Abstract
We have established a novel route for the synthesis of N-doped TiO2 by adopting flame aerosol (FSP) technique and investigated the effect of water content on the physico-chemical properties of the as-synthesized nanoparticles. The key characteristics of the developed method are to modify the precursor solution in order to incorporate nitrogen atoms into the TiO2 lattice without altering the FSP set-up. The reduction of the flame enthalpy resulting in N-incorporation into the TiO2 and the N-doping can be greatly enhanced further by the addition of secondary N-source (urea). Our XRD results reveal a shift of the (101) plane anatase diffraction peak to lower angles in our N-doped TiO2 compared to undoped TiO2, which suggest the distortion and strain in the crystal lattice prompted by the incorporation of the nitrogen atoms. The growth or expansion of crystal lattice can be attributed to the larger atomic radius of respective nitrogen atoms (r = 1.7 Å) compared to oxygen (r = 1.40 Å). Our XPS and EDX spectroscopy results elucidate that the nitrogen was effectively doped into the crystal lattice of TiO2 in our as-synthesized N-TiO2 catalysts predominantly in the form of interstitial nitrogen (Ti−O−N). The nitrogen atoms incorporation into the crystal lattice of titania modifies the electronic band structure of TiO2, resulting in a new mid-gap energy state N 2p band formed above O 2p valence band. This occurrence narrows the band gap of TiO2 (from 3.12 to ∼2.51 eV) in our N-doped TiO2 and shifts the optical absorption to the visible region.
Copyright © 2018 American Association for Aerosol Research
EDITOR:
1. Introduction
The most studied semiconductor is titanium dioxide (TiO2) for its utilization in the environmental processes comprising self-cleaning materials and as a photocatalyst in both aqueous and air streams for the oxidation of the volatile organic compounds (VOCs) (Fujishima and Honda Citation1972; Peral et al. Citation1997; Matthews Citation1990; Hoffmann et al. Citation1995; Ollis et al. Citation2010). Many efforts have been made to enhance the activity of the photocatalyst (TiO2) in solar radiation such as sensitization of TiO2 with organic dyes (Sun et al. Citation2003) and incorporate metal/metal oxides (Butler and Davis Citation1993; Di Paola et al. Citation2002; Anpo and Takeuchi Citation2003; Inturi et al. Citation2014). However, due to the leaching and photo-corrosion of the metal ions, alternative methods to improve photocatalytic activity of TiO2 are much needed (Marques et al. Citation2008; Inturi et al. Citation2016a, Citationb). Therefore, non-metal modified TiO2 is an attractive approach to enhance the photocatalytic activity under visible irradiation. Among the non-metals, nitrogen has emerged as a preeminent choice due to its stability, the similarity in atomic radius compared to oxygen and having lower ionization energy (Burda et al. Citation2003; Pelaez et al. Citation2012). Initially, Sato (Citation1986) reported lowering of the band gap energy by doping nitrogen ions into TiO2, but much attention was given towards developing N-modified TiO2 for solar driven photocatalysis (Devi and Kavitha Citation2013Citation; Tan et al. Citation2014) after the report by Asahi et al. (Citation2001). The presence of nitrogen can alter the band structure or suppress the recombination efficiency of the photogenerated electron-hole pairs, thus resulting in an enhanced photocatalytic capacity of TiO2 in the visible light region (Asahi et al. Citation2001).
So far, N-TiO2 has been prepared by using several synthesis methodologies. In particular, these synthesis methodologies can be categorized into two groups. Physical methods include implantation (Masahiko and Teruyoshi Citation2006), sputtering (Prabakar et al. Citation2007), and ball milling (Shifu et al. Citation2005). Chemical methods comprise of the direct hydrolysis (Shouxin et al. Citation2006), sol–gel method (Pomoni et al. Citation2008), hydrothermal synthesis (Wang et al. Citation2014) or solvo-thermal synthesis (Yang et al. Citation2010) including the oxidation of titanium nitride (Asahi et al. Citation2001).
Nevertheless, these synthesis methods are associated with numerous challenges for industrial implementations due to the low yield, the long processing time, high-energy consumption, large space requirement, and waste disposal. These drawbacks limit the large-scale application and scale-up of N-doped TiO2 for solar-driven photocatalytic degradation, whereas the flame aerosol syntheses method is an ingenious single-step synthesis method for producing a wide range of nanoparticles. In a typical aerosol technique, liquid precursor droplets are continuously sprayed into the flame and the oxidation of the precursor leads to the formation of metal oxide nanoparticles (Pratsinis Citation1998; Stark et al. Citation2001). Madler et al. (Citation2002), Jian et al. (Citation2009), Li et al. (Citation2016), and Hu et al. (Citation2017) reported the synthesis of porous TiO2 during the first-step, followed by stirring at 30 °C in 100 mL solution containing diluted HF for 40 h, and finally evaporating the solvents at 80 °C to attain F-doped TiO2. In a similar manner, Huo et al. (Citation2014) reported the preparation of near-surface modified TiO2 by flame synthesis. Nevertheless, that was a two-step process, in which TiO2 was prepared by flame synthesis followed by its surface modification by aqueous ammonia. However, the synthesis of N-doped TiO2 using one-step flame spray pyrolysis method has not been reported to date because the high enthalpy of the flame would result in the oxidation of nitrogen precursor into gaseous NOx.
In our current work, we have developed a novel synthesis of nitrogen-doped TiO2 nanomaterials by adopting a rapid one-step flame spray pyrolysis process for the first time (Smirniotis et al. Citation2018). We also investigated the effect of synthesis parameters on the N-doping into the TiO2 crystal lattice. We have developed this novel route of preparing the precursor solution, which has enabled us to incorporate relatively large amounts of nitrogen into the TiO2. Our single-step rapid aerosol synthesis method directs the nitrogen atoms mainly occupy interstitial positions in the TiO2 lattice. The increase in the nominal content of nitrogen in TiO2 resulted in the monotonic decrease of the bandgap energies from 3.11 eV to 2.51 eV. This pragmatic lowering of the band-gap energy for the aerosol N-doped TiO2 samples reveals that the nitrogen doping in TiO2 by flame spray pyrolysis technique is highly effective in extending the optical response of TiO2 in the visible region. Conversely, the increase in water content in the stock solution does not show significant impact on the band-gap energies reduction (from 2.55 eV to 2.52 eV). However, it had an effect on the crystallinity, surface area, and phase transformation of anatase to rutile.
2. Experimental
2.1. Synthesis method (FSP)
In the traditional FSP set-up, the liquid precursor was fed into the nozzle and atomized with high-flow dispersion gas in the nozzle around the precursor nozzle. The size of the aerosol droplet formed is a function of both the dispersion gas flow rate and viscosity of the precursor solution. A premixed flame (methane-oxygen) is employed to incinerate the formed atomized aerosol droplets due to the high-flame temperature (2400 K). The liquid precursors evaporate and undergo complete combustion leaving the metal/metal-oxide powder (Stark et al. Citation2001; Inturi et al. Citation2016a, Citationb). In order to synthesize N-doped TiO2 by the single-step flame spray pyrolysis (FSP), we have followed a systematic controlled procedure. Initially, we have added titanium tetraisopropoxide (TTIP) to diluted nitric acid followed by the addition of water, predetermined amounts of fuel (ethanol), and urea, respectively. We have observed a clear miscible homogeneous stock solution before spraying. We have given a schematic diagram of our aerosol reactor setup in . During the aerosol synthesis, the liquid precursor was fed through a spray nozzle at a flow rate of 3 mL min−1 using a syringe pump (Cole Parmer, 74900 series), where it was dispersed by a surrounding 5 L min−1 flow of oxygen gas (1.5 bar, Wright Brothers, 99.98%). Combustion of the dispersed droplets was ignited by a surrounding supporting flame (premixed 1.0 L min−1 oxygen/1.0 L min−1 methane). Additional 3 L min−1 sheath oxygen (BOC Gases) was supplied through the outer most sintered metal ring. The as-synthesized N-doped TiO2 aerosol nanoparticles leaving the flame are collected using a 150 mm diameter flat-filter (glass-fiber filter paper Whatman GF/A) supported by a vacuum pump (Madler et al. Citation2002; Li et al. Citation2016). The N-TiO2 aerosol nanoparticles were scraped from the filter for direct use as a catalyst without any further treatment. All the prepared materials are denoted as Y-XN-TiO2, where X is the molar concentrations of nitric acid (primary nitrogen source) and Y is the molar concentration of urea (secondary nitrogen source). The water percentages denoted as 25, 50, and 75% were associated with the molar concentrations.
2.2. Characterization
The surface textural properties such as BET surface area, pore diameter, and pore volume of the as-synthesized catalysts were measured from nitrogen adsorption equilibrium isotherms at 77 K (liquid nitrogen temperature) using an automated gas sorption system (Micromeretics ASAP 2010) operating in continuous mode. Before the analysis, 0.050 ± 0.01 g of TiO2 and N-doped TiO2 nanomaterials were evacuated under ultra-high purity helium atmosphere for 3 h at 250 °C in the degassing port of the instrument. Specific surface areas of the prepared catalysts (TiO2 and N-doped TiO2) were analyzed using the standard BET model. The Powder X-ray diffraction (XRD) profiles of the as-synthesized catalysts were measured by employing a Phillips Xpert diffractometer with nickel − filtered CuKα radiation source (wavelength 0.154056 nm) and a scintillation counter detector. The diffraction patterns data were recorded over a range of 10 − 80° (2θ) and 0.5 s step time. All the crystalline phases were acknowledged with respect to the reference data from International Center for Diffraction Data (ICDD) files. Shimadzu 2501PC UV–vis spectrophotometer was used to study the diffuse absorption of the light in the range of 200–900 nm. For this analysis, we used barium sulfate (BaSO4) as the background/standard. Bruker FRA 106/S Raman spectrometer (Germany) equipped with an InGaAs detector was employed for obtaining the Raman spectra at room temperature. Nd-YAG laser (1.5 W) radiation was used as the excitation source. Ti K-edge X-ray absorption spectra were recorded using the 7 C beamline at the Pohang Light Source (PLS) with a ring current of 120170 mA at 2.5 GeV.
TEM images of the selected as-prepared catalysts were recorded using an electron microscope (Philips CM 20). Prior to the analysis, flame made TiO2 and N-doped TiO2 catalysts were dispersed with the help of sonication in isopropyl alcohol and later moved onto carbon-Cu grid for the analysis. The 200 keV voltage with a LaB6 emission current and a point-to-point resolution of 0.27 nm are used to get the TEM images of the samples. The as-synthesized TiO2 and N-TiO2 aerosol nanoparticles were examined by X-ray photoelectron spectroscopy (XPS) experiments on a Pyris-VG thermo scientific X-ray photoelectron spectrometer system equipped with a monochromatic Al Kα (1486.7 eV) as a radiation source at 300 W under UHV (6.7 × 10−8 Pa). Sample charging during the XPS measurement of our flame made N-TiO2 samples was compensated by an electron flood gun. The electron lift-off angle was 45° with respect to the sample surface. The spectra were recorded in the fixed analyzer transmission mode with pass energies of 89.45 and 35.75 eV for recording survey and high-resolution spectra, respectively. The powdered aerosol nanoparticles were mounted onto the sample holder and evacuated overnight at room temperature at a pressure on the order of 10−7 Torr. The obtained N 1 s peaks of the as-synthesized materials were deconvoluted using Gaussian bands to identify several sub-bands with a 0.99 correlation coefficients (r2).
3. Results and discussion
The XRD (X-ray diffraction) patterns of aerosol made TiO2 and nitrogen-doped TiO2 catalysts are presented in . All the catalysts mainly exist in two main crystallographic forms, which are anatase (A) and rutile (R). For pristine TiO2, diffraction peaks located at 2θ = 25.4, 36.9, 37.8, 48.0, 54.2, 55.1, 62.6, 68.9, 70.1, and 74.9° correspond to the (101), (103), (004), (200), (105), (211), (204), (116), (220), and (215) planes of anatase titania (JCPDS card #84-1286), respectively. Furthermore, the diffraction peaks near 2θ = 27.5, 36.1, 41.1, 43.9, 56.6, 64.2, and 69.8° can be attributed to the (110), (101), (111), (210), (220), (002), and (301) planes, which can be well indexed to the rutile phase with a tetragonal structure (JCPDS card #75-1753). We observed similar diffraction patterns with a slight change in the peak positions for N-doped TiO2 samples. To investigate the effects of nitrogen doping on the anatase to rutile phase transformation, the fraction of rutile (XR) was calculated (Spurr and Myers Citation1957) from the characteristic peak intensities using the following Spurr and Myers equation (EquationEquation (1)[1] ):
[1]
Figure 2. Powder X-ray diffraction patterns of aerosol made TiO2, 0.8N-TiO2, 1-2N-TiO2, 2-1N-TiO2, and the effect of water content (25–75% in the precursor of 1-1N-TiO2).
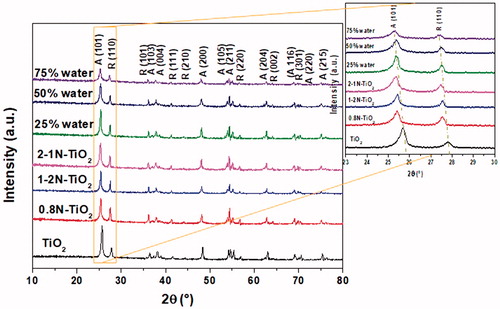
IA = diffraction peak intensity of anatase (101) plane and IR = diffraction peak intensity of rutile (110) plane of TiO2, respectively.
The comparative fractions of anatase phase (XA% = 100 − XR%) determined from EquationEquation (1)[1] are depicted in . As can be seen from , incorporation of nitrogen into the TiO2 crystal lattice has promoting effect on the phase transformation from anatase to rutile. We investigated the effect of both nitrogen sources (nitric acid and urea) on the structural properties of aerosol made N-TiO2 and compared with pristine TiO2 (). Initially, primary nitrogen source (HNO3) concentrations were selected as 0.8 M and 2.0 M. Furthermore, the primary nitrogen source (nitric acid) loadings were selected as 1.0 M and the secondary nitrogen source (urea) loadings were varied as 0.25 M and 2.0 M ().
Table 1. BET, XRD, UV-vis spectroscopy, and XPS characterization results of all the prepared samples.
Afterward, the secondary source (urea) loadings were selected as 1.0 M and the primary nitrogen source (nitric acid) loadings were varied ranging from 1.0 M to 2.0 M (). In addition, we also examined the effect of water concentration (namely 25%, 50%, and 75%) on the structural properties of TiO2. As we can observe from the magnified view, diffraction peaks corresponding to anatase (101) plane and rutile (110) planes in N-doped TiO2 samples slightly shifted to lower angles, which can be ascribed to distortion and strain in the crystal lattice triggered by the incorporation of the nitrogen atoms (Sun et al. Citation2008). Our diffraction peak shift results are in coordination with the calculated average size of titania crystal lattice (). The expansion or growth of crystal lattice can be ascribed to the larger atomic radius of corresponding nitrogen atoms (r = 1.71 Å) compared to oxygen (r = 1.40 Å). We can see the intensity of diffraction peaks of the as-synthesized 1-1N-TiO2 catalyst decreased with increase in the moisture content of the precursor from 5 to 75% (). In addition to the reduction in the diffraction peak intensity, we also observed a slight shift in the peak positions to lower angles compared to pure FSP TiO2. These results imply that our aerosol synthesis method directs nitrogen doping into the lattice of TiO2 and make changes in the crystal structure. The average particle size of the as-synthesized materials was determined using Scherrer's equation corresponding to the characteristic peaks of anatase phase (101) and rutile phase (110) and given in (Jenkins and Snyder Citation1996). It can also be seen from that the increase in water content of the precursor leads to the decrease in the average crystallite sizes due to the decrease in the flame temperature near the reactor zone. In a similar manner, the decrease in water content from 75% to 50% does not show much impact on the phase transformation of anatase to rutile, whereas further decrease in the water concentration to 25% demonstrated high impact (from 80.5% to 47.3% anatase), which can be ascribed to the increased flame temperature.
We also investigated the effect of nitrogen doping and the effect of the water content on the structure of TiO2 by Raman spectroscopy of the as-synthesized aerosol nanoparticles (). Raman scattering of aerosol made TiO2 and N-doped TiO2 samples prepared from primary and secondary N-sources are compared. To get insights into the effect of primary and secondary nitrogen contents on the structural properties of TiO2, we have compared 0.8N-TiO2, 1-2N-TiO2, and 2-1N-TiO2 samples with pristine TiO2. In a similar manner, we also examined the effect of water on the synthesis of N-doped TiO2 during the flame aerosol technique. As one can see from , we have observed five distinctive peaks near 141 cm−1 (Eg), 395 cm−1 (B1g), 514 cm−1 (A1g) and 637 cm−1 (Eg) phonon modes, which can be ascribed to the characteristics of the anatase phase. On the other hand, lower intensity Raman bands near 444 cm−1 of Eg character and near 607 cm−1 corresponding to A1g can be attributed to the rutile phase (Ohsaka et al. Citation1978; Tian et al. Citation2008; Lubas et al. Citation2014). It can be seen from that the intensity of the Raman bands has increased in the N-doped TiO2 samples compared to undoped TiO2. These results illustrate that the crystallinity has increased with the increase of the nitrogen doping into the crystal lattice of TiO2 and these results are inconsistent with our XRD results (). Conversely, the decrease in the water content of the precursor also led to the monotonical increase in the crystallinity of sample, which could be due to the increase of the flame temperature in reactor zone with higher content of fuel (ethanol) and lower content of water.
The specific surface area, pore size, and total pore volume of all the aerosol-made catalysts (TiO2, 0.8N-TiO2, 1-2N-TiO2, 2-1N-TiO2, as well as 1-1N-TiO2 with 25% water, 50% water, and 75% water) were determined from the analyses of BET measurements (). In , nitrogen adsorption/desorption isotherm curves of the investigated samples showed type III isotherms according to the international union of pure and applied chemistry (IUPAC) classification, with hysteresis loops of type H3 at a relative pressure range of 0.85–1.0. Type III isotherms illustrate the existence of mesopores. In theory, type III isotherms occur when the heat of adsorption is less than the adsorbate heat of liquification. On the other hand, the presence of slit-shaped pores and panel-shaped particles generates the H3 type hysteresis loops. Formation of type H3 loops indicates that the internal porosity of our aerosol-made nanoparticles is primarily slit-shaped and panel-shaped. These topographical features are favorable because they lead to a reduction in liquid diffusion.
Figure 4. N2 adsorption–desorption isotherms of as-synthesized TiO2, 0.8N-TiO2, and the effect of water content (25% to 75% in the precursor of 1-1N-TiO2.
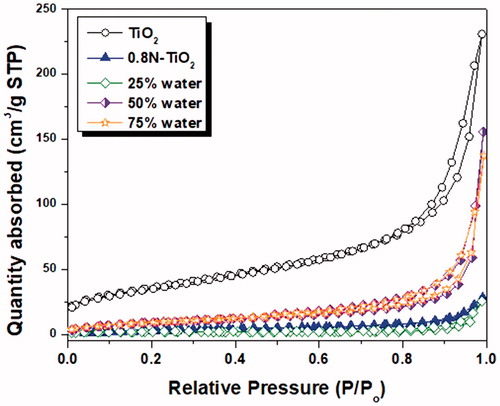
The UV–vis absorption spectra of the as-synthesized TiO2 and the N-doped TiO2 are shown in . In addition, the band-gap energies of the samples were measured from Kubelka–Munk plots and some of the plots were shown in (see the online supplementary information). Pristine TiO2 prepared by using flame spray pyrolysis is characterized by a sharp absorption edge at about 400 nm (Eg∼ 3.11 eV), whereas all the N-doped TiO2 samples shifted to the visible region and the optical band edge exhibited a remarkable red-shift with respect to that of pure TiO2. The increase of the nominal content of nitrogen in TiO2 resulted in monotonic decrease of the band-gap energies from 3.11 eV to 2.51 eV. This pragmatic lowering of the band-gap energy for the aerosol N-doped TiO2 samples reveals that the nitrogen doping in TiO2 by flame spray pyrolysis technique is highly effective in extending the optical response of TiO2 in the visible region. The nitrogen atoms incorporation into the crystal lattice of titania leads to the alteration of the electronic band structure of TiO2, thus resulting in a new mid-gap energy state N 2p band formed above O 2p valence band. This occurrence narrows the band gap of TiO2 (from 3.11 eV to ∼2.51 eV) in our N-doped TiO2 and shifts the optical absorption to the visible region. Conversely, the increase in water content in the precursor does not show significant impact on the band-gap energies (from 2.55 eV to 2.52 eV). However, it had an effect on the crystallinity, surface area, and phase transformation of anatase to rutile.
Figure 5. UV-vis diffuse reflectance spectra of as-prepared TiO2, 0.8N-TiO2, 1-2N-TiO2, 2-1N-TiO2, and the effect of water content (25–75% in the precursor of 1-1N-TiO2.
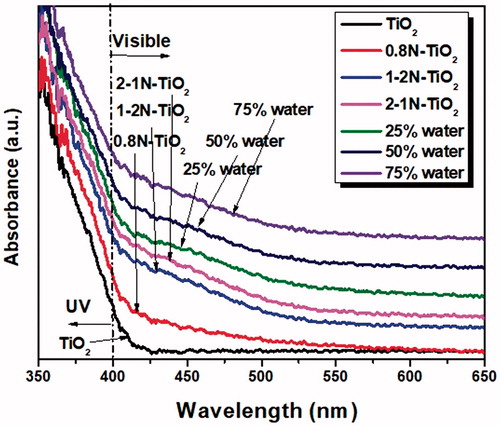
shows the transmission electron microscopy (TEM) images of the as-synthesized TiO2, and N-doped TiO2 materials along with the SAED patterns. In , pristine TiO2 comprises of inter-grown fundamental particles with a rough and uneven surfaces. The intergrowth of primary small particles results in aggregates with a significant extra framework void space, which is consistent with the textural mesoporous nature indicated in nitrogen adsorption isotherms. The SAED patterns of the pure titania particles shown in , illustrate the presence of (101), (004), (200), and (105) concentric diffraction rings of anatase TiO2 phase (Liu et al. Citation2012). Similar diffraction rings can be observed for N-doped TiO2 samples, representing the polycrystalline nature of the samples consistent with our XRD and Raman analysis. The topographical morphology and microstructure of hollow nitrogen-doped TiO2 spheres are evident in . The average diameter of the hollow N-doped TiO2 spheres is about 50–300 nm. The shell thickness is about 35 nm from the enlarged TEM image of a single hollow sphere in , which is in good agreement with the above result shown in the inset of TEM lattice image of the TiO2 nanoparticles taken from the shell of hollow N-modified TiO2 spheres. The inserts in illustrate the color change from white for pure FSP TiO2 () to light gray for 0.8N-TiO2 () with only nitric acid (primary N-source), whereas the color changed to light yellow in 1.0-2N-TiO2 () with the addition of 1.0 M urea (secondary source) and increasing the HNO3 concentration to 2.0 M (primary N-source). Further, the sample color reached a dark yellow in 2.0-1N-TiO2 () with increasing secondary N-source (urea) to 2.0 M.
Figure 6. Transmission electron microscopy (TEM) images of aerosol made (A) FSP TiO2, (B) 0.8N-TiO2, (C) 1-2N-TiO2, (D) 2-1N-TiO2, (E) 1-1N-TiO2 with 25% water, and (F) 1-1N-TiO2 75% water.
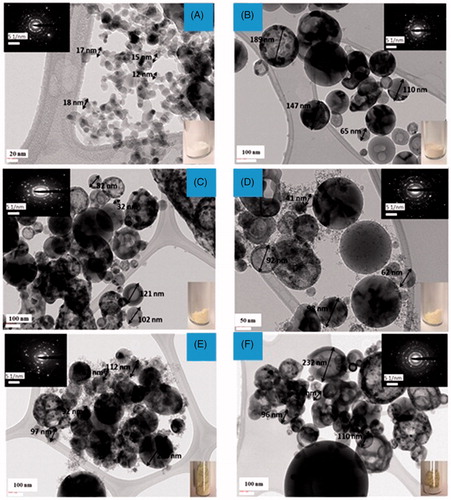
Further, we have investigated our aerosol made TiO2 and N-doped TiO2 samples by X-ray photoelectron spectroscopy (XPS) studies in order to find the nitrogen atomic concentration present in the as-synthesized nanoparticles ( and ). The N1s XPS spectra of all the prepared samples are presented in . The overlapped bands were fitted into three peaks at 401.7 eV, 399.2 eV, and 397.8 eV, which can be ascribed to the formation of substitutional and interstitial nitrogen atoms located in TiO2. In particular, the peak at 397.8 eV can be attributed to Ti-N bonding and it indicates that the nitrogen atoms are located at substitutional sites in the as-synthesized TiO2 crystal lattice. The second peak at 399.2 eV can be ascribed to O-Ti-N bonding, which reveals the formation of interstitial nitrogen atoms (Shifu et al. Citation2005; Devi and Kavitha Citation2013). The third peak at 401.7 eV can be indexed to the nitrogen present over the surface of the as-synthesized TiO2. Stated that the N 1 s peak at ≈400 eV binding energy is predominantly responsible for the visible induced photocatalysts. The relative atomic percentage of nitrogen in the as-synthesized aerosol N-doped TiO2 nanoparticles determined from XPS spectra were depicted in . As we see from the , 2-1N-TiO2 and 1-1N-TiO2 (with 75% water) samples demonstrated relatively high-atomic concentrations (1.62% and 0.97%) of nitrogen in TiO2, respectively. exhibits the influence of water concentration on the nitrogen doping. We observed a significant change in the nitrogen atomic concentration (from 0.43 to 0.96) with the increase in the water content (from 25% to 50%) in the precursor. Further increase in the water content (75%) in the precursor does not show much impact on the nitrogen concentration (). These results suggest that the optimal water content in the precursor is 50% to achieve higher N-doping in the TiO2.
4. Conclusions
In summary, we have successfully prepared N-doped TiO2 by adopting flame aerosol synthesis technique and investigated the effect of water concentration in the precursor solution. The appropriate properties of enhanced visible light absorption with abundant nitrogen content in the TiO2 frame structure allow these catalysts to become practical for large-scale real-world applications. Further, our research opens a strategic approach for developing efficient hole trapping centers on the semiconductors by grafting amorphous semiconductor nanoclusters and provides a simple and promising route for further improvement of the photocatalytic activities under visible light by coupling with hole trapping centers and electron trapping centers. High-flame temperature path, the formed spray precursor droplet rapidly evaporates resulting in the vapor phase combustion of the precursor. The combustion of the precursor vapor with high enthalpy results in the formation of atomic particles, which further nucleate and subsequently coalescence to form the aggregated small nanoparticles. Conversely, low-enthalpy path of the N-doped TiO2 containing 50% water in the precursor follows a precipitation route, causing a dominant intra-droplet reaction and resulting in the creation of larger dimension particles. Our flame spray pyrolysis method directs the nitrogen atoms to occupy predominantly interstitial positions in the TiO2 crystal lattice. The use of a secondary source of N in the form of urea increases significantly the level of nitrogen doping and reduces significantly the Eg of the synthesized materials. The presence of high amount of interstitial nitrogen can modify the band structure and suppress the recombination efficiency of the photo generated electron-hole pairs, thus resulting in the enriched photocatalytic activity of TiO2 under visible irradiation.
Supplemental Material
Download Zip (75.5 KB)Additional information
Funding
References
- Anpo, M., and Takeuchi, M. (2003). The Design and Development of Highly Reactive Titanium Oxide Photocatalysts Operating under Visible Light Irradiation. J. Catal., 216:505–516.
- Asahi, R., Morikawa, T., Ohwaki, T., Aoki, K., and Taga, Y. (2001). Visible-Light Photocatalysis in Nitrogen-Doped Titanium Oxides. Science, 293:269.
- Burda, C., Lou, Y., Chen, X., Samia, A. C. S., Stout, J., and Gole, J. L. (2003). Enhanced Nitrogen Doping in TiO2 Nanoparticles. Nano Lett., 3:1049.
- Butler, E. C., and Davis, A. P. (1993). Photocatalytic Oxidation in Aqueous Titanium Dioxide Suspensions: The Influence of Dissolved Transition Metals. J. Photochem. Photobiol. A: Chem., 70:273.
- Devi, L. G., and Kavitha, R. (2013). A Review on Nonmetal Ion Doped Titania for the Photocatalytic Degradation of Organic Pollutants under UV/Solar Light: Role of Photogenerated Charge Carrier Dynamics in Enhancing the Activity. Appl. Catal. B: Environ., 140–141:559.
- Di Paola, A., Garcı´a-López, E., Ikeda, S., MarcıÍ, G., Ohtani, B., and Palmisano, L., (2002). Photocatalytic Degradation of Organic Compounds in a Aqueous Systems by Transition Metal Doped Polycrystalline TiO2. Catal. Today, 75:87.
- Fujishima, A., and Honda, K. (1972). Electrochemical Photolysis of Water at a Semiconductor Electrode. Nature, 238:37–38.
- Hoffmann, M. R., Martin, S. T., Choi, W. Y., and Bahnemann, D. W. (1995). Environmental Applications of Semiconductor Photocatalysis. Chem. Rev., 95:69–96.
- Hu, Y., Jiang, H., Li, Y., Wang, B., Zhang, L., Li, C., Wang, Y., Cohen, T., Jiang, Y., and Biswas, P. (2017). Engineering the Outermost Layers of TiO2 Nanoparticles Using In Situ Mg Doping in a Flame Aerosol Reactor. AIChE J., 63:870–880.
- Huo, J., Hu, Y., Jiang, H., Hou, X., and Li, C. (2014). Continuous Flame Synthesis of near Surface Nitrogen Doped TiO2 for Dye-Sensitized Solar Cells. Chem. Eng. J., 258:163–170.
- Inturi, S. N. R., Boningari, T., Suidan, M. T., and Smirniotis, P. G. (2017). Novel synthesis of non-metal modified semiconductors by rapid flame spray pyrolysis method. invention disclosure submitted in United States Patents.
- Inturi, S. N. R., Boningari, T., Suidan, M., and Smirniotis, P. G. (2014). Transition Metal Modified TiO2 Catalysts for Visible- and UV-Light Driven Photodegradation of Aqueous Organic Pollutants. Appl. Catal. B: Environ., 144:333–342.
- Inturi, S. N. R., Boningari, T., Suidan, M., and Smirniotis, P. G. (2016a). Stabilization of Cr in Ti/SI/Cr Ternary Composites by Aerosol Flame Spray-Assited Synthesis of Visible Light Driven Photocatalysis. Ind. Eng. Chem. Res., 55:11839.
- Inturi, S. N. R., Suidan, M., and Smirniotis, P. G. (2016b). Influence of Synthesis Method on Leaching of the Cr-TiO2 Catalyst for Visible Light Liquid Phase Photocatalysis and Their Stability. Appl. Catal. B: Environ., 180:351–361.
- Jenkins, R., and Snyder, R. L. (1996). Introduction to X-Ray Powder Diffractometr. Wiley & Sons, Inc., New York.
- Jian, Z., Dieqing, Z., Zhenfeng, B., Guisheng, L., Yuning, H., Yunfeng, L., and Hexing, L. (2009). Aerosol-Spraying Synthesis of SiO2/TiO2 Nanocomposites and Conversion to Porous TiO2 and Single-Crystalline TiOF2. Chem. Commun., 36:5394–5396.
- Li, S., Ren, Y., Biswas, P., and Stephen, D. T. (2016). Flame Aerosol Synthesis of Nanostructured Materials and Functional Devices: Processing, Modeling, and Diagnostics. Prog. Energy Combust. Sci., 55:1–59.
- Limaye, A. U., and Helble, J. J. (2003). Effect of Precursor and Solvent on Morphology of Zirconia Nanoparticles Produced by Combustion Aerosol Synthesis. J. Am. Ceram. Soc., 86:273–278.
- Liu, J., Che, R., Chen, H., Zhang, F., Xia, F., Wu, Q., and Wang, M. (2012). Microwave Absorption Enhancement of Multifunctional Composite Microspheres with Spinel Fe3O4 Cores and Anatase TiO2 Shells. Small, 8:1214–1221.
- Lubas, M., Jasinski, J. J., Sitarz, M., Kurpaska, L., Podsiad, P., and Jasinski, J. (2014). Raman Spectroscopy of TiO2 Thin Films Formed by Hybrid Treatment for Biomedical Applications. Spect. Act. Part A: Mol. Biomol. Spectr., 133:867–871.
- Madler, L., Kammler, H. K., Mueller, R., and Pratsinis, S. E. (2002). Controlled Synthesis of Nanostructured Particles by Flame Spray Pyrolysis. Aerosol Sci., 33:369.
- Marques, F. C., Canela, M. C., and Stumbo, A. M. (2008). Use of TiO2/Cr-MCM-41 Molecular Sieve Irradiated with Visible. Catal. Today, 133–135:594–599.
- Masahiko, M., and Teruyoshi, W. (2006). Visible Light Photocatalysis of Nitrogen-Doped Titanium Oxide Films Prepared by Plasma-Enhanced Chemical Vapor Deposition. J. Electrochem. Soc., 153:C186–C189.
- Matthews, R. W. (1990). Purification of Water with Wear U.V. Illuminated Suspensions of Titanium Dioxide. Water Res., 24:653.
- Ohsaka, T., Izumi, F., and Fujiki, Y. (1978). Raman Spectrum of Anatase. TiO2. J. Raman Spectrosc., 7:321–324.
- Ollis, D., Pichat, P., and Serpone, N. (2010). TiO2 Photocatalysis-25 Years. Appl. Catal. B., 99:377.
- Pelaez, M., Nolan, N. T., Pillai, S. C., Seery, M. K., Falaras, P., Kontos, A. G., Dunlop, P. S. M., Hamilton, J. W. J., Byrne, J. A., and O'Shea, K. (2012). A Review on the Visible Light Active Titanium Dioxide Photocatalysts for Environmental Applications. Appl. Catal. B: Environ., 125:331.
- Peral, J., Domenech, X., and Ollis, D. F. (1997). Heterogeneous Photocatalysis for Purification, Decontamination, and Deodorization of Air. J. Chem. Technol. Biotechnol., 70:117.
- Pomoni, K., Vomvas, A., and Trapalis, C. (2008). Dark Conductivity and Transient Photoconductivity of Nanocrystalline Undoped and N-Doped TiO2 Sol Gel Thin Films. Thin Solid Films, 516:1271–1278.
- Prabakar, K., Takahashi, T., Nezuka, T., Takahashi, K., Nakashima, T., Kubota, Y., and Fujishima, A. (2007). Preparation and Photocatalytic Activity of TiOxNy/CdS Heterojunctions. J. Vac. Sci. Technol., A, 25:1188–1192.
- Pratsinis, S. E. (1998). Flame Aerosol Synthesis of Ceramic Powders. Prog. Energy Combust. Sci., 24:197–219.
- Sato, S. (1986). Photocatalytic Activity of NOx-Doped TiO2 in the Visible Light Region. Chem. Phys. Lett., 123:126.
- Shifu, C., Lei, C., Shen, G., and Gengyu, C. (2005). The Preparation of Nitrogen-Doped Photocatalyst TiO2 − xNx by Ball Milling. Chem. Phys. Lett., 413:404–409.
- Shouxin, L., Xiaoyun, C., and Xi, C. (2006). Preparation of N-Doped Visible-Light Response Nanosize TiO2 Photocatalyst Using the Acid-Catalyzed Hydrolysis Method. Chin. J. Catal., 27:697–702.
- Smirniotis, P. G., Boningari, T., Damma, D., and Inturi, S. N. R. (2018). Single-step rapid aerosol synthesis of N-doped TiO2 for enhanced visible light photocatalytic activity. Catal. Commun., 113:1–5.
- Spurr, R. A., and Myers, H. (1957). Quantitative Analysis of Anatase-Rutile Mixtures with an X-Ray Diffractometer. Anal. Chem., 29:760–762.
- Stark, W. J., Wegner, K., Pratsinis, S. E., and Baiker, A. (2001). Flame Aerosol Synthesis of Vanadia–Titania Nanoparticles: Structural and Catalytic Properties in the Selective Catalytic Reduction of NO by NH3. J. Catal., 197:182.
- Sun, H., Bai, Y., Jin, W., and Xu, N. (2008). Visible-Light-Driven TiO2 Catalysts Doped with Low-Concentration Nitrogen Species. Sol. Energy Mater Sol. Cells., 92:76–82.
- Sun, B., Vorontsov, A. V., and Smirniotis, P. G. (2003). Role of Platinum Deposited on TiO2 in Phenol Photocatalytic Oxidation. Langmui, 19:3151.
- Tan, H. Q., Zhao, Z., Niu, M., Mao, C. Y., Cao, D. P., Cheng, D. J., Feng, P. Y., and Sun, Z. C. (2014). A Facile and Versatile Method for Preparation of Colored TiO2 with Enhanced Solar-Driven Photocatalytic Activity. Nanoscale, 6:10216–10223.
- Tian, G. H., Fu, H. G., Jing, L. Q., Xin, B. F., and Kai, P. (2008). Preparation and Characterization of Stable Biphase TiO2 Photocatalyst with High Crystallinity, Large Surface Area, and Enhanced Photoactivity. J. Phys. Chem. C, 112:3083–3089.
- Wang, J., Fan, C., Ren, Z., Fu, X., Qian, G., and Wang, Z. (2014). N-Doped TiO2/C Nanocomposites and N-Doped TiO2 Synthesised at Different Thermal Treatment Temperatures with the Same Hydrothermal Precursor. Dalton Trans., 43:13783–13791.
- Yang, G., Jiang, Z., Shi, H., Xiao, T., and Yan, Z. (2010). Preparation of Highly Visible-Light Active N-Doped TiO2 Photocatalyst. J. Mater. Chem., 20:5301–5309.