or by different techniques (e.g., microscopy, fluorometry, etc.) or they could be combined if needed. The collection plate is 3.81 cm (1.5 inches) long, 2.54 cm (1 inch) wide, and 0.16 cm (1/16 inches) thick. Each quarter-cylinder grounded electrode has a length of 3.81 cm (1.5 inches), the circumference of 1.99 cm (π × 1/4 inches), and thickness of 0.04 cm (1/64 inches); they are inserted into grooves in the middle of the chamber. The outlet of the collector connects to an air mover with adjustable flow rate.
Experimental setup for testing PEBS with PSL particles in laboratory
The test system is shown in , and it consisted of a flow controller, a particle generator, an air-particle mixing element, a flow straightener, a test chamber, and a particle monitor. The system was housed inside a Class II Biosafety cabinet (NUAIRE Inc., Plymouth, MN, USA).
A six-jet Collison nebulizer (Mesa Laboratories Inc., Butler, NJ, USA) with a glass jar was used to aerosolize test particles from a liquid suspension at a flow rate (QA) of 5 L/min (pressure of 12 psi), and the aerosolized particles were combined with a dry air flow, Qd (5 L/min). The dry air and aerosolized particle stream were combined (Qd +QA = 10 L/min) and passed through a 2-mCi Po-210 charge neutralizer (Amstat Industries Inc., Glenview, IL) to reduce aerosolization-imparted particle charges to Boltzmann charge equilibrium. A HEPA-filtered dilution air flow, QD (60 L/min), provided by an in-house compressor was used to dilute the particle stream; it was controlled by a pressure regulator and monitored by a mass flowmeter (TSI Inc., Shoreview, MN, USA). The electrically neutralized particles then passed through two mixing boxes connected by a U-type duct connector to improve the uniformity of particle distribution across the flow cross-section (Han et al. Citation2005). A well-mixed flow stream then entered a raised test duct 15.2 cm (6 inches) in diameter and a 61 cm (24 inches) in length, as shown in . A flow straightener (honeycomb) was placed at the exit of the second elbow to eliminate large-scale turbulence and flow swirl generated by the mixing boxes and the 90° elbows. A raised test duct allowed the PEBS collector to be perpendicularly oriented relative to the air stream. This arrangement simulated a real-world sampling situation, where a person would wear a sampler in a vertical orientation in the upper part of their chest. The sampler was positioned six duct diameters downstream of the exit of the flow straightener in order to provide a uniform cross-sectional profile of test particles.
The PEBS was tested with six different aerodynamic diameters (0.026, 0.1, 0.2, 0.5, 1.0, and 3.1 µm) of green fluorescent polystyrene latex (PSL) particles (Duke Scientific Corp., Palo Alto, CA, USA). The airborne concentration of fluorescent PSL particles was approximately 103–104/L. The coefficient of variation (COV) of 0.5 µm PSL concentration across the test duct was about 2.7% at the measurement location. The COV was measured over five equally distributed sampling points in the cross-sectional area of the duct in triplicate.
In our tests, the PEBS was operated at a sampling flow rate (QS) of 10, 20, and 30 L/min provided by a vacuum pump. The collector was tested at charging voltages ranging from +5 kV to +8 kV, and collection voltages ranging from −3 kV to −7 kV. The sampling time varied from 2 to 240 min. At this stage of the project, the stainless steel collection electrode was not coated with any materials. The ozone concentration was measured using a UV photometric ozone monitor (Model 202, 2B Technologies Inc., Boulder, CO, USA) downstream of the PEBS ().
Determination of the collection efficiency
The collection efficiency of the PEBS was determined by comparing particle number concentration downstream of the collector with its charging/collection voltages ON and OFF either using a Grimm optical particles counter (OPC) (model 1.108, Grimm Technologies Inc., Douglasville, GA, USA) or a P-Trak (UPC 8525, TSI Inc.) connected to an isokinetic probe (Apex Instruments Inc., Fuquay-Varina, NC, USA). The Grimm was used for larger particles (0.5, 1, and 3.1 µm PSL), while P-Trak was used for smaller particles (0.026, 0.1, and 0.2 µm PSL). The use of direct reading instruments allowed performing a high number of experiments, which was important when the sampler underwent multiple adjustments in the initial phase of its development. When determining the collection efficiency by comparing the particle concentration downstream of the sampler with its voltage ON and OFF, one has to keep in mind that this efficiency ηEFF, is a sum of the collection efficiency of the charging section (ηEFF, CHARGER, i.e., losses in the charging section) and the subsequent collection of particles in the collection section (ηEFF, COLLECTOR).
The collection efficiency of the charging section, i.e., losses:[1]
and the collection efficiency of the collection section:[2] where CCHARGER-ON is particle number concentration with charger voltage ON and collector voltage OFF; CCHARGER&COLLECTOR-ON is particle number concentration when both charger and collector voltages are ON; COFF is particle number concentration with both charger and collector voltages OFF.
This metric does not take into account particle losses inside the PEBS. However, our separate investigation of particles deposited inside the sampler on other sampler components showed that those losses were <1% for 1 µm PSL particles when the sampler operated at 10 L/min. The losses inside the PEBS due to its other components (e.g., static blender or walls) were minimal: e.g., transmission efficiencies of approximately 93% through the static blender were observed when testing with 1 μm PSL particles at the highest investigated flow rate of 30 L/min. Thus, to simplify the measurement procedures, the COFF was used as a reference value for calculating the sampler's performances in the development stage.
While the collection estimation method described above allowed for the quick development of the sampler, it does not represent the actual collection efficiency, only its surrogate. To determine the actual collection efficiency, one has to compare the concentration of airborne test particles determined by the sampler, which is based on particles deposited on the collection plate and the sampling flow rate, and particle concentration upstream of the sampler determined by the reference method. Thus, the actual collection efficiency, ηACTUAL, COLLECTOR was determined by comparing the mass concentration of particles deposited on the PEBS collection electrode and removed by 5 mL of ethyl acetate with the mass of PSL particles isokinetically sampled onto a reference filter (25 mm cellulose membrane, Pall Inc., East Hills, NY, USA) positioned upstream of the sampler and operated at flow rate 2.2 L/min. PSL particle concentration in each sample was determined by measuring its fluorescence intensity using a digital filter fluorometer (Turner Quantech model FM109515, Barnstead/Thermolyne Corp., Dubuque, IA, USA) as described previously (Han and Mainelis Citation2008; Han et al. Citation2015a). The actual collection efficiency, ηACTUAL, COLLECTOR, was determined as follows:[3] where Ccollector and Creference filter are the concentrations of airborne PSL determined based on the amount of PSL captured by the collection plate and the reference filter, respectively. The calculations of these concentrations take into account the sampling flow rates, sample elution volumes, and volumes of sample aliquots used to measure fluorescence intensities (Han and Mainelis Citation2008). Because sample analysis by fluorescence is more time-consuming than the use of direct reading instruments described above, it was used only in the final stages of the sampler development. One of the figures presented below compared the efficiencies determined by the two methods.
In addition to collection efficiency, the sampler's concentration rate, RC (min−1), was calculated using the sampler's operational parameters as follows (Han and Mainelis Citation2008; Han et al. Citation2010, Citation2015b):[4] where Qs (L/min) is the sampling flow rate, VW (L) is the volume of the sample elution liquid, and ηEFF, COLLECTOR is the collection efficiency based on Equation (Equation2
[2] ).
Pilot tests with biological particles
The PEBS was pilot tested with two microorganisms, gram-positive Bacillus atrophaeus bacterial cells (ATCC 49337, American Type Culture Collection, MD, USA) and fungal spores Penicillium chrysogenum (ATCC 10135), at sampling flow rates QS = 10 L/min. Both microorganism species have been widely used in bioaerosol studies (Johnson et al. Citation1994; Hill et al. Citation1999; Nadkarni et al. Citation2002). The bacteria were cultured in nutrient broth (Becton, Dickinson and Company, Sparks, MD, USA) for 18 h at 30°C as per our established protocol (Han et al. Citation2015b). The cells were harvested by centrifugation at 7000 rpm (6140 g) for 5 min at 4°C (BR4, Jouan Inc., Winchester, VA, USA) and then washed four times with sterile DI water (Millipore Corp., Billerica, MA, USA). The final liquid suspension was diluted with sterile DI water to obtain a target airborne cell concentration of ∼104 cells/L as determined by the Grimm OPC. P. chrysogenum was plated onto Sabouraud dextrose agar (Becton Dickinson Microbiology Systems, Cockeysville, MD, USA) and incubated at room temperature (approximately 26°C) for 7 days following an established protocol (Han et al. Citation2015b). After incubation, about 3 mL of sterile deionized water was added to each plate of P. chrysogenum, and the spores were gently harvested from mycelium using a cell spreader. The volume of the resulting spore suspension was then increased to 50 mL. Fresh liquid suspension of 10 mL of each species was prepared for each test, and it was aerosolized using a three-jet Collison nebulizer with a polycarbonate jar, operated at a flow rate of 5 L/min (pressure of 12 psi). Polycarbonate jar was used to minimize damage to the microorganisms (Zhen et al. Citation2013, Citation2014). The collection efficiency was determined by measuring the bioaerosol number concentrations upstream and downstream of PEBS by a Grimm OPC and by using Equations (Equation1[1] ) and (Equation2
[2] ).
Results and discussion
shows the collection efficiencies of the charger (i.e., losses) and the collector of the PEBS as a function of charging voltage when sampling 1 µm PSL particles at different flow rates (10, 20, and 30 L/min) at a fixed collection voltage of −7 kV. The charging voltage was varied from +5 to +6 kV at 10 L/min sampling flow rate, from +6 to +7 kV at 20 L/min, and from +7 to +8 kV at 30 L/min sampling flow rate. As the charging voltage increased at each sampling flow rate, the collection efficiencies of the charger and collector increased to 5.5%–25.2% and 25.1%–72.6% at 10 L/min, respectively; 5.3%–14.6% and 46.2%–60.9% at 20 L/min; 10.4%–17.2% and 33.3%–43.8% at 30 L/min. Overall, as could be expected, when the sampling flow rate increased from 10 to 30 L/min (), the average collection efficiency in the charging and collection sections decreased at all settings of the charging voltage. The decrease was observed because with increasing sampling flow rates particles spent less time in the collection chamber and had a lower chance of being collected. also shows ozone concentrations emitted during PEBS operation. In the data presented here, the background concentration was subtracted. During each test, the temperature in the test chamber stayed in the range of 21–25°C and the relative humidity ranged from 26% to 41%. Ozone emission concentration increased with increasing charging voltage (i.e., field strength): from 2.7 to 17.7 ppb when voltage was increased from +5 to +6 kV at 10 L/min, from 7.4 to 18.8 ppb when voltage was increased from +6 to +7 kV at 20 L/min, and from 9.0 to 17.7 ppb when voltage was increased from +7 to +8 kV at 30 L/min. As could be expected, the ozone concentration decreased with increasing flow rate because the same amount of ozone was diluted in a larger air volume: 17.7 ± 1.4 ppb (10 L/min) vs.7.4 ± 0.1 ppb (20 L/min) at +6 kV charging voltage and 18.8 ± 1.2 ppb (20 L/min) versus 9.0 ± 0.4 ppb (30 L/min) at +7 kV charging voltage. The ozone concentration is not inversely proportional to the flow rate due to its production affected by atmospheric conditions (e.g., temperature, relative humidity) (Chen and Davidson Citation2002).
Figure 3. Collection efficiencies of the charging section (i.e., losses) and the collection section of the PEBS as a function of charging voltage when sampling 1 µm PSL particles at different flow rates (10, 20, and 30 L/min) at a fixed collection voltage of −7 kV. The charging voltage was varied from +5 to +6 kV at 10 L/min sampling flow rate, from +6 to +7 kV at 20 L/min, and from +7 to +8 kV at 30 L/min sampling flow rate. The second y-axis shows ozone emission concentrations by the PEBS with ozone background concentrations removed. Each data point is an average of least three repeats, and the error bars represent standard deviation. In these experiments, a tungsten wire 25.4 mm (1 inch) long and 0.076 mm (0.003 inches) in diameter was used in the charger. The tungsten wire at its midpoint was surrounded by a grounded ring of stainless steel wire 0.381 mm (0.015 inches) in diameter. In the collection section, the collection electrode was a dual-sided stainless steel plate 3.81 × 2.54 × 0.16 cm (1.5 × 1.0 × 1/16 inches). The plate was positioned in the middle of the collection chamber. The ground electrodes were two conductive half-cylinder with dimensions 3.81 × 1.99 × 0.04 cm (1.5 × 0.78 × 1/64 inches).
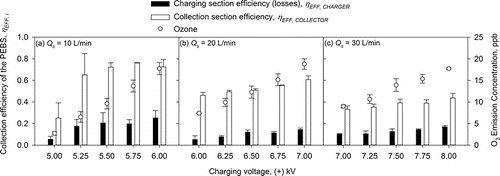
Based on the one-way ANOVA, for 10 L/min sampling flow rate, the collector's efficiency at charging voltage of +5 kV was significantly lower than that at other voltages (p < 0.05); at 20 L/min collection flow rate, the collector's efficiency was not significantly different (p > 0.05) within +6–6.5 kV range of the charging voltage; at 30 L/min sampling flow rate, the collection efficiency was not significantly different (p > 0.05) within +7.25–8 kV range; except for charging voltage of +7 kV. As a result, we selected the +5.5 kV charging voltage at 10 L/min sampling flow rate for further experiments because it yielded a relatively good collection efficiency (∼72%) and a relatively low ozone concentration (<10 ppb). Thus, remaining experiments at 10 L/min sampling flow rate were carried out using this charging voltage of +5.5 kV. For sampling at 20 and 30 L/min flow rates, we selected +6.5 kV and +7.5 kV charging voltages, respectively. These values were used in experiments presented later (for ).
Once the ionizer's charging voltage of +5.5 kV was selected, we determined the optimal collection voltage by testing the collection section efficiency, ηEFF, COLLECTOR, when the collection voltage was varied from −3 to −7 kV at 10 L/min sampling flow rate (). The collection section efficiency was not significantly different for voltages from −4 to −7 kV (p > 0.05); all collection efficiencies at these voltages were higher than the collection efficiency at −3 kV collection voltage. For the collection voltages of −4 kV and higher, the average efficiency in the collection section was 79.6 ± 3.7%, and the average ozone emission concentration was 6.3 ± 0.7 ppb. As shown here, the ozone emissions were independent of the collection voltage. However, additional ozone emissions in the collection section could still occur due to stray discharges (i.e., strong electrostatic fields due to sharp edges of the collection plate or ground plate). This was prevented by smoothing and rounding edges of electrodes in the collection section. Since the collection voltage of −7 kV resulted in the lowest coefficient of variation (COV = 0.009) of the collection efficiency and similar ozone production compared to other voltages above −4 kV, it was selected for further experiments.
Figure 4. The collection efficiency of the PEBS collection section as a function of collection voltage (varied from −3 to −7 kV) when collecting 1 µm PSL particles at 10 L/min flow rate and the fixed charging voltage of +5.5 kV. The second y-axis shows ozone emission concentrations by the PEBS with ozone background concentrations removed. Each data point is an average of least three repeats, and the error bars represent standard deviations. In these experiments, a tungsten wire 25.4 mm (1 inch) long and 0.076 mm (0.003 inches) in diameter was used in the charger. The tungsten wire at its midpoint was surrounded by a grounded ring of stainless steel wire 0.381 mm (0.015 inches) in diameter. In the collection section, the collection electrode was a dual-sided stainless steel plate 3.81 × 2.54 × 0.16 cm (1.5 × 1.0 × 1/16 inches). The plate was positioned in the middle of the collection chamber. The ground electrodes were two conductive half-cylinder with dimensions 3.81 × 1.99 × 0.04 cm (1.5 × 0.78 × 1/64 inches).
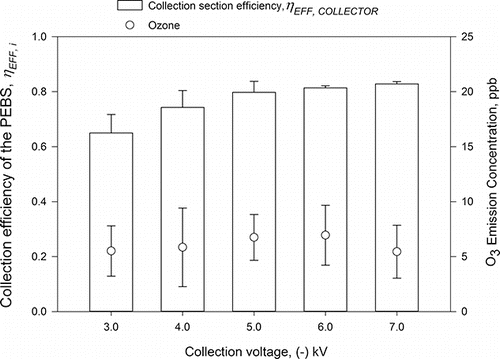
In the next step, the wire-to-wire charger performance was optimized by varying and selecting the wire diameter (i.e., 0.076, 0.203, 0.381, and 0.813 mm) of the ground electrode. The results are presented in . Since the wire diameter of the ground affects the strength of the electrostatic field, it affects ion emission and, in turn, the collection efficiency of both the charging and collection sections as well ozone emission. When +5.5/−7.0 kV for charging/collection was used and the wire diameter was increased from 0.076 mm (0.003 inches) to 0.813 mm (0.032 inches), the collection efficiency on the charging and collection sections increased from 8.7 ± 2.6% to 16.0 ± 3.1% and from 4.4 ± 3.5% to 76.5 ± 0.7%, respectively. At the same time, with increasing wire diameter, ozone emissions increased as well even though the value of the charging voltage remained fixed. As mentioned in our general design principles, it could be attributed to increasing ion current level in the charging section (Castle et al. Citation1969; Viner et al. Citation1992). For the investigated wire diameters, the collection efficiency of the collection section was not significantly different (p > 0.05), except for dw = 0.076 mm (0.003 inches) where the efficiency was ∼5%. Thus, for further experiments, we chose wire of 0.381 mm (0.015 inches) in diameter because it resulted in a lower ozone concentration than 0.813 mm (0.032 inches) wire and a lower COV than 0.381 mm (0.015 inches) wire.
Figure 5. The collection efficiency of the PEBS as a function of the wire diameter of the ground electrode in the charger. The experiments were performed with 1 µm PSL particles at a 10 L/min sampling flow rate and +5.5 kV/−7 kV charging/collection voltage. The efficiency was determined by measuring particle concentration downstream of PEBS with its voltage ON and OFF. The second y-axis shows ozone emission concentrations by the PEBS with ozone background concentrations removed. Each data point is an average of least three repeats, and the error bars represent standard deviations.
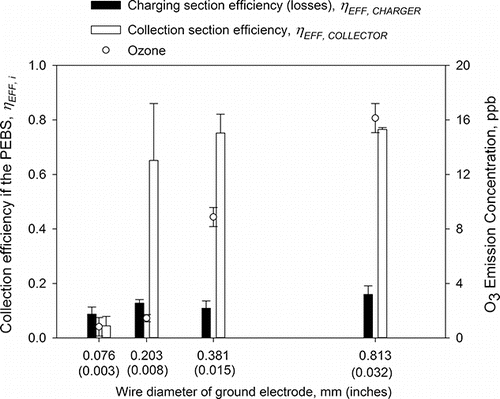
In the last step of PEBS optimization, we varied the length of the collection plate from 2.54 cm (1 inch) to 5.08 cm (2 inches), which resulted in the collection surface area, As, of 6.45, 8.06, 9.68, 11.29, and 12.90 cm2. The results are shown in . The surface collection area did not substantially affect the collection efficiency once it reached 8.06 cm2 (1.25 inches2). The collection section efficiencies for the surface areas of 8.06 cm2 and larger were not significantly different. They were all significantly higher than the collection efficiency at As = 6.45 cm2 (1.00 inch2) (p < 0.05). For further experiments, we chose the 9.68 cm2 (1.50 inch2) collection surface area because it had the same length (1.5 inches) as the ground electrodes and collection efficiency for this surface area had lowest COV (1.1%) among the investigated options.
Figure 6. The collection efficiency of the PEBS as a function of the collection electrode area. The experiments were performed with 1 µm PSL particles at a 10 L/min sampling flow rate and +5.5 kV/−7 kV charging/collection voltage. The second y-axis shows ozone emission concentrations by the PEBS with ozone background concentrations removed. Each data point is an average of least three repeats, and the error bars represent standard deviations.
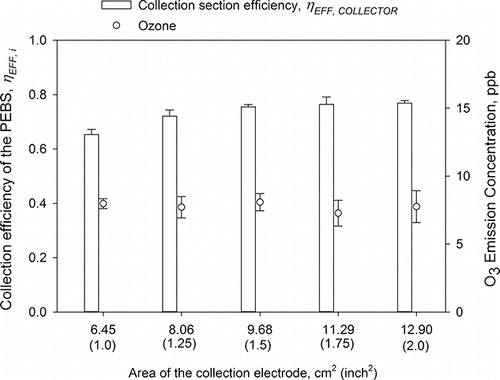
Thus, based on the results presented above, the PEBS, a two-stage bioaerosol collector, had the following parameters: (Equation1[1] ) the charging electrode was a tungsten wire 0.0762 mm (0.003 inches) in diameter and 2.54 cm (1 inch) in length, (Equation2
[2] ) the ground electrode in the charging section was a ring-type stainless steel wire 0.381 mm (0.015 inches) in diameter and 7.98 cm (3.14 inches) in length, (Equation3
[3] ) the collection electrode was a stainless steel plate (width: 2.54 cm [1 inch] × length: 3.81 cm [1.5 inches]) resulting in 9.68 cm2 (1.50 inch2) of surface area on one side, and (Equation4
[4] ) the ground electrode in the collection section was a quarter-cylindrical stainless steel plate with 7.61 cm2 (1.18 inch2) of surface area. The optimized charging voltages for PEBS were +5.5 kV at 10 L/min sampling flow rate, +6.5 kV at 20 L/min, +7.5 kV at 30 L/min, while −7.0 kV would be used for the collector.
presents a performance of the PEBS with those design and operational parameters as described in the previous section when collecting PSL of 0.026, 0.1, 0.2, 0.5, 1.0, and 3.1 µm in aerodynamic diameter (da). The average overall collection efficiency for the collection plate was 73.4 ± 4.9% over the entire range of tested particles and the average ozone emission concentration was 7.4 ± 1.4 ppb. The highest collection efficiency of 78.3 ± 1.3% was observed for 1 µm particles, while the average efficiency for smaller particles (0.5, 0.2, and 0.1 µm) was slightly lower. Because the number of charges acquired by particles is proportional to particle diameter squared (Hinds Citation1999), this decrease in efficiency could be expected as the particles get smaller. The average collection efficiency for the smallest 0.026 µm particles has increased (to 77.2 ± 3.0%) compared to 0.1–0.5 µm particles, most likely due to their greater diffusion (Hinds Citation1999) and high electrical mobility. The average collection efficiency for 3 µm PSL particles is also slightly lower than that for 1 µm particles. Our analysis of the particle deposition pattern inside the PEBS showed the increase of losses inside the charging section of the PEBS for 3.1 µm particles. However, the efficiency analysis by ANOVA showed that the collection section efficiency was not significantly affected by the particle size.
Figure 7. The collection efficiency of the PEBS as a function of PSL particle size (ranging from 0.026 to 3.1 µm). The experiments were performed with 1 µm PSL particles at a 10 L/min sampling flow rate and +5.5 kV/−7 kV charging/collection voltage. The efficiency was determined by measuring particle concentration downstream of PEBS with its voltage ON and OFF by a GRIMM OPC and P-Trak CPS. The second y-axis shows ozone emission concentrations by the PEBS with ozone background concentrations removed. Each data point is an average of least three repeats, and the error bars represent standard deviations.
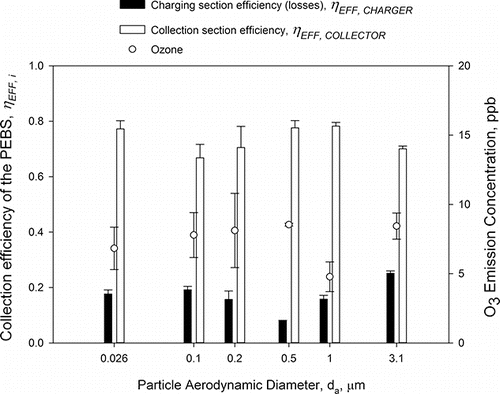
presents the performance of PEBS for three different sampling flow rates. An optimal charging voltage was used for each flow rate: +5.5 kV for 10 L/min, +6.5 kV for 20 L/min, and +7.5 kV for 30 L/min. The collection voltage was fixed at −7 kV for all three sampling flow rates. Because the charging voltage increased for increasing sampling flow rate, the ozone emission concentration increased from approximately 4.8 ppb at +5.5 kV and 10 L/min, to 11.9 ppb at +6.5 kV and 20 L/min, and then to 16.0 ppb at +7.5 kV and 30 L/min. The collection efficiency decreased with increasing sampling flow rate: 78.3 ± 1.3% at 10 L/min, 57.6 ± 3.0% at 20 L/min, and 40.2 ± 0.9% at 30 L/min (). This could be expected because the particles spent less time in charging and collection sections. The increase in ozone concentration was caused by the increase in charging voltage.
Figure 8. The collection efficiency of the PEBS as a function of sampling flow rate (10, 20, and 30 L/min). The experiments were performed with 1 µm PSL particles and 10 min sampling time. The charging voltage was different for each flow rate: +5.5 kV (10 L/min), +6.5 kV (20 L/min), and +7.5 kV (30 L/min), but the collection voltage was fixed at −7 kV. The collection efficiency was determined by measuring particle concentration downstream of PEBS with its voltage ON and OFF by GRIMM. The second y-axis shows ozone emission concentrations by the PEBS with ozone background concentrations removed. The third y-axis shows PEBS's concentration rate determined using Equation (Equation4[4] ). Each data point is an average of least three repeats, and the error bars represent standard deviations.
![Figure 8. The collection efficiency of the PEBS as a function of sampling flow rate (10, 20, and 30 L/min). The experiments were performed with 1 µm PSL particles and 10 min sampling time. The charging voltage was different for each flow rate: +5.5 kV (10 L/min), +6.5 kV (20 L/min), and +7.5 kV (30 L/min), but the collection voltage was fixed at −7 kV. The collection efficiency was determined by measuring particle concentration downstream of PEBS with its voltage ON and OFF by GRIMM. The second y-axis shows ozone emission concentrations by the PEBS with ozone background concentrations removed. The third y-axis shows PEBS's concentration rate determined using Equation (Equation4[4] ). Each data point is an average of least three repeats, and the error bars represent standard deviations.](/cms/asset/d0659288-9880-4644-992a-a1c0facd0b37/uast_a_1329516_f0008_b.gif)
also shows sampler's concentration rates, RC, based on the presented collection section efficiency, the 1 mL of elution liquid, and sampling flow rates of 10–30 L/min. Depending on the sampling flow rate, the concentration rates ranged from 7.8 × 103/min to 1.2 × 104/min for 1 µm PSL particles. If the volume of collection fluid could be decreased to 0.1 mL, then the concentration rate would increase by a factor of 10 exceeding values of 105/min. These calculations assume that particles are eluted from both sides of the collection plate.
In the latest part of the development, the collection efficiency of PEBS was determined when collecting 1 µm PSL particles for 10, 60, and 240 min at 10 L/min and the results are presented in . Here, we show the collection section efficiency ηEFF, COLLECTOR, determined using Grimm OPC and the actual collection efficiency, ηACTUAL, COLLECTOR, determined by comparing the number of particles deposited on the actual collection surface with that collected on the reference filter. Before setting out this test, it is important to mention that the two approaches to determine collection efficiency (Equations (Equation2[2] ) and (Equation3
[3] )) were compared and found to be within 0.6% (1 µm PSL particles at 10 L/min), 3.5% (1 µm at 30 L/min), and 9.2% (3 µm at 10 L/min). Concentrations of airborne PSL particles were ∼106–107/m3. As could be seen, the PEBS performance indicators determined by the two methods are very close: 82.4 ± 1.8% versus 81.7 ± 2.6%, 77.4 ± 5.4% vs. 76.6 ± 2.5%, and 73.3 ± 7.2% vs. 73.6 ± 4.1%, for 10, 60, and 240 min sampling time, respectively. The average values for the two parameters were approximately the same (78% vs. 77%) and not significantly different. For all three sampling times, the average ozone emission concentration was 8.6 ± 0.6 ppb. When the sampling time increased from 10 to 240 min, the collection efficiency decreased by approximately 10% on an absolute scale. However, the decrease was not significantly different. Also, for all sampling times, the actual collection efficiency was not significantly different from the collection section efficiency determined by OPC (p > 0.05).
Figure 9. The performance of PEBS determined by two different metrics as a function of sampling time (10, 60, and 240 min). The experiments were performed with 1 µm PSL particles at a 10 L/min sampling flow rate and +5.5 kV/−7 kV charging/collection voltages. The concentrations of test particles were ∼103–104/L. The second y-axis shows ozone emission concentrations by the PEBS with ozone background concentrations removed. Each data point is an average of least three repeats, and the error bars represent standard deviations.
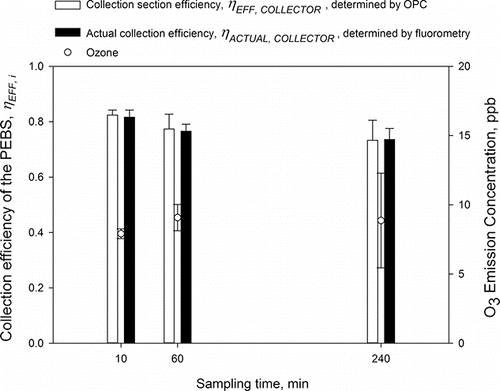
The resulting PEBS configuration described above (i.e., a wire-to-wire charger and collection chamber with a dual-sided electrode) was challenged with actual biological particles (i.e., B. atrophaeus and P. chrysogenum) at 10 L/min sampling flow rate and at +5.5/−7 kV charging/collection voltages. The ambient biological particles may carry native electrical charge, and it was shown in our earlier study (Yao and Mainelis Citation2006); however, due to relative high air velocity inside the PEBS at 10 L/min sampling rate, sampling of B. atrophaeus and P. chrysogenum without an extra charge added yielded the collection efficiency of only 6%–27%. Therefore, the biological particles had to be given extra charge, and the resulting collection efficiencies are presented in . The average collection efficiency for B. atrophaeus determined by an optical counter was 74.7 ± 1.1% (approximately 17% losses in the charging section), while for P. chrysogenum the collection efficiency was 69.2 ± 7.4% (about 24% losses in the charging section). This result was similar to our observation with similar sized PSL particles because the electrostatic force/drag force ratio is proportional to the particle diameter and charge level (Hinds Citation1999). The average ozone emission concentration was less than 7 ppb.
Table 1. Collection efficiency of the PEBS for B. atrophaeus bacteria and P. chrysogenum fungal spores when sampled at 10 L/min flow rates and at +5.5 kV/−7 kV charging/collection voltages. The data are presented as average ± standard deviation from at least three repeats.
The main focus of this work was to present a new personal sampler and to optimize its operating parameters (i.e., charging and collection voltages) and geometric parameters (i.e., ground wire diameter and collection plate area) so that the sampler could then be examined using airborne microorganisms. The data indicate that this personal sampler achieves ∼77% actual collection efficiency for sampling periods of up to 4 h while producing only 10 ppb of ozone. It is expected that this low ozone concentration will make the PEBS applicable for the collection of viable and culturable bioaerosols since ozone and nitrogen oxides might adversely affect the viability of airborne microorganisms (Cox Citation1987; Huang and Chen Citation2001). Thus, using the optimum sampling parameters determined in this study, we are currently experimenting with bacterial cells and fungal spores and investigating the application of various sample analysis methods, including epifluorescence microscopy, flow cytometry, quantitative polymerase chain reaction, adenosine triphosphate (ATP)-based bioluminescence, and culture-based methods.
Conclusions
A personal electrostatic bioaerosol sampler (PEBS) with a novel wire-to-wire charger successfully achieved collection efficiency exceeding 70% when challenged with a broad range of PSL particle sizes (26 nm to 3.1 µm) and two microorganism species. The device also showed steady performance during a long-term sampling period (up to 4 h). At the optimized operating voltages (+5.5/−7 kV for charging and collection) and geometric parameters (use of a ring made of stainless steel for the ground wire in the charger), the device produced low ozone emissions (less than 10 ppb). It is expected that the low ozone concentration will allow application of this sampler for the collection of the total as well as viable and culturable bioaerosols. We hope that the introduction of this personal sampler will promote innovation in the application of electrostatic tools for bioaerosol collection. The follow-up work will integrate the sampling section with power supplies, air movers, and operation controllers into a single wearable unit. The integrated sampler's performance will be investigated with various airborne biological agents in the laboratory and field settings. In addition, the sampler's compatibility with various microbiology analysis techniques will be explored.
Funding
The publication was supported by the grant R2110560 “Personal Electrostatic Bioaerosol Sampler (PEBS) with High Sampling Flow Rate” from CDC-NIOSH. Its contents are solely the responsibility of the authors and do not necessarily represent the official view of the CDC-NIOSH.
References
- Aizenberg, V., Reponen, T., Grinshpun, S. A., and Willeke, K. (2000). Performance of Air-O-Cell, Burkard, and Button Samplers for Total Enumeration of Airborne Spores. AIHAJ-Am. Ind. Hyg. Assoc., 61:855–864.
- Asefa, D. T., Langsrud, S., Gjerde, R. O., Kure, C. F., Sidhu, M. S., Nesbakken, T., and Skaar, I. (2009). The Performance of SAS-Super-180 Air Sampler and Settle Plates for Assessing Viable Fungal Particles in the Air of Dry-Cured Meat Production Facility. Food Control, 20:997–1001.
- Awad, M. B., and Castle, G. S. P. (1975). Ozone Generation in an Electrostatic Precipitator With a Heated Corona Wire. J. Air Pollut. Control Assoc., 25:369–374.
- Boelter, K. J., and Davidson, J. H. (1997). Ozone Generation by Indoor, Electrostatic Air Cleaners. Aerosol Sci. Technol., 27:689–708.
- Castle, G. S. P., Inculet, I. I., and Burgess, K. I. (1969). Ozone Generation in Positive Corona Electrostatic Precipitators. IEEE Trans. Ind. Gen. Appl., 5:489–496.
- Chang, C.-W., and Hung, P.-Y. (2012). Evaluation of Sampling Techniques for Detection and Quantification of Airborne Legionellae at Biological Aeration Basins and Shower Rooms. J. Aerosol Sci., 48:63–74.
- Chang, J. S., Lawless, P. A., and Yamamoto, T. (1991). Corona Discharge Processes. IEEE Trans. Plasma Sci., 19:1152–1166.
- Chen, J., and Davidson, J. H. (2002). Ozone Production in the Positive DC Corona Discharge: Model and Comparison to Experiments. Plasma Chem. Plasma Process., 22:495–522.
- Chen, J., and Davidson, J. H. (2003). Model of the Negative DC Corona Plasma: Comparison to the Positive DC Corona Plasma. Plasma Chem. Plasma Process., 23:83–102.
- Cookingham, C. E., and Solomon, W. R. (1995). 10 Bioaerosol-Induced Hypersensitivity Diseases. Bioaerosols, 2:205–234.
- Cox, C. S. (1987). The Aerobiological Pathway of Microorganisms. Wiley Interscience, Chichester, England, pp. 218–229.
- Dosemeci, M., Alavanja, M. C. R., Rowland, A. S., Mage, D., Zahm, S. H., Rothman, N., Lubin, J. H., Hoppin, J. A., Sandler, D. P., and Blair, A. (2002). A Quantitative Approach for Estimating Exposure to Pesticides in the Agricultural Health Study. Ann. Occup. Hyg., 46:245–260.
- Douwes, J., Thorne, P., Pearce, N., and Heederik, D. (2003). Bioaerosol Health Effects and Exposure Assessment: Progress and Prospects. Ann. Occup. Hyg., 47:187–200.
- Duquenne, P., Marchand, G., and Duchaine, C. (2013). Measurement of Endotoxins in Bioaerosols at Workplace: A Critical Review of Literature and a Standardization Issue. Ann. Occup. Hyg., 57:137–172.
- Eduard, W., Heederik, D., Duchaine, C., and Green, B. J. (2012). Bioaerosol Exposure Assessment in the Workplace: The Past, Present and Recent Advances. J. Environ. Monit.: JEM, 14:334–339.
- Francis, M., Sun, R., Cervelli, J. A., Choi, H., Mandal, M., Abramova, E. V., Gow, A. J., Laskin, J. D., and Laskin, D. L. (2017). Editor's Highlight: Role of Spleen-Derived Macrophages in Ozone-Induced Lung Inflammation and Injury. Toxicol. Sci., 155:182–195.
- Fung, F., and Hughson, W. G. (2003). Health Effects of Indoor Fungal Bioaerosol Exposure. Appl. Occup. Environ. Hyg., 18:535–544.
- Goheen, S. C., Larkin, E. C., and Bissell, M. G. (1984). Ozone Produced by Corona Discharge in the Presence of Water. Int. J. Biometeorol., 28:157–161.
- Gorner, P., Fabries, J.-F., Duquenne, P., Witschger, O., and Wrobel, R. (2006). Bioaerosol Sampling by a Personal Rotating Cup Sampler CIP 10-M. J. Environ. Monit., 8:43–48.
- Han, B., Hudda, N., Ning, Z., Kim, H.-J., Kim, Y.-J., and Sioutas, C. (2009). A Novel Bipolar Charger for Submicron Aerosol Particles Using Carbon Fiber Ionizers. J. Aerosol Sci., 40:285–294.
- Han, T., An, H. R., and Mainelis, G. (2010). Performance of an Electrostatic Precipitator with Superhydrophobic Surface when Collecting Airborne Bacteria. Aerosol Sci. Technol., 44:339–348.
- Han, T., Fennell, D., and Mainelis, G. (2015a). Development and Optimization of the Electrostatic Precipitator with Superhydrophobic Surface (EPSS) Mark II for Collection of Bioaerosols. Aerosol Sci. Technol., 49:210–219.
- Han, T., and Mainelis, G. (2008). Design and Development of an Electrostatic Sampler for Bioaerosols with High Concentration Rate. J. Aerosol Sci., 39:1066–1078.
- Han, T., O'Neal, D. L., McFarland, A. R., Haglund, J., and Ortiz, C. A. (2005). Evaluation of Mixing Elements in an L-Shaped Configuration for Application to Single-Point Aerosol Sampling in Ducts. HVAC&R Res., 11:657–672.
- Han, T., Zhen, H., Fennell, D. E., and Mainelis, G. (2015b). Design and Evaluation of the Field-Deployable Electrostatic Precipitator with Superhydrophobic Surface (FDEPSS) with High Concentration Rate. Aerosol Air Qual. Res., 15:2397–2408.
- Hewitt, G. W. (1957). The Charging of Small Particles for Electrostatic Precipitation. Trans. Am. Inst. Electr. Eng., Part I: Commun. Electron., 76:300–306.
- Hill, S. C., Pinnick, R. G., Niles, S., Pan, Y. L., Holler, S., Chang, R. K., Bottiger, J., Chen, B. T., Orr, C. S., and Feather, G. (1999). Real‐Time Measurement of Fuorescence Spectra From Single Airborne Biological Particles. Field Anal. Chem. Technol., 3:221–239.
- Hinds, W. C. (1999). Aerosol Technology. Wiley, New York.
- Huang, S.-H., and Chen, C.-C. (2001). Filtration Characteristics of a Miniature Electrostatic Precipitator. Aerosol Sci. Technol., 35:792–804.
- Johnson, B., Martin, D. D., and Resnick, I. G. (1994). Efficacy of Selected Respiratory Protective Equipment Challenged with Bacillus subtilis subsp. niger. Appl. Environ. Microbiol., 60:2184–2186.
- Kammer, R. (2005). The Microbial Killing Effect of Airborne Ozone. Examination Project Work. Department of Chemistry and Biomedical Sciences, University of Kalmar, Sweden, SE-391 82.
- Kenny, L. C., Bowry, A., Crook, B., and Stancliffe, J. D. (1999). Field Testing of a Personal Size-Selective Bioaerosol Sampler. Ann. Occup. Hyg., 43:393–404.
- Kesavan, J., and Sagripanti, J.-L. (2015). Evaluation Criteria for Bioaerosol Samplers. Environ. Sci.: Process. Impacts, 17:638–645.
- Koutsoubis, J. M., and MacGregor, S. J. (2000). Electrode Erosion and Lifetime Performance of a High Repetition Rate, Triggered, Corona-Stabilized Switch in Air. J. Phys. D: Appl. Phys., 33:1093.
- Kulkarni, P., Namiki, N., Otani, Y., and Biswas, P. (2002). Charging of Particles in Unipolar Coronas Irradiated by In-Situ Soft X-Rays: Enhancement of Capture Efficiency of Ultrafine Particles. J. Aerosol Sci., 33:1279–1296.
- Lin, G.-Y., Chen, T.-M., and Tsai, C.-J. (2012). A Modified Deutsch-Anderson Equation for Predicting the Nanoparticle Collection Efficiency of Electrostatic Precipitators. Aerosol Air Qual. Res., 12:697–706.
- Lindsley, W. G., Schmechel, D., and Chen, B. T. (2006). A Two-Stage Cyclone Using Microcentrifuge Tubes for Personal Bioaerosol Sampling. J. Environ. Monit. 8:1136–1142.
- Liu, B. Y. H., and Yeh, H. C. (1968). On the Theory of Charging of Aerosol Particles in an Electric Field. J. Appl. Phys., 39:1396–1402.
- Macher, J., Chen, B., and Rao, C. (2008). Field Evaluation of a Personal, Bioaerosol Cyclone Sampler. J. Occup. Environ. Hyg., 5:724–734.
- Macher, J. M. (1997). Evaluation of Bioaerosol Sampler Performance. Appl. Occup. Environ. Hyg., 12:730–736.
- Madsen, A. M., Kruse, P., and Schneider, T. (2006). Characterization of Microbial Particle Release from Biomass and Building Material Surfaces for Inhalation Exposure Risk Assessment. Ann. Occup. Hyg., 50:175–187.
- Madsen, A. M., Matthiesen, C. B., Frederiksen, M. W., Frederiksen, M., Frankel, M., Spilak, M., Gunnarsen, L., and Timm, M. (2012a). Sampling, Extraction and Measurement of Bacteria, Endotoxin, Fungi and Inflammatory Potential of Settling Indoor Dust. J. Environ. Monit., 14:3230–3239.
- Madsen, A. M., and Sharma, A. K. (2008). Sampling of High Amounts of Bioaerosols Using a High-Volume Electrostatic Field Sampler. Ann. Occup. Hyg., 52:167–176.
- Madsen, A. M., Tendal, K., Schlünssen, V., and Heltberg, I. (2012b). Organic Dust Toxic Syndrome at a Grass Seed Plant Caused by Exposure to High Concentrations of Bioaerosols. Ann. Occup. Hyg., 56:776–788.
- Mainelis, G., Adhikari, A., Willeke, K., Lee, S.-A., Reponen, T., and Grinshpun, S. A. (2002a). Collection of Airborne Microorganisms by a New Electrostatic Precipitator. J. Aerosol Sci., 33:1417–1432.
- Mainelis, G., Grinshpun, S. A., Willeke, K., Reponen, T., Ulevicius, V., and Hintz, P. J. (1999). Collection of Airborne Microorganisms by Electrostatic Precipitation. Aerosol Sci. Technol., 30:127–144.
- Mainelis, G., Willeke, K., Adhikari, A., Reponen, T., and Grinshpun, S. A. (2002b). Design and Collection Efficiency of a New Electrostatic Precipitator for Bioaerosol Collection. Aerosol Sci. Technol., 36:1073–1085.
- Mandal, J., and Brandl, H. (2011). Bioaerosols in Indoor Environment-A Review with Special Reference to Residential and Occupational Locations. Open Environ. Biol. Monit. J., 4:83–96.
- Murray, K. D., Gross, V. P., and Hobbs, P. C. D. (1992). Clean Corona Ionization. EOS/ESD Technology Journal, Jan:36–40.
- Nadkarni, M. A., Martin, F. E., Jacques, N. A., and Hunter, N. (2002). Determination of Bacterial Load by Real-Time PCR Using a Broad-Range (Universal) Probe and Primers Set. Microbiol., 148:257–266.
- Napoli, C., Tafuri, S., Montenegro, L., Cassano, M., Notarnicola, A., Lattarulo, S., Montagna, M. T., and Moretti, B. (2012). Air Sampling Methods to Evaluate Microbial Contamination in Operating Theatres: Results of a Comparative Study in An Orthopaedics Department. J. Hosp. Infect., 80:128–132.
- Nazaroff, W. W. (2016). Indoor Bioaerosol Dynamics. Indoor Air, 26:61–78.
- Niewulis, A., Podliński, J., Berendt, A., and Mizeraczyk, J. (2014). Influence of Electrode Geometric Arrangement on the Operation of Narrow Circular Electrostatic Precipitator. Int. J. Plasma Environ. Sci. Technol., 8:60–71.
- Nóbrega, S. W., Arnosti Jr, S., and Coury, J. R. (2001). Evaluation of the Performance of a Wire-Plate Electrostatic Precipitator. Braz. J. Chem. Eng., 18:313–325.
- Ohkubo, T., Hamasaki, S., Nomoto, Y., Chang, J. S., and Adachi, T. (1990). The Effect of Corona Wire Heating on the Downstream Ozone Concentration Profiles in an Air-Cleaning Wire-Duct Electrostatic Precipitator. IEEE Trans. Ind. Appl., 26:542–549.
- Park, J.-W., Park, C. W., Lee, S. H., and Hwang, J. (2015). Fast Monitoring of Indoor Bioaerosol Concentrations with ATP Bioluminescence Assay Using an Electrostatic Rod-Type Sampler. PLOS ONE, 10:e0125251.
- Pearson, C., Littlewood, E., Douglas, P., Robertson, S., Gant, T. W., and Hansell, A. L. (2015). Exposures and Health Outcomes in Relation to Bioaerosol Emissions From Composting Facilities: A Systematic Review of Occupational and Community Studies. J. Toxicol. Environ. Health. Part B, Crit. Rev., 18:43–69.
- Persoons, R., Parat, S., Stoklov, M., Perdrix, A., and Maitre, A. (2010). Critical Working Tasks and Determinants of Exposure to Bioaerosols and MVOC at Composting Facilities. Int.. J. Hyg. Environ. Health, 213:338–347.
- Plank, T., Jalakas, A., Aints, M., Paris, P., Valk, F., Viidebaum, M., and Jõgi, I. (2014). Ozone Generation Efficiency as a Function of Electric Field Strength in Air. J. Phys. D: Appl. Phys., 47:335205.
- Preller, L., Burstyn, I., De Pater, N., and Kromhout, H. (2004). Characteristics of Peaks of Inhalation Exposure to Organic Solvents. Ann. Occup. Hyg., 48:643–652.
- Roux, J. M., Sarda-Estève, R., Delapierre, G., Nadal, M. H., Bossuet, C., and Olmedo, L. (2016). Development of a New Portable Air Sampler Based on Electrostatic Precipitation. Environ.. Sci.. Pollut. Res., 23:8175–8183.
- Schachter, E. N., Maunder, L. R., and Beck, G. J. (1984). The Pattern of Lung Function Abnormalities in Cotton Textile Workers. Am. Rev. Respir. Dis., 129:235–245.
- Su, W.-C., Tolchinsky, A. D., Sigaev, V. I., and Cheng, Y. S. (2012). A Wind Tunnel Test of Newly Developed Personal Bioaerosol Samplers. J. Air Waste Manage. Assoc., 62:828–837.
- Tan, M., Shen, F., Yao, M., and Zhu, T. (2011). Development of an Automated Electrostatic Sampler (AES) for Bioaerosol Detection. Aerosol Sci. Technol., 45:1154–1160.
- Tsai, C.-J., Lin, G.-Y., Chen, H.-L., Huang, C.-H., and Alonso, M. (2010). Enhancement of Extrinsic Charging Efficiency of a Nanoparticle Charger With Multiple Discharging Wires. Aerosol Sci. Technol., 44:807–816.
- Viner, A. S., Lawless, P. A., Ensor, D. S., and Sparks, L. E. (1992). Ozone Generation in DC-Energized Electrostatic Precipitators. IEEE Trans. Ind. Appl., 28:504–512.
- Wu, Y., Shen, F., and Yao, M. (2010). Use of Gelatin Filter and BioSampler in Detecting Airborne H5N1 Nucleotides, Bacteria and Allergens. J. Aerosol Sci., 41:869–879.
- Xiangrong, Z., Lianze, W., and Keqin, Z. (2002). An Analysis of a Wire-Plate Electrostatic Precipitator. J. Aerosol Sci., 33:1595–1600.
- Yang, X.-F., Kang, Y.-M., and Zhong, K. (2009). Effects of Geometric Parameters and Electric Indexes on the Performance of Laboratory-Scale Electrostatic Precipitators. J. Hazard. Mater., 169:941–947.
- Yao, M., and Mainelis, G. (2006). Utilization of Natural Electrical Charges on Airborne Microorganisms for Their Collection by Electrostatic Means. J. Aerosol Sci., 37:513–527.
- Yao, M., and Mainelis, G. (2007). Analysis of Portable Impactor Performance for Enumeration of Viable Bioaerosols. J. Occup. Environ. Hyg., 4:514–524.
- Zhen, H., Han, T., Fennell, D. E., and Mainelis, G. (2013). Release of Free DNA by Membrane-Impaired Bacterial Aerosols Due to Aerosolization and Air Sampling. Appl. Environ. Microbiol., 79:7780–7789.
- Zhen, H., Han, T., Fennell, D. E., and Mainelis, G. (2014). A Systematic Comparison of Four Bioaerosol Generators: Affect on Culturability and Cell Membrane Integrity When Aerosolizing Escherichia Coli Bacteria. J. Aerosol Sci., 70:67–79.