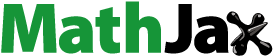
Abstract
Inhalation of aerosols containing pathogenic viruses can result in morbidity, in some cases leading to mortality. The objective of this study was to develop a model for assessing how infectious viruses might distribute in airborne particles using bacteriophage MS2 as a surrogate for human viruses. Particle deposition in the respiratory system is size-dependent, and small virus-containing particles can be inhaled deeply into the lower lungs, potentially leading to more severe respiratory disease manifestations. Laboratory-generated virus-containing particles were size-selected by a differential mobility analyzer and then collected by the newly introduced Super-Efficient Sampler for Influenza Virus. The number of infectious and total viruses per particle as a function of particle size varied with the spraying medium: it approximated a cubic exponential value scaling for deionized (DI) water, a quartic exponential value for artificial saliva (AS), and between quadratic and cubic exponential value for beef extract solution (BES). The survivability of MS2 did not change significantly with particle size for DI water and BES, while that for AS was maximum at 120 nm. Viruses could be homogeneously distributed or aggregated inside or on the surface of the particles, depending on the composition of the spraying medium.
Copyright © 2019 American Association for Aerosol Research
EDITOR:
Introduction
Exposures to pathogenic airborne viruses are associated with a wide range of diseases in humans, including the common cold, influenza, chickenpox, etc. (Leclair et al. Citation1980; Kutter et al. Citation2018). In 1918, a pandemic caused by an extremely virulent strain of influenza H1N1 virus resulted in 20–50 million deaths globally. Airborne viruses in breathing air have been found in a wide range of particle sizes. It is unclear, though, whether these viruses partition into all particle sizes, or are preferentially represented within a narrow size range.
Characterizing the distribution of infectious viruses in aerosols is of significant importance in understanding the transmission of respiratory viruses and for establishing effective public health countermeasures to restrict their spread, especially for the prevention of epidemics. For example, to prevent aerosol transmission of virus-containing particles <5 µm emanating from the coughs and expirations of an infected person, best practices would include housing of the patient in a negative-pressure isolation room and use of N95 respirators by attending health-care practitioners, rather than a surgical mask that is designed to block droplets of particles >5 µm in diameter emitted by the patient (Seto Citation2015). Moreover, due to their low settling velocity, smaller particles remain airborne for a longer time than larger ones, and they are transported farther distances by air currents; both prolong the risk for acquiring an infection through inhalation of infectious virus aerosols. For example, settling velocity increases from 3.48 × 10−5 m/s for 1 μm particles to 3.06 × 10−3 m/s for 10 μm particles (Hinds Citation1998). Furthermore, virus particles <200 nm are inhaled more readily down into the lower respiratory system than larger ones, and thus potentially result in a higher risk of lower respiratory tract infection (Tellier Citation2009).
Several studies were conducted to investigate the size distribution of virus-containing particles. Appert et al. (Citation2012) studied the size fractions of aerosols containing infectious MS2 phage and human adenovirus serotype-1 using an Andersen impactor and an 8-stage non-rotating micro-orifice uniform deposit impactor (MOUDI); the recovery of adenovirus showed dependence on aerodynamic particle size, while that of bacteriophage MS2 was independent of particle size. However, that study did not reveal the relationship between infectious virus and its carrier particle size: does a small particle or a large particle contain more infectious viruses? Such information is very important for the accurate assessment of the infectivity and transmissibility of virus aerosols and can provide a useful tool for the design of an effective strategy to protect the public from inhalation exposure.
Zuo et al. (Citation2013) found that the distribution of infectious bacteriophage MS2 was better represented by particle volume distribution rather than number distribution. However, their study only investigated particles larger than 100 nm and viruses were collected with a gelatin filter, which required follow-up extraction and recovery processes that might result in an underestimate of the number of infectious viruses collected and thus lead to inaccurate data analysis (Fabian et al. Citation2009). Compared with artificial saliva (AS) and bacterial cell culture medium (i.e., tryptic soy broth), human saliva seemed to be much less protective for bacteriophage MS2 (Zuo et al. Citation2014). Using size-selective sampling of MS2 aerosols (45, 90, 300 nm) produced with DI water as the nebulization media, Walls et al. (Citation2016) found that the number of virions per particle depended on the carrier particle size, following a volume-based size distribution, and among the three sizes studied, the survivability of the MS2 was generally constant. Since these studies either used samplers that are inefficient in recovering infectious viruses or used limited size resolution to allow statistical analysis (Walls et al. Citation2016; Zuo et al. Citation2013), comprehensive studies that include sampling of a wide size range of particles with a reliable virus aerosol sampler will provide valuable information that will provide a better understanding of the infectivity of airborne viruses as a function of its carrier size and composition.
The recently described VIable Virus Aerosol Sampler (VIVAS), which is based on water-based condensation to enlarge particle size, has been applied for the collection of laboratory-generated aerosols containing bacteriophage MS2 and influenza A virus, and naturally occurring airborne viruses in a student infirmary (Lednicky et al. Citation2016; Pan et al. Citation2016, Citation2017). All things otherwise equal, the collection efficiency of the VIVAS is more than 10 times higher for laboratory generated aerosols of infectious MS2 (Pan et al. Citation2016) and seven times higher for the infectious H1N1 influenza viruses (Lednicky et al. Citation2016) than that for the industry standard device, the BioSampler®. Moreover, the detection limit of the VIVAS is much lower than that of the BioSampler for bacteriophage MS2 (Lednicky et al. Citation2016). Operationally, the VIVAS mimics what happens upon inhalation of aerosols into human lungs on a cold day by (a) introducing cold aerosol particles into a warm growth tube saturated with water vapor, resulting in (b) encapsulation of small particles into larger droplets. The combination results in efficient collection of the enlarged particles through gentle impaction in a manner that preserves the viability of the collected virus particles (Hering and Stolzenburg Citation2005; Hering et al. Citation2005).
The objective of this study was to determine the size distribution of lab-generated infectious MS2 phage-containing particles as a function of the spraying medium, so as to provide supporting information for investigating distribution of infectious viruses in the real world. Virus infectivity was assessed by plaque assays, and virus quantification regardless of viability was accomplished by reverse transcription quantitative polymerase chain reaction (RT-qPCR).
Material and methods
Virus aerosol sampler
The water-based “Super-Efficient Sampler for Influenza Virus” (SESI) used in this study (Figure S1) is the second generation of the VIVAS (Lednicky et al. Citation2016; Pan et al. Citation2017). It contains eight parallel growth tubes with a total flow rate of 8.5 liters per minute (lpm), and has four main sections, namely the conditioner, initiator, moderator and collector. The difference between the SESI and the VIVAS is that the SESI incorporates a “moderator” stage to limit the water vapor content of the aerosol exiting the growth region, and a temperature control on the collector to allow collection at different temperatures and enable control over the volume of collected liquids. The advantages of this modified design are that temperature of the collector can be chosen and water accumulation or evaporation in the collection part can be avoided, enabling high survivability of the viruses.
For this work, the conditioner was held at 5 °C, and the initiator was maintained at 40 °C to activate condensational growth for particles as small as 10 nm. The moderator, which reduces excess water vapor entering the collector, was held at 10 °C. After condensation occurred, particles traveled through 24 nozzles (3 per growth tube) to impinge onto 1.5 ml of collection fluid. The temperature of the nozzles was kept at 22 °C to eliminate water condensation, while the temperature of the collection fluid was held at 5 °C.
Test virus
Bacteriophage MS2 has a single-stranded RNA genome, an approximate diameter of 27.5–30 nm, and only infects male Escherichia coli cells (Golmohammadi et al. Citation1993). Freeze-dried MS2 (Cat. #15597-B1) from the American Type Culture Collection (ATCC, Manassas, VA, USA) was re-suspended in sterile deionized (DI) water to a titer of 1014–1015 plaque-forming units (PFU) ml−1, and aliquots were stored at −80 °C. Immediately before aerosol generation, aliquots of MS2 were diluted to a titer of 108–109 PFU ml−1 with DI water, beef extract solution (BES), or AS.
Experimental design and procedure
A schematic of the experimental sampling system is shown in Figure S2. Aerosol containing MS2 was generated using a six-jet Collison nebulizer (Model CN25, BGI Inc., Waltham, MA, USA) operated at 3.5 lpm. A TSI Aerodynamic Particle Sizer (APS) model 3321 (TSI, Inc., Shoreview, MN, USA) was used to measure particle size distribution exiting the Collison nebulizer. The aerosol flow was then combined with dry air at 5 lpm in a dilution dryer to remove excess moisture before it flowed onto moving to the next compartment.
To determine the particle size distribution, the aerosol flow exiting the dilution dryer was directed to a Scanning Mobility Particle Sizer (SMPS), which consists of a Differential Mobility Analyzer (DMA, model 3081, TSI Inc.), and Condensation Particle Counter (CPC, model 3010, TSI Inc.). The DMA selects particles based on their electrical mobility controlled by a supplied voltage. The CPC counts the monodisperse particles exiting the DMA. The tested particle sizes included 30, 60, 90, 120, 150, 200, and 300 nm. Thirty-nanometer particles correspond to the diameter of singlet naked MS2 particles while 300 nm particles comport to the cutoff size of many bioaerosol samplers, such as that for the BioSampler running at the recommended flow rate (Hogan et al. Citation2005).
To determine the infectious virus size distribution and survivability of infectious virus aerosol, after aerosol flow passed through the dilution dryer, the DMA was used for size selection and thereafter the SESI for sampling size-selected aerosol. The SESI was operated at 8.5 lpm and all collections had a sampling time of 15 min. The collection fluid was used for both plaque assay and RT-qPCR analysis. Finally, the exhaust gas flow was passed through a high-efficiency particulate air (HEPA) filter before discharging to the exhaust hood.
Spraying media
The three spraying media tested were DI water, BES, and AS. DI water was used to explore properties of the virus particles that are not complexed with extraneous materials. BES has traditionally been used to emulate components that might occur in a naturally produced complex aerosol, such as peptides, amino acids, nucleotide fractions, organic acids, minerals, and vitamins. AS, a dilute fluid that mimics human saliva, is composed of more than 98% water, and contains electrolytes, proteins, and enzymes (Aps and Martens Citation2005; Diaz-Arnold and Marek Citation2002; Woo et al. Citation2010, Citation2012; Woo Citation2012). A concentration of 0.3% (w/v) mucin was chosen for AS in this study to mimic the protein content in human saliva so that the AS and human saliva would have similar rheological properties, i.e., viscosity and elasticity (Veerman et al. Citation1996). During the preparation of AS, we confirmed its properties changed upon autoclaving (as performed by others). Physical size distributions of the aerosolized AS without autoclaving and with autoclaving (AS-AC) were measured using the SMPS, whereas only AS without autoclaving was used for further studies of infectious and total size distributions to better approximate in vivo conditions.
Sample analysis
The same collection and spraying media were used for enumerating infectious MS2. Following collection, the samples were serially diluted for single-layer bioassay onto tryptone agar petri dishes (Berg et al. Citation1984). Briefly, 300 µl of serially diluted samples were added to 9 ml of tryptic yeast agar maintained at 48 °C along with 300 µl of log-phase E. coli. The petri dishes were incubated at 37 °C overnight, and plaque forming units (PFU) counted.
RT-qPCR provides a mechanism for determining total virus (both infectious and non-infectious) compared to only infectious virus as determined by plaque assay. RNA extraction for RT-PCR analysis was accomplished using a QIAamp Viral RNA mini kit (Cat#52904) per manufacturer’s recommendations, with one modification: RNA was retrieved from the silica columns using two 40 μl elutions in the final step, instead of one 60 μl elution step. One-step qPCR was carried out on a Bio-Rad CFX 96 qPCR (see the online supplementary information [SI]).
Data analysis
The size distributions of the MS2 by number or volume as a function of particle size aerosolized with different spraying media are determined by dividing the number or volume of particles (within the particle size bin measured by the SMPS) by the logarithm of the particle size bin of the DMA. They are shown in as the left vertical axis dN/dlogdp and the right vertical axis dV/dlogdp, respectively.
Figure 1. Particle size distributions by number and by volume obtained from the SMPS, infectious MS2 size distribution obtained through plaque assay, and total MS2 size distribution obtained from RT-qPCR, for three aerosolized suspension media: (a) DI water, (b) BES, and (c) AS. All the data points measured with SMPS were repeated four times. Data points of DI water for both infectious and total MS2 were triplicates, while for BES and AS, data points were repeated twice. Standard deviations were calculated and shown in the figures as error bars.
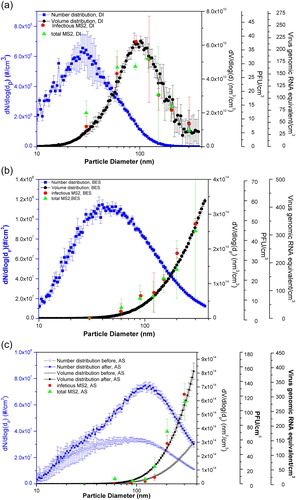
To determine the infectious virus size distribution as a function of particle size, defined as as shown in the right vertical axis PFU/cm3, the following EquationEquation (1)
(1)
(1) was used:
(1)
(1)
where is the virus titer in the collection liquid sample over time t (min),
is the liquid volume in the collection sampler over time t, and
is the aerosol flow rate into the sampler, Δlogdp is the logarithm of the particle size bin of the DMA. By replacing
with
which is the virus genomic RNA equivalent in the collection liquid sample over time t, the total virus size distribution within the size range of the DMA as a function of particle size can be obtained, as shown in the right vertical axis virus genomic RNA equivalents/cm3.
To determine the number of infectious MS2 per particle (NPFU) in the selective particle size bin, EquationEquation (2)(2)
(2) was used:
(2)
(2)
where
is the number concentration of aerosol particles within a particle size bin measured by the CPC. Similarly, the MS2 genomic RNA equivalent per particle (NRNA) in the selective size bin was determined by EquationEquation (3)
(3)
(3) :
(3)
(3)
The survivability of the MS2 can be obtained from the ratio of infectious MS2 to total MS2, i.e., as NPFU/NRNA or CPFU/CRNA.
Data were statistically analyzed using 95% confidence level, z-test, and one-way analysis of variance (ANOVA). A p-value of less than 0.05 was considered statistically significant.
Results and discussion
Particle size distribution
The count modes (peak number) of the aerosolized particles from different suspension media (DI water, BES, AS, and AS-AC) measured by the SMPS are shown in Table S1, also as displayed in . The count mode of aerosolized MS2 with DI water (28.5 nm) as the suspension medium corresponded to the size of singlet naked MS2, whereas there was no correlation between the size of the singlet naked MS2 with other types of suspension media. After evaporation of water during the dilution process, MS2 aerosols generated with DI water were composed of mainly MS2 alone. Therefore, particle size distribution generated with DI water best reflected the particle sizes of singlet naked MS2 virions and their aggregates. For AS and BES as the spraying media, after evaporation of water, particles were comprised of MS2 and the solid components of the suspensions. Compared with those solid components, MS2 virions only accounted for a small portion. This can be seen from the differences in the left vertical scales of as those of BES and AS are much higher than that of DI water. Thus, particle size distributions generated with BES and AS were dictated by the solid components of the suspensions. For non-autoclaved AS, particle size distribution was measured immediately after aerosolization or 2 h after continuous aerosolization by the Collison nebulizer; the count mode shifted from 90 nm at the beginning to 120 nm 2 h later. This is consistent with the study of Zuo et al. (Citation2014), which suggests that shear stress imposed by the nebulization process might break up entanglements of the proteins, thus leading to different size distributions generated over time.
Autoclaving of the AS had a significant effect on the particle size distribution, as the count mode measured by the SMPS after autoclaving was constant throughout the experiment, at around 45 nm. The likely reason is that mucin (the protein in AS), which is composed of large glycoproteins with long chain structures that tend to entangle (Bansil and Turner Citation2006), is dissociated into units (monomers) during the autoclaving process (Rehman and Shah Citation2005). Subsequently, those proteins can decompose to form smaller peptides, leading to smaller sized particles. Our results are consistent with Zuo et al. (Citation2014), which showed that size distribution of AS without mucin is stable during the experiment.
Virus size distribution as a function of nebulization suspension
The particle size distributions by number and volume, infectious virus size distribution, and total virus size distribution generated using three nebulizer suspensions, are shown in . For aerosols generated with DI water (), the infectious and total virus concentrations were observed to increase with increasing particle size, reaching a maximum concentration of infectious viruses of 37 PFU/cm3 at 95 nm for infectious viruses, and total virus genomic RNA concentration of 210 genome equivalents/cm3 at 120 nm for total virus genomic RNA; both measures thereafter decreased as particle size increases. Overall, both the infectious and total virus size distributions better followed the particle size distribution by volume rather than by number. Similar trends were also observed for other types of suspensions, as shown in . These results are similar to previous results obtained for particles collected with gelatin filter or a condensation growth tube with TSB, human saliva, and AS as the spraying media (Walls et al. Citation2016; Zuo et al. Citation2014). In the size range investigated (30–300 nm), the infectious and total MS2 concentrations increased with the increase of the particle sizes for droplets nebulized with BES and AS (), as reported from previous studies (Zuo et al. Citation2013). However, the infectious and total MS2 concentrations aerosolized with DI water increased up to 100 nm and then decreased relative to further increases of particle sizes due to low volume concentration of aerosolized particles above that size. This trend for DI water as suspension medium has not been reported in previous studies, perhaps due to the limited size range used in those studies (Walls et al. Citation2016; Zuo et al. Citation2014). For all the nebulizer suspensions, all total virus number counts were greater than the infectious virus number counts; these differences suggest that viruses were inactivated during the nebulization, transportation, or sampling processes. However, the infectious virus concentration were about the same in the nebulizer before and after aerosolization (Zuo et al. Citation2014).
Virus and genomic RNA equivalent per particle as a function of particle diameter and nebulization suspension
The infectious and total virus counts per particle as a function of particle diameter are shown in . Data points at 300 nm for DI water were excluded in the data analysis due to the large error caused by the small amount of particles collected (<10 #/cm3). For AS as the nebulization media, data points at 60 nm were also excluded in the linear regression and data analysis, due to their large error, probably caused by the heterogeneous nature of the AS suspension (to be explained in next subsection) and low probability of the existence of the virions in the dominant AS components in small particles. The average of the total particle concentrations measured at the beginning and 2 h later was used for AS for the following calculation. Regardless of the nebulization suspension, both infectious and total virus counts per particle increased with particle size. Results of the fitting curve indicated that the associated exponential values, which indicate how the distribution of infectious viruses changes with particle size, were not significantly different for the infectious viruses measured by culture methods and the total viruses measured by the RT-qPCR for each of the nebulizing suspensions, except for the AS; p-values are 0.74 for DI water, 0.53 for BES, and 0.009 for AS.
Figure 2. Infectious and total MS2 per particle generated in different suspension media as a function of aerosol particle size: (a) DI water, (b) BES, and (c) AS.
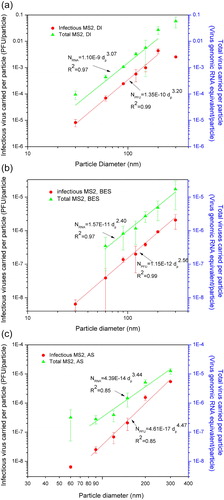
Although the infectious and total MS2 concentrations visually follow the volume size distribution as shown in , further analyses of these data reveal that the associated exponential values for infectious and total MS2 per particle also depended on the type of suspension medium. For different suspension media, the exponential values changed from 3.20 (95% confidence: 2.74–3.66) for infectious and 3.07 (95% confidence: 2.07–4.06) for total MS2 aerosolized from DI water, to 2.55 (95% confidence: 2.31–2.81) for the infectious MS2 and 2.41 (95% confidence: 1.86–2.98) for the total MS2 aerosolized from BES, and then to 4.47 (95% confidence: 3.75–5.17) for the infectious MS2 and 3.44 (95% confidence: 2.31–4.55) for the total MS2 aerosolized from the AS. In other words, the size distributions of infectious and total viruses were not proportionate to the particles’ volume. Our results agree well with prior studies that the exponential values for virus aerosols generated from AS is higher than 3 and the exponential values for MS2 aerosols generated from AS without mucin is slightly lower than from AS (Zuo et al. Citation2014). shows that no or very few viruses existed at 30 nm for BES and AS as the suspension media, which suggest that most viruses aggregate or are associated with other components of the spraying media. also shows that the amount of infectious viruses per particle is always lower than the total viruses per particle.
Survivability of MS2
Survivability of the viruses as a function of particle diameter is shown in . Survivability, expressed as the ratio of infectious viruses/total counts, was always less than 1 for all the nebulization media tested. For DI water and BES, the survivability of MS2 did not change significantly with particle size (p > 0.05), with the survivability less than 0.2 for almost all particle sizes. In contrast, the survivability of MS2 varied with particle size for AS, with the maximum survivability of 0.82 for 120 nm particles. Survivability was very low at the size of 60 nm.
Figure 3. Survivability of MS2 as a function of particle diameter for different nebulization suspensions. Note: DI excluded and AS excluded are the data points that were not taken into analysis in the “Virus and genomic RNA equivalent per particle as a function of particle diameter and nebulization suspension” section. Data points of DI water for both infectious and total MS2 were triplicates, while for BES and AS, data points were repeated twice. Standard deviations were calculated and shown in the figures as error bars.
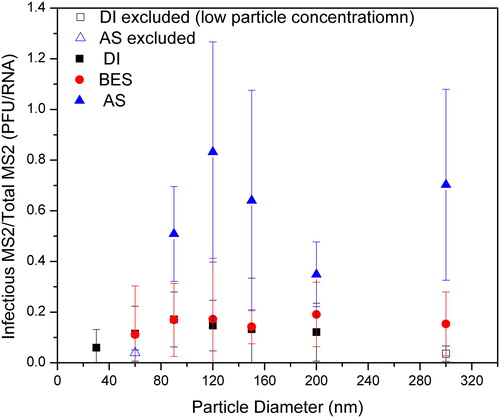
The current experimental set-up does not identify where these viruses get deactivated within the system. All processes, including nebulization, transport, classification, and sampling, could lead to the deactivation of the viruses. However, the survivability of MS2 with AS as the suspension media varied with particle size, and the survivability value was relatively larger than the DI water and BES (p < 0.0001); the difference suggests that the protective effect of the AS, mucin in particular, is better than that of BES and DI water (no protection for viruses; p = 1 for DI and BES). One possible reason is the shielding offered by the solid parts of the AS that protects MS2 from deactivation but yet unspecified means or breakage of the virions into subcomponents. For the deactivation of bacteriophage MS2, the most plausible explanation is the loss of viability after exposure to the air-water interface (AWI), where irreversible rearrangement and folding of the viruses’ protein take place (Thompson and Yates Citation1999; Trouwborst and De Jong Citation1973; Trouwborst et al. Citation1974; Zuo et al. Citation2014). Since MS2 is relatively hydrophobic, it tends to be attracted to the AWI, and that leads to deactivation. The addition of peptone and other components may reduce surface tension, and thus reduce the quantity of MS2 that partition into the AWI (Adams Citation1948; Trouwborst and De Jong Citation1972). Different types of mucins exist in saliva, and their functions include protection of oral tissues against dehydration and chemically induced injuries, and they can form aggregates or form complexes with other biomolecules (Bansil and Turner Citation2006; Veerman et al. Citation1996). Hence, in the presence of mucin, AS may aggregate, trap, and hydrate more MS2 particles inside the particles, and subsequently reduce exposure to the AWI compared with DI water and BES used as nebulizing media. The low survivability of infectious MS2 at 60 nm might be due to absence of mucin in small particles, as mucin is composed of large glycoprotein molecules that are less likely to exist at small particle sizes.
illustrates possible types of distribution of virions within an aerosolized particle for different suspension media. Once aerosolized, the generated droplets would initially carry substantial amount of water with some solid components of the suspension media. These droplets thereafter lose water in the dilution drier before entering the classifier. If the infectious and total virus concentrations follow size distribution by number, virus distribution is independent of particle size, as shown in (power law factor = 1). However, if the infectious and total virus concentrations follow size distribution by surface, the virus tends to disperse on the surface of the particles as shown in (power law factor = 2). If the virus concentration follows size distribution by volume, the virus would disperse homogeneously inside the particles, as shown in (power law factor = 3). If the exponential values for infectious and total MS2 per particle are larger than 3, viruses might be aggregated together inside the particles as shown in .
Figure 4. Conceptual illustration of various distribution patterns of virions inside aerosolized particles with respect to power law factor of (a) 1, (b) 2, (c) 3, and (d) >3 (cross-sectional view of the particles). Three particle sizes are presented (evolving from the smaller to larger), and the smallest spheres represent virions.
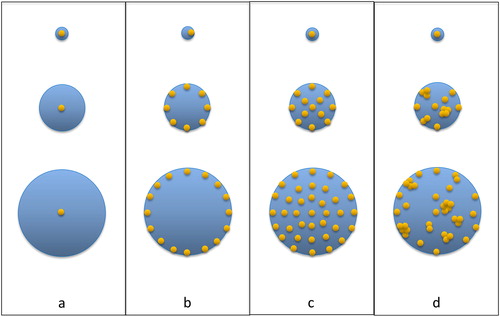
For DI water, only viruses, virus debris, or residual growth media were left after passing through the dilution drier. If no empty space existed inside virus-containing particles after dilution, MS2 or debris could only aggregate together to comprise larger particles, more likely following the size distribution by volume, as depicted in but without empty spaces; 3 is within the 95% confidence level of the power law factor. For BES, the virus size distribution inside of the particles is between . The MS2 size distribution inside the BES depends more on the relative hydrophobic or hydrophilic interaction between the BES and MS2. The extraction process for preparing beef extract is complex, and the preparation process also affects its hydrophilic or hydrophobic nature. If MS2 is more hydrophobic than the BES, MS2 will be attracted to the surface of the particles, and therefore be deactivated through AWI. However, if the BES is more hydrophobic than MS2, the BES will move to the surface of the particles, preventing MS2 from reaching the AWI and subsequently protecting MS2 from deactivation. Results from this study suggest that the BES we used might be less hydrophobic compared with MS2 bacteriophage; the power law factor is significantly less than 3. For AS, the virus size distribution is more likely to follow . The complex structure of the mucins, involving hydrophilic/hydrophobic domains, hydrogen bonds, and electrostatic interactions and their propensity to aggregate and form complexes with other polymers, can encapsulate MS2 inside particles and subsequently protect infectious MS2. Thus, rather than being homogeneously distributed inside the particles, MS2 might aggregate together within some domains of the particles depending on the association strength.
As the number of infectious viruses carried per particle is related to the aerosol generation and the subsequent evaporation processes, the theoretical infectious virus concentration for each particle size assuming that dry components of the aerosolized droplets is a constant fraction for all particle sizes (Walls et al. Citation2016) was estimated. The basic principle of this calculation is the number of infectious viruses carried per particle is equal to the number of infectious viruses in the droplet before evaporation, which could be determined by multiplying the virus concentration in the Collison Nebulizer with the volume of the aerosolized droplet (Walls et al. Citation2016). The count median diameter of the droplets was 3 µm as measured by APS, which agrees with another study from our lab (Chien et al. Citation2018). Assuming there was no deactivation of MS2 during the nebulization process, the theoretical vs. measured MS2 concentrations per particle are shown in . For all types of suspension media, the measured PFU/particle had the same trend as the theoretical PFU/particle, increasing with an increase of the theoretical PFU/particle, but for all cases the measured PFU/particle was less than the theoretical data, possibly due to deactivation of viruses during aerosolization or transportation processes. This is different from Zuo et al. (Citation2013), which shows that measured PFU/particle could be higher than the theoretical PFU/particle. One possible reason is the assumed count median diameter they used (about 500 nm) was too small compared with our measured results. For the BES and AS as the suspension media, it can be seen from that particles carried less infectious viruses per particle than those aerosolized with DI water. One possible reason is the infectious viruses shifted to larger particle size as a result of aggregation with other components in the BES and AS. Another possible reason for the BES as the suspension liquid is its hydrophobicity (discussed earlier), making MS2 more likely to stay in the liquid suspension in the Collison nebulizer rather than to be captured into droplets.
Conclusion
In summary, a sampler based on water condensation particle growth was used to obtain high efficiency of physical and viable collection of MS2 particles for investigating aerosolized infectious and total virus size distributions. The results illustrate that the number of infectious and total viruses per particle as a function of particle size varied with the nebulization medium. A conceptual model was created to explain the different distribution patterns of viruses inside the particles—viruses could aggregate together inside the virus-containing particles when aerosolized from the AS, homogeneously distributed within the particles when aerosolized from DI water, or tend to accumulate on the surface of the particles when aerosolized from the BES. A possible explanation is the different hydrophilic/hydrophobic properties of the spraying media that result in different power scaling size distributions. Furthermore, compared with the DI water and BES, AS could better protect infectious viruses from deactivation by preventing viruses reaching the AWI, possibly due to the complex structure of the mucin component. Future studies should test different types of viruses, especially hydrophilic virus, generated from different types of suspension media. Whether hydrophobic suspension media will protect aerosolized hydrophilic virus better, or whether hydrophilic suspension media will deactivate hydrophobic viruses, is an important knowledge gap; the present study has shed some light into this subject though more research is needed. Besides, investigation of the virus distribution in aerosol particles could be extended to above 500 nm to micrometer range. Results of this analytical model could put insight into the transmission model of infectious disease generated from different sources, i.e., human cough, sneezing, or infected surgery masks, so as to design an effective strategy to prevent the transmission of infectious virus aerosol.
Supplemental data for this article is available online at on the publisher's website.
Download Zip (218.9 KB)Additional information
Funding
References
- Adams, M. H. 1948. Surface inactivation of bacterial viruses and of proteins. J. Gen. Physiol. 31 (5):417–431.
- Appert, J., P. C. Raynor, M. Abin, Y. Chander, H. Guarino, S. M. Goyal, Z. Zuo, S. Ge, and T. H. Kuehn. 2012. Influence of suspending liquid, impactor type, and substrate on size-selective sampling of MS2 and adenovirus aerosols. Aerosol Sci. Technol. 46 (3):249–257. doi: 10.1080/02786826.2011.619224.
- Aps, J. K. M., and L. C. Martens. 2005. The physiology of saliva and transfer of drugs into saliva. Forensic Sci. Int. 150 (2–3):119–131. doi: 10.1016/j.forsciint.2004.10.026.
- Bansil, R., and B. S. Turner. 2006. Mucin structure, aggregation, physiological functions and biomedical applications. Curr. Opin. Colloid Interface Sci. 11 (2–3):164–170. doi: 10.1016/j.cocis.2005.11.001.
- Berg, G., R. S. Safferman, D. R. Dahling, D. Berman, and C. J. Hurst. 1984. USEPA manual methods for virology. EPA 600/4-84-013, US Environmental Protection Agency, Cincinnati, OH.
- Chien, C. H., C. Zhou, H. C. Wei, S. Y. Sing, A. Theodore, C. Y. Wu, Y. M. Hsu, and B. Birky. 2018. Feasibility test of cellulose filter for collection of sulfuric acid mists. Sep. Purif. Technol. 195:398–403. doi: 10.1016/j.seppur.2017.12.028.
- Diaz-Arnold, A. M., and C. A. Marek. 2002. The impact of saliva on patient care: A literature review. J. Prosthet. Dent. 88 (3):337–343. doi: 10.1067/mpr.2002.128176.
- Fabian, P., J. J. McDevitt, E. A. Houseman, and D. K. Milton. 2009. Airborne influenza virus detection with four aerosol samplers using molecular and infectivity assays: Considerations for a new infectious virus aerosol sampler. Indoor Air 19 (5):433–441. doi: 10.1111/j.1600-0668.2009.00609.x.
- Golmohammadi, R., K. Valegard, K. Fridborg, and L. Liljas. 1993. The refined structure of bacteriophage MS2 at 2·8 Å resolution. J. Mol. Biol. 234 (3):620–639. doi: 10.1006/jmbi.1993.1616.
- Hering, S. V., and M. R. Stolzenburg. 2005. A method for particle size amplification by water condensation in a laminar, thermally diffusive flow. Aerosol Sci. Technol. 39 (5):428–436. doi: 10.1080/027868290953416.
- Hering, S. V., M. R. Stolzenburg, F. R. Quant, D. R. Oberreit, and P. B. Keady. 2005. A laminar-flow, water-based condensation particle counter (WCPC). Aerosol Sci. Technol. 39 (7):659–672. doi: 10.1080/02786820500182123.
- Hinds, W. C. 1998. Aerosol technology: Properties, behavior, and measurement of airborne particles. 2nd ed. Hoboken, NJ: John Wiley & Sons.
- Hogan, C. J., E. M. Kettleson, M. H. Lee, B. Ramaswami, L. T. Angenent, and P. Biswas. 2005. Sampling methodologies and dosage assessment techniques for submicrometre and ultrafine virus aerosol particles. J. Appl. Microbiol. 99 (6):1422–1434. doi: 10.1111/j.1365-2672.2005.02720.x.
- Kutter, J. S., M. I. Spronken, P. L. Fraaij, R. A. Fouchier, and S. Herfst. 2018. Transmission routes of respiratory viruses among humans. Curr. Opin. Virol. 28:142–151. doi: 10.1016/j.coviro.2018.01.001.
- Leclair, J. M., J. A. Zaia, M. J. Levin, R. G. Congdon, and D. A. Goldmann. 1980. Airborne transmission of chickenpox in a hospital. New Engl. J. Med. 302 (8):450–453. doi: 10.1056/NEJM198002213020807.
- Lednicky, J., M. Pan, J. Loeb, H. Hsieh, A. Eiguren-Fernandez, S. Hering, Z. H. Fan, and C.-Y. Wu. 2016. Highly efficient collection of infectious pandemic influenza H1N1 virus (2009) through laminar-flow water based condensation. Aerosol Sci. Technol. 120 (3):805–815. doi: 10.1080/02786826.2016.1179254.
- Pan, M., T. Bonny, J. Loeb, X. Jiang, J. Lednicky, A. Eiguren-Fernandez, S. Hering, Z. H. Fan, and C. Y. Wu. 2017. Collection of viable aerosolized influenza and other respiratory viruses in a student health care center through water-based condensation growth. mSphere 2 (5):e00251–e00317. doi: 10.1128/mSphere.00251-17.
- Pan, M., A. Eiguren-Fernandez, H. Hsieh, N. Afshar-Mohajer, S. V. Hering, J. Lednicky, Z. H. Fan, and C. Y. Wu. 2016. Efficient collection of viable virus aerosol through laminar-flow, water-based condensational particle growth. J. Appl. Microbiol. 120 (3):805–815. doi: 10.1111/jam.13051.
- Rehman, Z. U., and W. H. Shah. 2005. Thermal heat processing effects on antinutrients, protein and starch digestibility of food legumes. Food Chem. 91 (2):327–331. doi: 10.1016/j.foodchem.2004.06.019.
- Seto, W. H. 2015. Airborne transmission and precautions: Facts and myths. J. Hosp. Infect. 89 (4):225–228. doi: 10.1016/j.jhin.2014.11.005.
- Tellier, R. 2009. Aerosol transmission of influenza a virus: A review of new studies. J. R. Soc. Interface 6:S783–S790. doi: 10.1098/rsif.2009.0302.focus.
- Thompson, S. S., and M. V. Yates. 1999. Bacteriophage inactivation at the air-water-solid interface in dynamic batch systems. Appl. Environ. Microbiol. 65 (3):1186–1190.
- Trouwborst, T., and J. C. De Jong. 1972. Mechanism of the inactivation of the bacteriophage T1 in aerosols. Appl. Microbiol. 23 (5):938–941.
- Trouwborst, T., and J. C. De Jong. 1973. Interaction of some factors in the mechanism of inactivation of bacteriophage MS2 in aerosols. Appl. Microbiol. 26 (3):252–257.
- Trouwborst, T., S. Kuyper, J. C. de Jong, and A. D. Plantinga. 1974. Inactivation of some bacterial and animal viruses by exposure to liquid-air interfaces. J. Gen. Virol. 24 (1):155–165. doi: 10.1099/0022-1317-24-1-155.
- Veerman, E. C. I., P. A. M. Keybus, A. Vissink, and A. V. N. Amerongen. 1996. Human glandular salivas: Their separate collection and analysis. Eur. J. Oral Sci. 104 (4):346–352. doi: 10.1111/j.1600-0722.1996.tb00090.x.
- Walls, H. J., D. S. Ensor, L. A. Harvey, J. H. Kim, R. T. Chartier, S. V. Hering, S. R. Spielman, and G. S. Lewis. 2016. Generation and sampling of nanoscale infectious viral aerosols. Aerosol Sci. Technol. 50 (8):802–811. doi: 10.1080/02786826.2016.1191617.
- Woo, M. H. 2012. Improving protection against viral aerosols through development of novel decontamination methods and characterization of viral aerosol. PhD diss., University of Florida, Gainesville, FL.
- Woo, M. H., A. Grippin, D. Anwar, T. Smith, C. Y. Wu, and J. D. Wander. 2012. Effects of relative humidity and spraying medium on UV decontamination of filters loaded with viral aerosols. Appl. Environ. Microbiol. 78 (16):5781–5787. doi: 10.1128/AEM.00465-12.
- Woo, M.-H., Y.-M. Hsu, C.-Y. Wu, B. Heimbuch, and J. Wander. 2010. Method for contamination of filtering facepiece respirators by deposition of MS2 viral aerosols. J. Aerosol Sci. 41 (10):944–952. doi: 10.1016/j.jaerosci.2010.07.003.
- Zuo, Z., T. H. Kuehn, A. Z. Bekele, S. K. Mor, H. Verma, S. M. Goyal, P. C. Raynor, and D. Y. H. Pui. 2014. Survival of airborne MS2 bacteriophage generated from human saliva, artificial saliva, and cell culture medium. Appl. Environ. Microbiol. 80 (9):2796–2803. doi: 10.1128/AEM.00056-14.
- Zuo, Z., T. H. Kuehn, H. Verma, S. Kumar, S. M. Goyal, J. Appert, P. C. Raynor, S. Ge, and D. Y. H. Pui. 2013. Association of airborne virus infectivity and survivability with its carrier particle size. Aerosol Sci. Technol. 47 (4):373–382. doi: 10.1080/02786826.2012.754841.