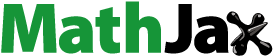
Abstract
A differential mobility classifier (DMC) is one of the core components in electrical mobility particle sizers for sizing sub-micrometer particles. A DMC in the cylindrical configuration (i.e., constructed by axial aligning of the inner and outer cylinders) is typically included in the sizers. The knowledge of construction tolerance is required in the design of a cylindrical DMC. The numerical approach was applied in this study. Our study shows that the DMC transfer function deteriorated as the axial eccentricity was increased (i.e., the peak is reduced and the width at the half peak height is broaden). At high axial eccentricity, the transfer function peak would split into two. In addition to the flow parameters such as the sheath-to-aerosol flow rate ratio and total flow rate, the effect of geometrical parameters (i.e., the length and aspect ratio of the particle classification channel, and the ratio of outer-to-inner cylinder radii) on the transfer function of an eccentric DMC were also investigated. It is found that the classification length and the sheath-to-aerosol flow rate ratio have obvious impact on the transfer function of an eccentric DMC. Furthermore, the particle diffusivity reduced the effect of axial eccentricity on DMC transfer function, especially for particles with the sizes less than 10 nm.
Copyright © 2019 American Association for Aerosol Research
EDITOR:
1. Introduction
A differential mobility classifier (or analyzer), DMC (or DMA), has been applied in aerosol particle studies for sizing and classifying particles in sub-micrometer and nanometer size ranges (by the electrical mobility of particles). It is one of the core components in electrical particle mobility sizers for measuring size distribution of particles. A DMC has also been applied by the National Institute of Standards and Technology (NIST) to certify the sizes of standard PSL particles in the diameters less than 100 nm (Kinney et al. Citation1991; Liu and Pui Citation1974).
DMCs in different configurations have been designed and their performance has been reported in the literature. The basic design of a DMC is either in the cylindrical, parallel-plate, or disk configuration (Ramechecandane et al. Citation2011; Steer et al. Citation2014; Zhang et al. Citation1995). Among them, cylindrical DMCs are the most popular because there is no sidewall presence in the particle classification channel. shows the schematic diagram of a cylindrical DMC. It is generally constructed by coaxially aligning one inner and one outer cylinder. Two inlets for polydisperse particle and clean sheath flows and two outlets for classified particle and excess flows, respectively, are designed in a DMC. Prior to entering a DMC, particles are required to be electrically charged to a well-known charge distribution. Once they enter, particles flow into the classification channel via the particle entrance slit. The clean sheath flow in the DMC serves as the barrier to prevent charged particles from reaching the classified particle exit slit as they follow the flow downstream. For the particle sizing or classification, an electrical field is established in the annular spacing between the inner and outer cylinders to drive charged particles crossing the sheath flow. Once crossed, charged particles in a certain range of electrical mobility will exit from the classified particle exit slit. Particles with electrical mobility greater or less than the certain range will be either deposited in the inner cylinder or carried out by excess flow. In principle, the flow direction in a DMC shall be different from that of an electrical field in order to achieve the particle separation. For the design simplicity, the flow direction is typically perpendicular to the electrical field direction.
Figure 1. The schematic diagram of a cylindrical DMC and computational domain in this modeling: (a) side view (with the illustration of the particle movement in the classification channel); (b) for the axial view of a concentric DMC; (c) for the axial view of an eccentric DMC.
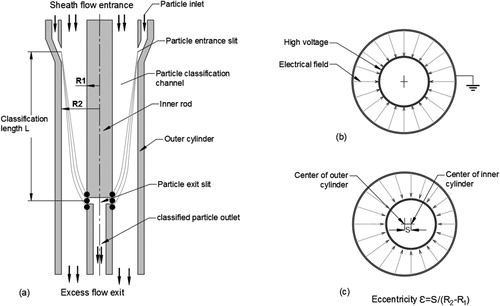
The development of modern cylindrical DMCs stems from the success of sorting particles in a narrow electrical mobility window and collecting the sorted ones by a filter created by Hewitt (Citation1957). Liu and Pui (Citation1974) improved the Hewitt’s design, enabling the extraction of classified particles from the DMC classification channel and counting the concentration of classified particles via an aerosol electrometer. They were the first to term the classifier as the ‘‘differential mobility analyzer’’ (Flagan Citation1998). Knutson and Whitby (Citation1975) further developed the fundamental theory to describe the performance of a cylindrical DMC, that is, transfer function (defined as the probability of a particle entering the classifier through the aerosol’s inlet and leaving with the classified aerosol flow), for non-diffusive particles. Their effort eventually led to the commercialization of the DMC originally designed by Liu and Pui (Citation1974).
The effect of particle diffusivity on the DMC transfer function was first investigated by Kousaka, Okuyama, and Adachi (Citation1985) and Kousaka et al. (Citation1986) through the comparison of theoretical and experimental data obtained from the tandem DMA (TDMA) setup. The theoretical transfer function of a cylindrical DMC for diffusive particles was later derived by Stolzenburg (Citation1988). Note that the summary of the derivation could be found in the paper of Stolzenburg and McMurry (Citation2008). Due to the requirement to study the particle nucleation events in the atmosphere, the late development of a scientific DMC is primarily on the sizing of particles in single digit nanometers (Fissan et al. Citation1996; Kozlowski and Ferna Citation2013; Tanaka and Takeuchi Citation2002; Zhang and Flagan Citation1996; Zhang et al. Citation1995).
The axial alignment of outer and inner cylinders in a cylindrical DMC is assumed perfect in all the DMC studies. In the real DMC construction, the axial eccentricity indeed exists when aligning the outer cylinder with the inner one (due to the presence of machining and construction tolerance). Unfortunately, our knowledge on the effect of axial eccentricity to the performance of a cylindrical DMC is very limited. The above knowledge, on the other hand, becomes essential in the design phase of a cylindrical DMC. To the authors’ knowledge, the effect of axial eccentricity on the performance of a cylindrical DMC was only investigated in the dissertation work of Knutson (Citation1972) with the assumptions of 2D flow and electrical fields in the particle classification channel and for non-diffusive particles. Note that the above work is in fact presented in the appendix, not in the main content of the dissertation. Uin, Tamm, and Mirme (Citation2011) applied the Knutson’s theory to investigated the transfer function of a very long DMA with the construction imperfection. Note that the above work is in fact presented in the appendix, not in the main content of the dissertation. The assumption of a 2D flow field, that is, either uniform or fully developed velocity profiles, in the particle classification channel of a cylindrical DMC is questionable. It is because the velocity profile of sheath flow is developing into a fully-developed profile as the flow initially enters the DMC classification channel in the uniform profile. The developing flow in the classification channel should be considered in 3D, not 2D, in an eccentric DMC. The numerical modeling will lift the assumption made in the Knutson’s derivation.
The objective of this work is thus to investigate the effect of axial eccentricity on the performance of cylindrical DMCs. A numerical approach was applied because of its cost-effectiveness compared with the experimental approach and its ability to lift the fully-developed velocity profile assumption made in the Knutson’s derivation. The COMSOL Multiphysics 5.3a® and MATLAB R2017a® were used to model the DMC performance. Prior to our investigation, the model was verified by the comparison of numerical results obtained for both concentric and eccentric DMCs with those calculated by the theory given in the work of Knutson (Citation1972) and in the experimental work of Chen et al. (Citation1998). The studied parameters include the geometrical variables, that is, the axial eccentricity, the ratio of outer to inner cylinder radii, the aspect ratio of the particle classification channel (i.e., the ratio of hydraulic diameter of the annular spacing and the length of the particle classification channel), and the cross-sectional area of the particle classification channel in addition to the flow parameters, that is, the sheath-to-aerosol flow rate ratio and total flow rate (i.e., the sum of sheath and aerosol flow rates). Furthermore, the effect of particle diffusivity on the eccentric DMC transfer function was also studied.
2. Numerical modeling of cylindrical DMCs
Both COMSOL Multiphysics 5.3a® and MATLAB R2017a® Multiphysics were used to set up the DMC modeling. A typical computational domain for modeling the performance of a cylindrical DMC is shown in . are the cross-sectional views of the computational domain in the longitudinal and radial directions, respectively, for a concentric DMC. shows a typical cross-sectional view of an eccentric DMC. Note that the eccentricity, ε, of the inner cylinder relative to the outer one is defined as the ratio of the axial displacement (relative to the outer cylinder axis) to the annular spacing of the classification channel.
2.1. For the flow and electrical fields
The steady and incompressible Navier-Stokes and continuity equations were applied to model the flow field in a DMC. The electrical field in the classification channel of a DMC was calculated by the Laplace equation for the electrical potential, and the electrical field intensity,
was calculated as
For the flow field calculation, the velocity at the sheath flow entrance was assumed either in the uniform or fully developed profile, while a uniform velocity profile was assumed at the particle inlet. A uniform velocity profile was assumed at the classified particle outlet. The pressure boundary condition was set at the exit of excess flow. The no-slip boundary condition was applied to all the solid walls in contact with fluid flow. For the electrical field, the inner cylinder was set at an elevated voltage, and the outer one was on the electrical ground.
2.2. Particle trajectory
Because of the small sizes of studied particles (i.e., negligible particle inertia effect), the motion of non-diffusive particles in a DMC was assumed to follow the flow and electrical fields (i.e., negligible particle inertial effect), the trajectory of a singly-charged particle in a DMC was thus computed via Equation (1):
(1a)
(1a)
(1b)
(1b)
(1c)
(1c)
where
are the initial particle location;
are the location of the particle after Δt time; u, v, w: are the three velocity component in the x, y, z coordinates respectively where y is the axial direction of particle traveling distance; Zp is particle mobility and
are the electrical field in x, y, and z coordinates. The initial velocity of particle released at the particle inlet was assumed the same as the flow velocity at the same location.
The modeling of diffusive particle motion was done by the Monte Carlo method (Hagwood, Sivathanu, and Mulholland Citation1999). The additional random displacement terms, where i = x, y, and z, are included in EquationEquation (1)
(1a)
(1a) . The random displacement was generated form the normal data distribution and its standard deviation of
is given as:
(2)
(2)
where i is the Cartesian coordinates (x, y, z); D is the particle diffusivity and
is the time step; and g is the random generator function.
2.3. For the DMA transfer function
The performance of a DMC is characterized by its transfer function, defined as the probability of a particle with a given electrical mobility entering the DMC classification region and reaching the classified aerosol exit. The calculation of the transfer function of a DMC for singly charged particles of a given size was based on the particle flux. In the computation, the annular spacing for the particle entrance were partitioned into fine meshes distributed based on the meshing scheme suggested in the works of Masset, Brüls, and Kerschen (Citation2011) and Beckers and Beckers (Citation2012). For non-diffusive particles, a representative particle was released from the centroid of each mesh at the particle entrance. The trajectories of representative particles were calculated and their fates (i.e., either deposited in or transmitted through the classification channel) were recorded. With the assumption of uniform particle concentration at the particle entrance, the transfer function of a DMC was calculated as
(3)
(3)
where
is the numerical transfer function of a DMC for singly charged particles of a given size;
is the total number of particles released from the aerosol entrance;
is the flow velocity at the released particle location;
is the area of the mesh associated with the released particle, i, and
is the probability of particles released from the mesh, i, and penetrating through the particle classification channel of a DMC. For non-diffusive particles, the value of
is 1.0 if a representative particle released from the centroid of the mesh, i, was penetrating through the classification channel and is 0 if the particle were either deposited or carried out by excess flow. For diffusive particles, at least 100 particles were released from the mesh, i, to access the probability of a particle exiting the classification channel through the classified particle slit.
Note that, for a concentric DMA, the theoretical transfer function for non-diffusive particles under the assumption of 2D flow and electrical fields in the particle classification channel, is given by Knutson and Whitby (Citation1975) and can be re-expressed by Stolzenburg (Citation1988) as,
(4)
(4)
where
in which
is the particle electrical mobility and
is the electrical mobility of particles at the maximum penetration at the same voltage;
is the flow ratios as
is the classified aerosol flow rate,
is the ploydisperse aerosols flow,
is the excess flow rate, and
is the clean sheath flow rate. The required voltage for the maximal penetration through the classification channel for particles of a given electrical mobility is given by Hewitt (Citation1957),
(5)
(5)
where
and
are the radii of the outer and inner cylinders, respectively; L is the length of particle classification channel; and
is the electrical mobility of singly charged particles of a given size. In the cases of eccentric DMCs, the eccentricity, ε, of axial alignment of inner and outer cylinders is defined as the ratio of the axial displacement of the inner rod and outer cylinder,
to the annular spacing,
between them.
(6)
(6)
The theoretical transfer function of an eccentric DMC for non-diffusive particles was derived by Knutson (Citation1972). For reference, the derivation is briefly summarized in the supplementary information (SI).
gives the values of the physical properties of gas and particles used in our modeling. The effect of axial eccentricity on the performance of a cylindrical DMC was investigated for particles in the diameters of 5, 10, 50, 160, 340, and 815 nm and under the various conditions of sheath-to-aerosol flow rate ratio, β, of 3.33, 4, 5, 6.67, 10, 20, 50 (at which the both sheath and aerosols flow rates were varied from 5 to 20 lpm and from 0.5 to 2 lpm, respectively). The diffusivity of particles was considered for the particle sizes less than 50 nm.
Table 1. The list of physical properties of gas and particles, sheath and aerosol flow rates used in the modeling of cylindrical differential mobility classifiers.
3. Validation of numerical modeling
show the comparison of the height and width at the half peak height (WHPH) of the numerical transfer function with the experimental data given in Chen et al. (Citation1998) for Nano-DMA when operated at the aerosol and sheath flow rates of 1.5 and 15 lpm. The height and WHPH data shown in the above figures were obtained from the de-convolution of TDMA curves, calculated first by the convolution of numerical transfer functions and then by the de-convolution of TDMA, assuming the functional form of the DMC transfer function is in the triangular shape. A reasonable agreement between the numerical and experimental data was obtained. The height of the DMC transfer function decreases as the particle size reduces and the WHPH of the DMC transfer function remains constant at 0.1 and increases as the particle size is less than 10 nm. The above observed trends are because of the Brownian diffusion of particles.
Figure 2. The comparison of numerical transfer functions with the experimental data (Chen et al. Citation1998) and diffusive transfer function (Stolzenburg Citation1988) for Nano-DMA: (a) the height and (b) width at half peak height (WHPH) of transfer function (assumed in triangular shape) as the function of particle size; (c) the diffusive transfer function of Stolzenburg (Citation1988) for 3 nm particle size. The Nano-DMA was operated at the aerosol and sheath flow rates of 1.5 and 15 lpm.
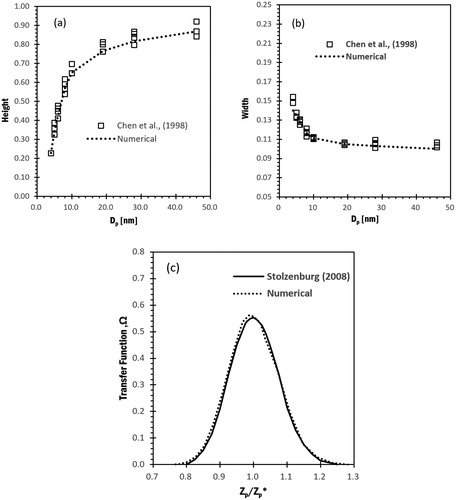
The comparison of the numerical transfer function of Nano-DMA with the theoretical one (Stolzenburg and McMurry Citation2008) for the particle size of 3 nm is also given in when it was operated at the aerosol and sheath flow rates of 1.5 and 15 lpm, respectively. A good agreement was achieved in the above comparison.
4. Result and discussion
4.1. Flow and electrical fields in an eccentric DMA
For illustration, the flow field (at the cross section of 20 cm from the sheath flow entrance) in the particle classification channel of an eccentric DMC having the inner and outer diameters of 20 and 40 mm, respectively, and with 40% eccentricity when operated at the sheath flow rate of 10 lpm is given in . is the velocity in the circumferential direction (whose magnitude was scaled up by a factor of 1,500) and is the contour of the velocity magnitude in the axial direction. The velocity profile of sheath flow at the entrance of the particle classification channel was assumed uniform. Because of the axial eccentricity, the annular spacing between the inner and outer cylinders of the DMC is not constant. As a result, the axial velocity is maximal at the location with the widest spacing and reduced as the spacing became smaller, eventually reaching the minimum at the location with the narrowest spacing. The circumferential velocity is minimal at the location with the narrowest spacing and maximal at the location with the widest spacing. For the same spacing reason, the electrical field intensity is the highest at the narrowest spacing and the lowest at the widest spacing (as shown in ). Note that the developing length for the flow in an eccentric DMC channel under different flow rates and DMC eccentricity was further obtained in this work. A brief summary of this part of work is given in the SI for reference.
Figure 3. The illustration of flow and electrical fields in the classification channel of a cylindrical DMC with an outer and inner cylinder radii of 40 and 20 mm, respectively, and at 40% eccentricity. The shown data is at the cross section located at 20 cm downstream the sheath flow entrance: (a) the circumferential component of sheath flow velocity (with the magnification of 1,500); (b) the contour of axial component of sheath flow velocity; (c) the contour of electrical potential (normalized by the applied voltage, V0).
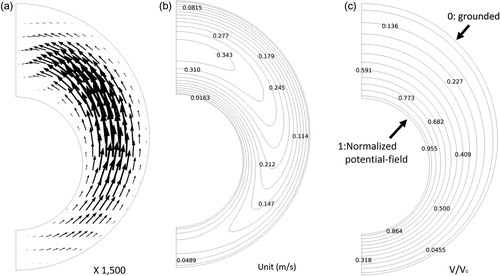
4.2. Transfer function of an eccentric DMC
shows the numerical transfer function of cylindrical DMCs with the axial eccentricity of 1%, 2%, and 5% for the particle size of 160 nm. These DMCs, operated at the aerosol and sheath flow rates of 1 and 10 lpm, respectively, had the particle classification length, inner and outer cylinder diameters of 5, 2, and 4 cm, respectively. Also included in the same figure is the theoretical transfer function calculated by the eccentric DMC theory given by Knutson (Citation1972). Both uniform and fully developed velocity profiles at the sheath flow entrance of each studied DMC were assumed. In the cases with uniform velocity profile assumption, the flow in the particle classification channel was 3D (i.e., with the presence of the circumferential flow component) because of the sheath flow velocity profile developing to a fully developed one. In the cases of assuming the fully developed profile at the sheath flow entrance, no circumferential velocity component was present in the classification channel of a DMC.
Figure 4. The comparison of the numerical transfer function of an eccentric DMC with the assumption of uniform and fully-developed velocity profiles at the sheath flow entrance with theoretical transfer function of Knutson (Citation1972) for particle size of 160 nm. The studied DMC has the particle classification length of 5 cm, and an inner and outer cylinder radii of 10 and 20 mm, respectively. The sheath and aerosol flow rates of the studied DMCs are 10 and 1 lpm, respectively. (a) For 1% eccentricity, (b) 2%, and (c) 5%.
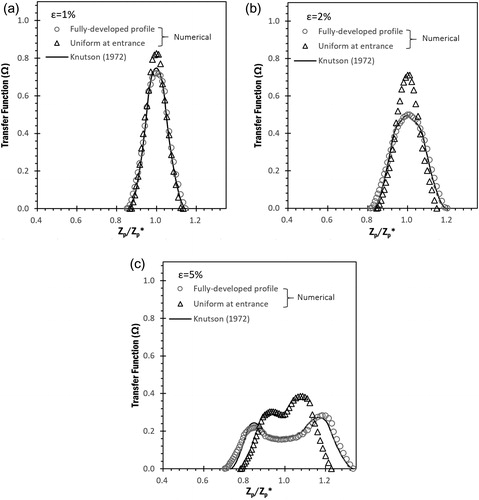
It is observed that, in general, the peak of DMC transfer function was reduced while the WHPH was broadened as the axial eccentricity of the DMC was increased. In the case of 5% eccentricity, the peak of transfer function was split into two (i.e., double-peaked) with one peak in the low electrical mobility range, lower than the other one in high mobility range. Note that the theoretical transfer functions with the assumption of fully developed velocity profiles are comparable to numerical ones, assuming the fully-developed profile at the sheath flow entrance, but not with the ones assuming the uniform profile.
To better understand the splitting of transfer function peak in the case of 5% eccentricity, the locations of 160 nm particles in the neighborhood of the classified particle exit slit after they were released from the particle inlet for the DMCs with eccentricities of 1% and 5%, and under the assumption of uniform and fully-developed flow profiles at the sheath flow entrance (plotted as the function of circumferential angle from 0 to 2π) is shown in and b for the DMC with 1% and 5% eccentricity, respectively). Note that the circumferential angles related to the location at the narrowest and widest spacing of the DMC particle classification channel are 0 and π, respectively. Because of the variation of particle traveling distance and time, the particle position when moving close to the classified particle exit slit was varied at different angles. Only particles moved in the spacing nearby the classified particle exit slit (shown as the lines in the figure) are expected to exit the classification channel. From the figure, the location variation of particles in the neighborhood of the exit slit was increased with the increase in DMC eccentricity, resulting in more particle loss in the classification channel. The variation status of particle position nearby the classified particle exit slit is also dependent on the electrical mobility of particles. The nearby-the-exit-slit location of particles with higher electrical mobility is less varied than that of ones with low electrical mobility. It is why the peak in low mobility range is lower than that in high mobility range once the peak of the transfer function is split.
Figure 5. The position of particles in the neighborhood of the classified particle exit slit after they were released from their initial location under the assumption of a uniform and fully developed velocity profile at the sheath flow entrance of studied DMC: the angle of 0 is associated with the widest spacing and π with the narrowest spacing of the annular classification channel. The annular classification channel of an eccentric DMC having the 5 cm classification length, and inner and outer radii of 10 and 20 mm (operated at the aerosol and sheath flow rates of 1 and 10 lpm, respectively): (a) 1% eccentricity and (b) 5%.
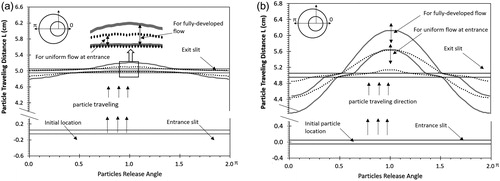
Moreover, the peak of transfer function is calculated by assuming the fully developed profile at the DMC sheath flow entrance is lower and its WHPH is wider compared to those in the cases with the uniform profile assumption. The reason for the above observation is illustrated in as the variation status of particle position at the exit slit in the case with the uniform flow assumption is less than that in the case with fully developed flow assumption.
4.3. Dominant factor for the shape of eccentric DMC transfer function
The distortion of flow and electrical field in the particle classification channel of an eccentric DMC is attributable to the shape change of the DMC transfer function. In this part of the work, we investigated which one is the dominant factor influencing the shape of the DMC transfer function. shows the numerical transfer functions calculated with the assumption of uniform and fully developed velocity profiles at the sheath flow entrance for a cylindrical DMC with 5% eccentricity, operated at the aerosol and sheath flow rates of 1 and 10 lpm, respectively. Also included in the figure is the calculated transfer function with the assumption of uniform velocity profile in the entire particle classification channel. The particle classification length of studied DMCs was varied from 5 to 45 cm. The studied particle size was 160 nm.
Figure 6. The numerical transfer function of a DMC with 5% eccentricity and operated at the aerosol and sheath flow rates of 1 and 10 lpm, respectively. The inner and outer cylinder radii of studied DMCs were 10 and 20 mm, respectively. The studied particle size is 160 nm. (a) The cases with the classification channel lengths of 5, 15, and 45 cm under the uniform velocity profile at the sheath flow entrance, and under the fully developed profile; (b) is for the cases under the assumption of both fully-develop and uniform velocity profiles at the sheath flow entrance, and under the assumption of uniform sheath flow in the entire channel. The DMC channel length was fixed at 45 cm; (c) is for the cases with both flow and electrical field distortion, and with the distortion of electrical field only. The DMC classification length was fixed at 5 cm. For the reference, the full width at half maximum (FWHM) of shown transfer functions is also given.
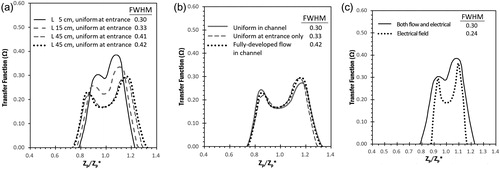
shows the shape variation in the transfer function of a DMC with the inner and outer cylinder radii of 10 and 20 mm (operated at the aerosol and sheath flow rates of 1 and 10 lpm) as the classification length of the eccentric DMC was increased. Also included in the figure is the transfer function calculated with the fully developed flow profile assumption. It is found that the transfer function of an eccentric DMC approached the one calculated with the assumption of fully developed velocity profile as the DMC classification length increased. It is because, under the same sheath flow rate, the percentage of the developing flow length relative to the entire classification channel length was reduced as the DMC particle classification length was increased. gives the transfer functions of an eccentric DMC under different flow fields in the particle classification channel (i.e., uniform profile at the sheath flow entrance, uniform and fully developed flow profile in the entire classification channel). The particle classification length of the DMC was 45 cm. It is found that the effect of flow field variation on the transfer function of the eccentric DMC is in fact secondary.
shows the comparison of calculated transfer function of a DMC with the classification length of 5 cm and 5% eccentricity under the assumptions of uniform velocity profile in the entire particle classification and only at the sheath flow entrance. The resulting transfer function calculated by assuming uniform flow profile in the classification channel is attributed to the effect of electric field distortion. The difference between the two transfer functions is attributed to the effect of flow field distortion. It is thus found that the shape and peak split of an eccentric DMC transfer function is primarily due to the distortion of the electric field. The distortion of the flow field makes the transfer function broader.
4.4. Effect of sheath-to-aerosol flow ratio and total flow rate on eccentric transfer function
The effect of sheath-to-aerosol flow rate ratio (β) on the transfer function of an eccentric DMC was investigated in this part of study. The studied DMC with the 2.5% eccentricity has the classification length of 45 cm, and the inner and outer cylinder diameters of 20 and 40 mm, respectively. shows the numerical transfer functions of the studied DMCs when operated at β = 4, 5, 10, and 20. It is noticed that the effect of eccentricity on the transfer function of an eccentric DMC is much more severe when operated at a high sheath-to-aerosol flow rate ratio (i.e., at high sizing resolution power). Also notice that it is confirmed by our modeling that the eccentric DMC transfer function for non-diffusive particles remained the same when the sheath-to-aerosol flow rate ratio was kept constant.
Figure 7. Comparison of the numerical transfer function of a DMC with an inner and outer cylinder radii of 10 and 20 mm, respectively and with 2.5% eccentricity when operated at sheath-to-aerosol flow rate ratios β of 4, 5, 10, 20 (for sheath flow of 10 lpm and areoles flow of 2.5, 2, 1, and 0.5, respectively). The classification length of studied DMCs was 45 cm. For the reference, the full width at half maximum (FWHM) of shown transfer functions is also given.
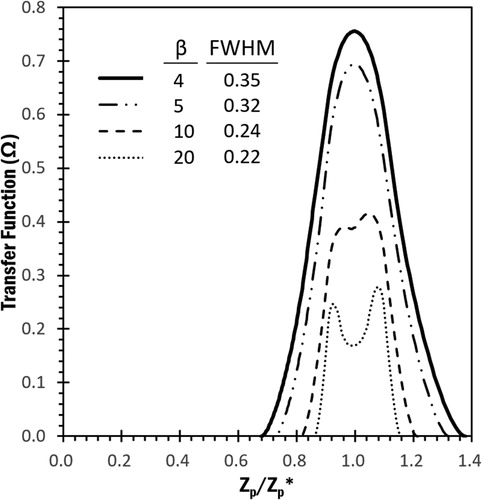
shows the numerical transfer function of a cylindrical DMC with the 5% axial eccentricity and the particle classification length of 5 cm (operated at the sheath-to-aerosol flow rate ratio of 10 under different total flow rates). It is found that the reduction of total flow rate while keeping the sheath-to-aerosol flow rate ratio constant increased in the particle loss, resulting in the reduction of transfer function. It is because of flow velocity reduction at the classified particle exit slit (to overcome the electric force for particle exiting).
Figure 8. Comparison of the calculated transfer functions of a DMC having R1, R2, and L of 10, 20, and 50 mm, respectively when operated at the total flow rates of 2.75, 5.5, 11, and 22 while keeping the value of β at 10. The studied DMCs have the eccentricity of 5%. For the reference, the full width at half maximum (FWHM) of shown transfer functions is also given.
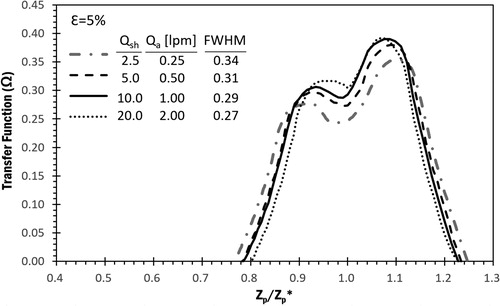
4.5. Effect of DMC geometry on eccentric transfer function
Three geometrical parameters of a cylindrical DMC (i.e., L,
where L is the particle classification length, and R1 and R2 are the radii of inner and outer cylinders) were considered in this part of the investigation.
illustrates the effect of the particle classification channel, L, on the transfer function of a DMC having 5% eccentricity and operated at the aerosol and sheath flow rates of 1 and 10 lpm, respectively, while keeping both the radial ratio and the channel aspect ratio
of 2 and 5, respectively. It is found that the value of the transfer function was decreased as the length of the particle classification channel was increased. It is because of the increased effect of circumferential velocity component in a long DMC channel, causing more particle loss to the walls of inner cylinder. gives the numerical transfer functions for the DMCs with 5% eccentricity and a particle classification length of 5 cm while having radial ratios of 1.2, 2, and 4 (for the radii ratios R2:R1 of 6:5, 2:1, and 1.33:0.33 cm, respectively). The result shows the increase of the radii ratio (which is associated with the decrease of the cross-sectional area of the annular channel) decreased the height of the eccentric DMC transfer function. It is because, at the same flow rate and classification channel length, the small cross-sectional area resulted in high circumferential velocity during the flow developing, leading to more particle loss to the inner cylinder walls. presents the calculated transfer function of cylindrical DMCs with the 5% eccentricity, 5 cm particle classification length and radial ratio of 2 while varying the channel aspect ratios of 1, 5, and 10 (for the R2:R1 ratio of 10:5, 2:1, 1:0.5, respectively). It is found that the effect of the channel aspect ratio on an eccentric transfer function is minor. The DMC with a high channel aspect ratio has slightly lower transfer function compared to those of DMC with lower channel aspect ratios (due to the same reason described above).
Figure 9. The effect of DMC geometrical parameters on the transfer function of an eccentric DMC; (a) varying particle classification length (L = 2.5, 5, 10, and 15 cm) of a DMC while keeping the radii ratio and channel aspect ratio constant (2 and 5); (b) varying the radii ratio (R2/R1 = 1.2, 2, and 4) while keeping the channel length and aspect ratio constant (5 cm and 5); (c) varying the channel aspect ratio, L/(R2 − R1) = 1, 5, and 10) while keeping the channel length and the radii ratio constant (5 cm and 2). For the reference, the full width at half maximum (FWHM) of shown transfer functions is also given.
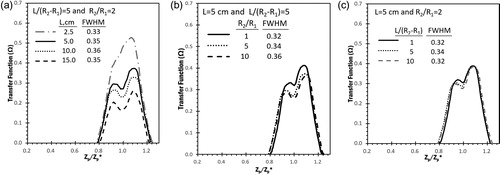
4.6. Effect of particle diffusivity on eccentric DMC transfer function
shows the transfer functions for DMCs with the eccentricity of 0, 1%, 2%, and 5% for particles in the sizes of 5, 10, and 50 nm. The DMCs with the particle classification length of 5 cm were operated at the aerosol and sheath flow rates of 1 and 10 lpm. In all the studied cases, the increase of particle diffusivity reduced the peak and increased WHPH of the DMC transfer functions. It is found that the combinational effects of axial eccentricity and particle diffusivity make the peak and WHPH of the DMC transfer function lower and broader, compared with those observed due to individual effects. In addition, the particle diffusivity smeared the double-peaked transfer functions, making the peak splitting occur at the higher eccentricity. As shown in the figure, the double-peaked transfer function was not observed for the particle size less than 10 nm (which was observed for all the particle sizes without the consideration of particle diffusivity, given in ) for the same DMC operated under the same flow rate conditions.
Figure 10. Calculated transfer function of DMCs having the 0%, 1%, 2%, and 5% eccentricity for diffusive particles: (a) 50 nm particles; (b) 10 nm particles; (c) 5 nm particles. The studied DMC have inner and outer radii of 10 and 20 mm, and classification length of 5 cm were operated at the aerosol and sheath flow rates of 1 and 10 lpm, respectively. For the reference, the full width at half maximum (FWHM) of shown transfer functions is also given.
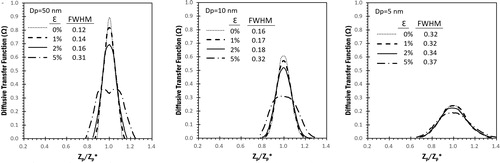
4.7. Classification of eccentric DMC transfer function
For a given DMC operated at the fixed rates of aerosol and sheath flows, the shape of the transfer function is varied as the axial eccentricity is increased. An example of such variation of DMC transfer function is shown in for the DMC having the R1, R2, and L of 1, 2, and 45 cm, and operated at the aerosol and sheath flow rates of 1 and 10 lpm, respectively. The shape of the transfer function for the concentric DMC is in the triangular (once the electrical mobility, Zp was normalized by the central mobility, Zp*). With the increase of axial eccentricity, the peak of DMC transfer function is decreased, flattened and eventually double-peaked. Such shape variation is also dependent on the sheath-to-aerosol flow rate ratio, β. Accordingly, the evolution of transfer function shape for a cylindrical DMC due to the axial eccentricity could be classified into three regions: single-peaked, transient, and double-peaked regions (as shown in ). The determination of transfer function in three regions was based on the slopes at the peak of transfer function, which are described in the SI for the reference. From the above figure, it is shown that a DMC is required to have very tight tolerance on the axial eccentricity to well perform the particle sizing measurement in high sizing resolution, especially for the one with long particle classification channel.
Figure 11. (a) The classification of non-diffusive transfer function of eccentric DMCs with inner and outer radii of 10 and 20 mm, and a classification length of 45 cm. The aerosol and sheath flow rates of the DMCs were 1 and 10 lpm, respectively. (b) Three classified zones for eccentric DMC transfer function as the functions of the eccentricity ε and the flow ratio β for DMAs with the classification lengths of 5 and 45 cm. For the reference, the full width at half maximum (FWHM) of shown transfer functions is also given.
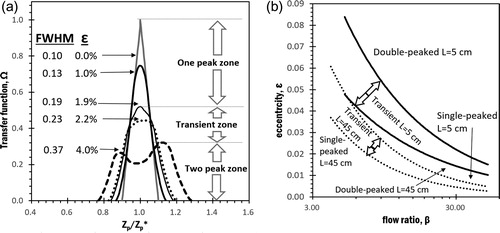
5. Conclusion
The effect of axial eccentricity on the transfer function of a cylindrical DMC has been numerically investigated. COMSOL 5.3a® Multiphysics and MATLAB R2017a® were applied to set up the numerical model. Our modeling was validated by the comparison of the calculated transfer functions with the experimental and theoretical ones for concentric and eccentric DMCs reported in the literature.
The axial misalignment of the inner rod and outer cylinder in a cylindrical DMC distorts the flow and electrical fields in the annular particle classification channel of the DMC, resulting in the change of its transfer function. The eccentricity effect on the DMC transfer function was characterized by the decrease of peak and increase of width at half the peak height in the DMC transfer function at the low axial eccentricity. As the eccentricity increased, the peak of the DMC transfer function was flatted and eventually split into two peaks (i.e., double-peaked transfer function with one in low mobility range lower than the other in high mobility range). Further study shows that the shape distortion of transfer function of a cylindrical DMC was dominantly attributed to the distortion of electrical field in the annular particle classification channel. The distortion of flow field in an eccentric DMC made the width of transfer function broader.
The sheath-to-aerosol flow rate ratio (β) had significant effect on the transfer function of an eccentric DMC. As the value of β increased, the shape change of the eccentric transfer function was increasingly sensitive to the axial eccentricity. In addition, the eccentric DMC transfer function will not be the same when varying the total flow rate while keeping the sheath-to-aerosol flow rate ratio constant. It is because of the difference in the circumferential velocity component during the flow profile development from the uniform one at the sheath flow entrance to the fully developed one downstream the classification channel.
The effect of the DMC classification channel length on the eccentric DMC transfer function is more obvious on a long DMC compared with that on a short DMC. It is due to the fact that the flow profile in the annular classification channel of a short DMC remains mostly uniform (i.e., the effect of the circumferential velocity component on the particle motion is minor) in the short DMC. The radius ratio of a cylindrical DMC (i.e., R2/R1) has minor effect on the performance of an eccentric DMC in the cases of assuming fully developed velocity profile at the sheath flow entrance. Its effect on an eccentric DMC transfer function is however observable in the cases of assuming uniform velocity profile at the sheath flow entrance. The above observation is even more obvious in a long DMC. The effect of aspect ratio of particle classification channel on an eccentric transfer function is however negligible.
The peak height and sizing resolution of the eccentric DMC transfer function was reduced with the consideration of particle diffusivity. The particle diffusion made the effect of axial eccentricity less obvious on eccentric DMC transfer function. The double-peaked transfer function of an eccentric DMC occurred at higher eccentricity when compared to that without considering the particle diffusivity.
Supplemental Material
Download Zip (235.1 KB)Additional information
Funding
References
- Beckers, B., and P. Beckers. 2012. A general rule for disk and hemisphere partition into equal-area cells. Comp. Geom.-Theor. Appl. 45 (7):275–83. doi:10.1016/j.comgeo.2012.01.011.
- Chen, D. R., D. Y. H. Pui, D. Hummes, H. Fissan, F. R. Quant, and G. J. Sem. 1998. Design and evaluation of a nanometer aerosol differential mobility analyzer (Nano-DMA). J. Aerosol Sci. 29 (5-6):497–509. doi:10.1016/S0021-8502(97)10018-0.
- Fissan, H., D. Hummes, F. Stratmann, P. Büscher, S. Neumann, D. Y. H. Pui, and D. Chen. 1996. Experimental comparison of four differential mobility analyzers for nanometer aerosol measurements. Aerosol Sci. Technol. 24 (1):1–13. doi:10.1080/02786829608965347.
- Flagan, R. C. 1998. History of electrical aerosol measurements. Aerosol Sci. Technol. 24 (4):301–80. doi:10.1080/02786829808965530.
- Hagwood, C., Y. Sivathanu, and G. Mulholland. 1999. The DMA transfer function with brownian motion a trajectory/Monte-Carlo approach. Aerosol Sci. Technol. 30 (1):40–61. doi:10.1080/027868299304877.
- Hewitt, G. W. 1957. The charging of small particles for electrostatic precipitation. AIEE, Part I: Commun. Electron. Trans. 76(3):300–6.
- Kinney, P. D., D. Y. H. Pui, G. W. Mulholland, and N. P. Bryner. 1991. Use of the electrostatic classification method to size 0.1 μm SRM Particles – A feasibility study. J. Res. Natl. Inst. Stand. Technol. 96(2):147–76.
- Knutson, E. O. 1972. The distribution of electric charge among the particles of an artificially charged aerosol. Mechanical Engineering, PhD thesis, University of Minnesota.
- Knutson, E. O., and K. T. Whitby. 1975. Aerosol classification by electric mobility: Apparatus, theory, and applications. J. Aerosol Sci. 6:443–51. [Database] doi:10.1016/0021-8502(75)90060-9.
- Kousaka, Y., K. Okuyama, and M. Adachi. 1985. Determination of particle size distribution of ultra-fine aerosols using a differential mobility analyzer. Aerosol Sci. Technol. 4 (2):209–25. doi:10.1080/02786828508959049.
- Kousaka, Y., K. Okuyama, M. Adachi, and T. Mimura. 1986. Effect of brownian diffusion on electrical classification of ultrafine aerosol particles in differential mobility analyzer. J. Chem. Eng. Japan/JCEJ 19 (5):401–7. [Database] doi:10.1252/jcej.19.401.
- Kozlowski, J., and J. Ferna. 2013. Hand-held differential mobility analyzers of high resolution for 1 – 30 nm particles: Design and fabrication considerations. J. Aerosol Sci. 57:45–53. doi:10.1016/j.jaerosci.2012.10.009.
- Liu, B. Y. H., and D. Y. H. Pui. 1974. A submicron aerosol standard and the primary, absolute calibration of the condensation nuclei counter. J. Colloid Interface Sci. 47 (1):155–71. doi:10.1016/0021-9797(74)90090-3.
- Masset, L., O. Brüls, and G. Kerschen. 2011. Partition of the circle in cells of equal area and shape, 6. http://hdl.handle.net/2268/91953.
- Ramechecandane, S., C. Beghein, F. Allard, and P. Bombardier. 2011. Modelling ultrafine/nano particle dispersion in two differential mobility analyzers (M-DMA and L-DMA). Build Environ. 46 (11):2255–66. doi:10.1016/j.buildenv.2011.05.005.
- Steer, B., B. Gorbunov, R. Muir, A. Ghimire, and J. Rowles. 2014. Portable planar DMA: Development and tests portable planar DMA: Development and tests. Aerosol Sci. Technol. 48(3):251–60. doi:10.1080/02786826.2013.868863.
- Stolzenburg, M. R. 1988. An ultrafine aerosol size distribution measuring system. ProQuest Dissertations and Theses, University of Minnesota.
- Stolzenburg, M. R., and P. H. McMurry. 2008. Equations governing single and tandem DMA configurations and a new lognormal approximation to the transfer function. Aerosol Sci. Technol. 42 (6):421–32. doi:10.1080/02786820802157823.
- Tanaka, H., and K. Takeuchi. 2002. C60 monomer as an inherently monodisperse standard nanoparticle in the 1 nm range. Jpn. J. Appl. Phys. 41 (Part 1, No. 2A):922–4. doi:10.1143/JJAP.41.922.
- Uin, J., E. Tamm, and A. Mirme. 2011. Very long DMA for the generation of the calibration aerosols in particle diameter range up to 10 μm by electrical separation. Aerosol Air Qual. Res. 11 (5):531–8. doi:10.4209/aaqr.2011.05.0068.
- Zhang, S.-H., and R. C. Flagan. 1996. Resolution of the radial differential mobility analyzer for ultrafine particles. J. Aerosol Sci. 27 (8):1179–200.
- Zhang, S., Y. Akutsu, L. M. Russell, R. C. Flagan, J. H. Seinfeld, Y. Akutsu, and J. H. Seinfeld. 1995. Radial differential mobility analyzer. Aerosol Sci. Technol. 23 (3):357–72. [Database] doi:10.1080/02786829508965320.