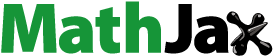
Abstract
An advanced rotating aerosol chamber was developed to study the changes of fluorescence spectral profile and intensity, the viability and the quantitative polymerase chain reaction (qPCR) signal of Escherichia coli aerosol particles as they are exposed to simulated atmospheric conditions over time. These conditions included relative humidity (RH) below 30% or ∼75%, ozone ∼100 ppb, volatile organic compounds (VOCs) α–pinene (∼5 ppb) or toluene (∼45 ppb), and simulated solar (SS) irradiation. Individual experiments examined the effects of these conditions applied individually and in combination. Experimental results demonstrate that the 263 nm laser excited UV fluorescence band (280–400 nm) showed the greatest rate of decrease, and the visible band (400–580 nm) generally had a smaller change rate which followed the change in the UV band. The 351 nm excited visible band (380–650 nm) had the smallest decay rates, and sometimes increased, when exposed to ozone, high RH, and VOCs. Generally, the viability, qPCR signal intensity, and the fluorescence intensity decayed faster when more variables were applied in combination. Simulated solar irradiation was the most dominant factor in the aging process, followed by the combination of high RH and ozone. Interestingly, the decay of fluorescence and qPCR signal do not appear to correlate directly with loss in viability. Therefore, additional studies are expected to further understand the mechanisms by which atmospheric chemical processes impact viability, qPCR signal intensity, and the fluorescence of biological aerosols.
EDITOR:
1. Introduction
Airborne aerosol particles, including biological particles, play an important role in the atmosphere. The physical, chemical, and biological properties of bioaerosols, and their interactions with the environment all have significant impacts on climate and human health (Ariya and Amyot Citation2004; Huang et al. Citation2014; Rosenfeld et al. Citation2014). There have been extensive studies on the properties of bioaerosol particles in collected samples, free moving particles, and trapped particles (Aptowicz et al. Citation2013; Hinds Citation1999; Krieger, Marcolli, and Reid Citation2012; Loh et al. Citation2012; Pan Citation2015; Redding, Schwab, and Pan Citation2015). However, the properties of aerosols in the atmosphere show significant temporal changes as they interact with the surrounding environment. The changes in bioaerosol properties in the atmosphere may result from oxidization processes, desiccative, or pH stresses from changes in aerosol water content due to relative humidity (RH) changes, degradation by solar radiation or photochemistry, interactions with radical species, and secondary aerosol formation processes (Cataldo Citation2006; Cliff et al. Citation2005; Cox, Hood, and Baxter Citation1973; Deguillaume et al. Citation2008; Dennis-Smither et al. Citation2014; Fan et al. Citation2002; Fujimori Citation1985; Ignatenko Citation1988; Kanaani et al. Citation2007; May, Druett, and Packman Citation1969; Pan et al. Citation2014; Ratnesar-Shumate et al. Citation2015; Santarpia et al. Citation2012; Selma et al. Citation2008). Early studies observed total fluorescence changes for fungal spores during exposure to flowing air in cultures of different ages (Kanaani et al. Citation2007), as well as the changes of viability, absorption, and fluorescence for bacteria and aromatic amino acids in bulk samples after exposure to ozone (Cataldo Citation2006; Cliff et al. Citation2005; Cox, Hood, and Baxter Citation1973; Deguillaume et al. Citation2008; Dennis-Smither et al. Citation2014; Fan et al. Citation2002; Fujimori Citation1985; Ignatenko Citation1988; Kanaani et al. Citation2007; May, Druett, and Packman Citation1969; Selma et al. Citation2008). In contrast, there are limited studies (Pan et al. Citation2014; Ratnesar-Shumate et al. Citation2015; Santarpia et al. Citation2012) on the effects of these “open air factors” (OAF) on aerosolized biological organisms suspended in air. Santarpia et al. (Citation2012) found that the UV fluorescence band (280–400 nm) excited by a 263 nm laser demonstrated large decreases for both Yersinia rohdei and MS2 bacteriophage aerosols in the presence of high concentrations of ozone (4300 ppb), especially at high relative humidity (RH). Pan et al. (Citation2014) studied the changes of fluorescence spectra from an eight-amino-acid-peptide aerosol within a atmospherically relevant ozone concentrations (0 or 150 ppb) under various RH conditions (∼20%, 50%, or 80%). The UV fluorescence band, excited by a 263 nm laser, of particles decreased significantly over time, while the visible fluorescence band excited by the 351 nm laser increased over time, especially in experiments where both ozone and RH were high. Ratnesar-Shumate et al. (Citation2015) further investigated the change of fluorescence spectra and biological activity of aerosolized Bacillus spores and MS2 bacteriophage, as they were exposed to ozone at different RH levels in a rotating drum. The changes observed in these studies appear primarily caused by the oxidation of tryptophan to N-formyl kynurenine (NF) and kynurenine (KY) (Fujimori Citation1985; Ignatenko Citation1988; Pan et al. Citation2014; Ratnesar-Shumate et al. Citation2015; Santarpia et al. Citation2012).
In the troposphere, particularly in areas impacted by anthropogenic emissions, trace chemical compositions are quite complex, and include the presence of many compounds that could affect the properties of bioaerosols. The changes of bioaerosols exposed to more complex mixtures of trace gases (e.g., O3, radical species, and volatile organic compounds [VOCs]), under different meteorological conditions (e.g., solar radiation, high RH, and temperature), require further study. Observations of these changes continue to be necessary to inform biosensor development, reduce false alarm rates, understand the limitations of bioaerosol detection and measurement, and better inform the use of existing instruments. Understanding the impact of OAF processes on bioaerosol measurement techniques remains an important subject of study. In this article, changes of fluorescence spectra, viability, and the sensitivity of qPCR detection for aerosolized Escherichia coli (E. coli) particles under laboratory-generated complex chemical aging processes in an improved rotating drum are reported.
2. Experimental arrangement
The experimental arrangement was similar to what has been described previously (Pan et al. Citation2014; Ratnesar-Shumate et al. Citation2015; Santarpia et al. Citation2012), but with an advanced rotating drum that was capable of introducing more chemical compounds. A general schematic of the experimental setup is displayed in . Briefly, the experimental apparatus was composed of a Goldberg rotating reaction drum chamber, an ultrasonic aerosol generator, an UV aerodynamic particle sizer (UV-APS), a single particle fluorescence spectrometer (SPFS), an all-glass impinger (AGI), and equipment to generate, monitor, and control the ozone, RH, simulated solar (SS) irradiation, and two model atmospheric VOCs (α-pinene or toluene). The VOCs were chosen to be representative of both biogenic (α-pinene) and anthropogenic (toluene) compounds that are commonly attributed to the formation of secondary organic aerosol in the atmosphere (Gatzche et al. Citation2017; Hildebrandt Ruiz et al. Citation2015). Concentration or intensity of ozone, water vapor, SS irradiation, and the two VOCs were controlled individually, so they can be introduced alone or together to examine their effects individually or in combination. The chamber had a volume of ∼400 L, corresponding to a radius of 15 in and a length of 36 in. The dimensions of the chamber ensure there was sufficient volume for intermittent sampling throughout the aging period of up to several hours. Dry, compressed laboratory air, filtered with a carbon and high-efficiency particulate arresting (HEPA) filter (Whatman Carbon Cap 150), was used to supply clean dry air to all vapor and aerosol generation systems. Each of the air flows was metered at a target flow rate with mass flow controllers (MFC, Alicat Scientific) and controlled through a LabVIEW interface. Teflon-coated stainless steel was used as the inert material of the drum to avoid the inadvertent generation of static charge during the rotation of the drum (Pan et al. Citation2014; Ratnesar-Shumate et al. Citation2015). All drum components involved in rotation were selected to ensure that no plastic on plastic contact was made during rotation. The Teflon coating also limited the reactivity and absorption of the chamber walls to improve observations inside the drum.
Figure 1. The schematic of the experimental setup. The scale of the axel relative parts of the rotating drum is exaggerated to show the details of the air and aerosol flow paths and different monitors.
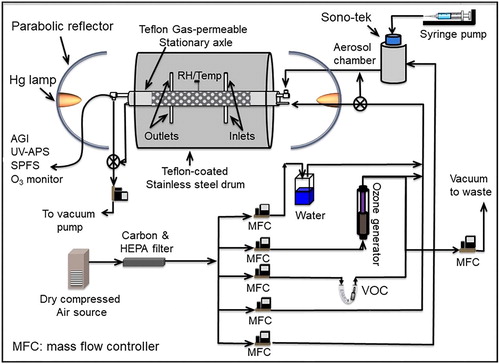
Chemically conditioned air (ozone, RH, and VOCs) was introduced into the chamber by two methods. Initially, conditioned air was input through the ports labeled inlets (), which allowed better mixing and directly replaced air in the drum with conditioned air. This method preconditioned the drum to the target chemical concentration and humidity quickly. Second, during an experiment, conditioned air flowed along a 5-cm-diameter perforated tube, lined with an expanded PTFE membrane, which acted as a gas permeable conditioning system. This approach allowed mixing of gaseous species through diffusion, while minimizing the impact to the aerosol concentration either by dilution or disruption to the rotational flow. Both the direct injection line and the sampling line had a conical end, with the increased cross-sectional area intended to reduce the disruption of rotating inertia by limiting injecting or sampling air velocity.
During the experimental process, the drum rotated at 1 RPM to reduce the settling rate for particles with a mode size about 2 μm in diameter. Change in particle size distribution, in a similar drum, rotating at 1 RPM is reported in Santarpia et al. (Citation2012) and Ratnesar-Shumate et al. (Citation2015). The average particle lifetime (1/e) in this study, under control conditions (low RH, no SS and no ozone, or VOCs), was 2.85 h (. Experimental conditions, such as high RH and SS light, can significantly change the physical loss rate, which requires normalization (described below).
Figure 2. Trials of the experimental variables applied into the rotational drum. (a) High relative humidity (RH∼75%) or low RH (below 30%) are maintained in the chamber for different trials. (b) Ozone was injected and kept around 100 ppb in two trials. Linear evaporation of toluene (c) and α-pinene (d). (e) The intensity distribution of the UV portion of the SS illumination across the rotation chamber. (f) The remaining percentage of 2-μm-diameter PSL particles remaining within the rotating drum.
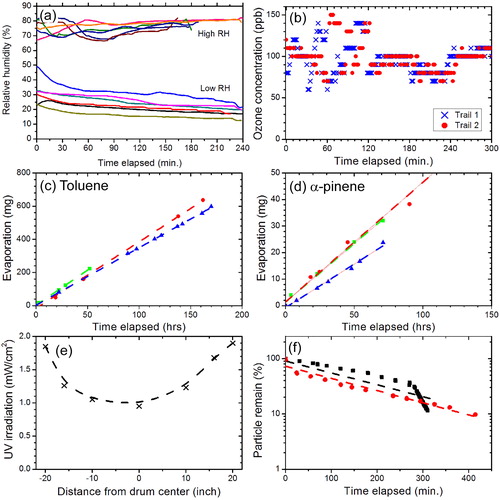
2.1. Relative humidity
To achieve a target RH, dry air was bubbled through a container with warm 18 MΩ DI water and then mixed with dry air. RH was monitored by a wireless temperature and humidity transmitter (ZW-003, HOBO Inc.) inside the drum. The ratio of humid to dry air was adjusted to produce the target RH using MFCs. Although there were initial humidity variations and additional evaporation from nebulized droplets, the target RH was maintained, with limited variations for both the high target (75–80%) or low target RH <30% (.
2.2 Ozone
Ozone was generated using a software-operated UV penlight (Pen Ray, 97-0067-01), enclosed within a custom PTFE housing, which was plumbed to allow MFC-controlled airflow over the UV lamp. The mercury lamp produces UV light with energy sufficient to photolyze oxygen (wavelengths <220 nm). The oxygen radical formed recombines with molecular oxygen (O2) to form ozone (O3). The ozone concentration was measured every 30 min, in combination with aerosol sampling. The long time between measurements caused fluctuation in concentration. Two randomly selected trials that show ozone concentration varying as a function of time (10 s intervals), when measured and controlled at 30-min cycles, are presented in . Similar to other measurement and injection strategies, the 30-min sampling time was intended to reduce disturbances to the rotational flow.
2.3. Volatile organic compounds
VOCs were introduced into the system using a diffusion vial (VICI Dynacal) to vaporize the target chemical compounds. Toluene and α-pinene were chosen as representative pollutant compounds, generated from anthropogenic and naturally occurring biogenic emissions processes, respectively. A VICI U-shaped glass permeation tube holder was used to encase the diffusion vial submerged in a 74 °C silicone oil bath. The tube holder was covered with Teflon-lined screw caps and contained glass beads to increase turbulence and heat transfer on the inlet side. The other side of the U-shaped glass tube contained a VICI Dynacal diffusion vial with a 2.0 mm diameter, 50.0 mm long capillary tube at the end of a reservoir containing 2.5 mL of the liquid to be vaporized.
Vaporized material from this type of diffusion vial released at a linear rate governed by the capillary diameter and length, as well as the temperature, molecular weight, pressure, and diffusion coefficient of the material as
(1)
(1)
where r = rate of diffusion (ng/min), T = temperature of vapor (°K), D0 = diffusion coefficient, M = molecular weight (g/mol), A = cross-sectional area of capillary (cm2), L = length of diffusion path (cm), P = atmospheric pressure (mm Hg), and ρ = vapor pressure of chemical at temperature T. As the VOC concentration in the supply line was constant, the VOC concentration in the drum was controlled by varying the VOC-laden air flow rate introduced into the drum and directing any residual to a waste line. A temperature of 74 °C ensured that both VOCs vaporized at high rates, minimizing any dilution effect on the humid air or ozone concentrations, while also lowering the volume and velocity of air entering the drum to prevent unnecessary particle losses. The vaporization rate of toluene was measured to be 4.01 mg/h (), of which only a fraction was introduced into the drum to achieve the target concentration of 45 ppb during experiments. This compares well with the predicted vaporization of toluene at 74 °C, 4.08 mg/h, using 74 °C for T, ρ of 0.316 bar (Goodwin Citation1989), Vici Vial C measurements (A = 0.0314 cm2, L = 5.08 cm, D0 of 0.0849 cm2/s), an ambient pressure of 610 mmHg and M of 92.13 g/mol. Similarly, the α-pinene vaporization rate was 0.38 mg/h (), diluted to achieve the target concentration of 5 ppb in the drum.
2.4. Simulated solar radiation
Simulated solar (SS) radiation was produced by two mercury halide lamps (1000 W, Virtual Sun) and directed into the drum with two parabolic reflectors aligned at both ends of the drum. Reflectors were covered with 0.25-in-thick expanded PTFE to produce a diffuse, but nearly perfect reflection of light at wavelengths down to ∼290 nm. The drum ends were covered by 0.125-in-thick acrylic windows (Acrylite, OP-4), which allows for more than 70% transmission of UV light at 280 nm, and ∼90% at 350 nm. Portable evaporative coolers were used at the bulb and window interface to limit the temperature fluctuation and keep the chamber between 23 and 28 °C. Measurements of UV irradiation along a diameter across the drum () showed reasonably uniform illumination across the chamber, with a minimum in the middle of the drum, furthest from each light source.
2.5. Generation of bioaerosols
Aerosols were generated using a 3 W, 120 kHz ultrasonic nozzle (Sono-Tek Inc.) from a liquid suspension in a 30-mL syringe driven by a 100 μl/min syringe pump (Sono-Tek Inc.). The nebulized droplets were introduced into the top of a stainless cylindrical aerosol drying chamber to mix with clean carrier air before entering the rotating drum. Clean air entered the chamber through a conical expansion, located near the top, at a rate of 10 L/min to limit shear stresses, particle velocities and impaction during drying. The drying chamber was 24 in in height and 6 in in diameter. Aerosols exited the chamber through a second conical reduction at the bottom of the aerosol chamber, and were introduced directly to the rotating drum. This aerosolization process has been described elsewhere for reproducibly achieving the target particle size, but may also affect the bioaerosol property when compared to natural bioaerosol formation (Pan et al. Citation2014; Ratnesar-Shumate et al. Citation2015).
2.6. Growth and preparation of E. coli material
E. coli K-12 strain was propagated from frozen stocks streaked for single colonies on lysogeny broth (LB) agar and incubated overnight at 37 °C. Single colonies were scaled to 10 mL overnight, then taking 0.5 mL of that overnight sample, scaled to 50 mL of LB, and incubated overnight at 37 °C in a baffled flask shaken at 250 RPM. Stock concentrations were determined by serially diluting and plating 1 mL replicates on 3 M Petrifilm Aerobic count plates. Three stocks of E. coli were prepared for these experiments. The titer varied from 8.33 × 109 to 1.16 × 1010 colony forming units per mL (CFU/mL). Each stock was kept in spent media for up to 28 days, for use in multiple experiments. To ensure that the viability or integrity of the E. coli cells was not changing significantly during the retention period, the initial impinger samples from each experiment were compared with others produced from the same E. coli stock. By comparing the concentration of collected E. coli following aerosolization, it was possible to determine if the cells were more sensitive to the aerosolization process after storage, or substantially affected by extended stationary phase storage. The data indicate that no significant change in the E. coli collected in the initial impinger sample over the time each stock was stored. The average result and standard deviation of the recovered E. coli concentration in AGI-30 impingers for each of the stocks was: 8.73 × 103±6.02 × 103 CFU/mL, 2.99 × 104±1.68 × 104 CFU/mL, and 3.86 × 104±1.43 ×104 CFU/mL. The variations in initial impinger recovered stocks resulted in standard deviations of 0.23, 0.26, and 0.18 logs over all experimental condition combinations, and storage time periods of 23, 21, and 15 days, respectively. Further, no trend was observed in these concentrations over the lifetime of each stock, indicating the degradation of the E. coli in storage, over the course of these experiments, unlikely impacted the results of these experiments. Working suspensions of E. coli K-12 for aerosol generation were created daily by diluting refrigerated stocks in sterile-filtered DI water (5% E. coli in spent medium and 95% DI water), to lower the contribution of dissolved solids from the spent media to the final dry particle size yielding an average working suspension concentration of 4.98 × 108 CFU/mL.
2.7. Fluorescence measurements
The SPFS (Pan et al. Citation2014; Ratnesar-Shumate et al. Citation2015; Santarpia et al. Citation2012) was used to measure the fluorescence spectra of individual bioaerosol particles withdrawn from the rotating drum at particular time periods throughout each aging experiment. The SPFS provided dispersed fluorescence spectra in 280–560 nm excited by a 263-nm laser pulse, fluorescence spectra in 380–650 nm excited by a 351-nm laser pulse, and an elastic scattering particle size illuminated by a 685 nm laser. An ultraviolet aerodynamic particle sizer (UV-APS, Model #3312, TSI Inc.) measured the total fluorescence (430–580 nm) of aerosols excited by a 355 nm laser and the aerodynamic size of the aerosol between 0.523 and 20 μm, which supplied the aerosol concentration and size distribution during each experiment to normalize biological losses for physical losses (described below). Air was sampled into the UV-APS at 1 L/min directly from the rotating drum with 4 L/min of sheath air being drawn from the surrounding laboratory. The SPFS measured fluorescence intensity for each particle was normalized with its size by dividing a factor d2.05 (d is the diameter of the particle) for 263-nm excitation and d2.8 for 351 nm excitation. The values 2.05 and 2.8 were estimated from the calculations of fluorescence verse diameter for dry homogeneous spherical particles composed of bacteria (Pan et al. Citation2014; Ratnesar-Shumate et al. Citation2015).
2.8. Biological quantification
Aerosol samples were collected at the beginning and end of each experiment via AGI-30s (Ace Glass Inc.) with 20 mL of phosphate-buffered saline (PBS) sampling at an airflow rate of 12.5 L/min. Each sample was analyzed for bacterial viability and for changes in DNA detection signature by quantitative polymerase chain reaction (qPCR). To determine viability, samples were serially diluted in PBS and plated in triplicate on 3 M Petrifilm. Since Petrifilm does not allow an observation of colony morphology, a single LB agar plate was used in parallel to ensure that aerosol samples contained only the organism of interest, and not previously tested or environmental aerosol contaminants. All samples were immediately frozen after culture, and then thawed together for DNA isolation using a Qiagen QIAamp DNA Mini Kit. Primers and probes were based on an existing published K-12-specific assay (Lu et al. Citation2014). Quantifications and PCR efficiency were based on a serially diluted standard curve from the test E. coli stock.
Finally, quantification by viable counts and qPCR results were normalized to particle concentrations in the drum, to compensate for the varying levels of particle loss that depended on experimental conditions and the sample time. Specifically, the mass concentration of aerosols from the UV-APS was used. Therefore, log decay rate normalized to aerosol concentration was calculated as,
(2)
(2)
where aerosol concentration C was determined by qPCR or culture, mass aerosol concentration n is determined by UV-APS; t is the time point for the second AGI sampling, which is equal to the aging time of aerosol within the chamber; and the subscripts 0 and 1 indicate the initial and final aerosol sampling time point, respectively.
2.9. Experimental process
To determine the effect of each variable, either individually or in combination with other variables, both a zero and a target concentration of each variable were chosen to perform the experiment. In total, two VOCs, SS radiation, ozone, and two relative humidity values, made the combination of these variables into 24 test conditions. Each of these conditions was repeated one to four times (). Each experiment lasted at an average of 2.75 h, not including the time required for conditioning and purging the chamber. The original goal was 3 replicates of variables predicted to have combinatory effects, e.g., toluene with UV light, instead of toluene and ozone, which reacts much slower. Combinations that had less replicates were added at the end of the experimental process after first completing replicates for predicted interactions and combinatory effects. These samples were pooled with experiments having similar conditions for statistical calculations. Toluene and α-pinene were never combined in the same experiment during this study. During each experiment, E. coli aerosols were generated for ∼10 min to reach a particle concentration of 200 particles/cm3 in the chamber. Immediately following chemical conditioning and aerosol generation, initial measurements were made with the UV-APS, SPFS, and an initial sample was collected with the AGI-30 for 5 min at 12.5 L/min (60 L of air total). Throughout each experiment, UV-APS and SPFS measurements were recorded every 30 min. UV-APS samples were collected for 3 min using a 30 s sampling cycle at 1 L/min. The SPFS sampled at 1 L/min to record 200 fluorescence spectra from individual particles for both 263-nm and 351-nm excitations associated with its elastic scattering determined size during each measurement. The time to obtain 200 spectra at each measurement varied from sample to sample, depending on the aerosol concentration in the chamber. During the experimental period, ozone, RH, and VOC concentrations were adjusted, as described previously to maintain their preset values. Each time aerosol sample was drawn from the drum chamber, the population was effectively diluted due to the influx of particle-free air to maintain pressure equilibrium. To maintain a representative population for each experiment, AGI-30 samples were only taken at the start and end of each experiment due to the high flow rate required for sampling. At the end of each experiment, the drum was purged at 55 L/min for 30 min, until the aerosol concentration was below 1% of its initial value.
Table 1. Summary of test replicates performed in this study.
3. Results and discussions
3.1. Changes in fluorescence intensity and profile
The averaged fluorescence spectra excited by 263 nm and 351 nm laser from 200 E. coli aerosol particles at different times, measured by SPFS during these experiments, are shown in . Each representative spectrum shown here is the averaged data from 200 spectra recorded during a single measurement period. Only spectra collected at t = 0, t = 30 min, and then 1 h intervals thereafter are presented. This approach demonstrates the rapid spectral changes observed in the first hour of many experiments, while limiting the visual overlap as the rate of change decreases over the remainder of the experiment. In addition to the spectra, the integrated fluorescence intensity over three emission bands are plotted versus time for all measurements (shown in the right column in ). These averaged emission bands clearly illustrate the trends in fluorescence amplitudes. The UV263 is the integrated fluorescence band intensity from 280 to 400 nm when excited by a 263 nm laser. Vis263 represents the integrated fluorescence band intensity from 400 to 580 nm when excited by a 263 nm laser. Last, Vis351 is the integrated fluorescence band intensity from 380 to 650 nm when excited by a 351 nm laser.
Figure 3. Time-dependent change of fluorescence spectra and the corresponding integrated band intensities from aerosolized E. coli cells under various conditions in the rotational drum with only one variable applied, these variables were high relative humidity (high RH∼75%), ozone (∼100 ppb), α-pinene (∼5 ppb), toluene (∼45 ppb), or SS illumination. The drum is kept at RH∼25% if no high RH is applied. The three fluorescence bands are the integrated intensity from fluorescence defined as UV263: band 280–400 nm excited by 263 nm laser; Vis263: band 400–580 nm excited by 263 nm laser; and Vis351: band 380–650 nm excited by 351 nm laser. The fluorescence spectra are the averaged data from one of the replicated tests. The integrated band intensities are the averaged data from all measurements (from different days’ replicated tests at the same conditions), and each test was collected from 200 individual E. coli aerosol particles.
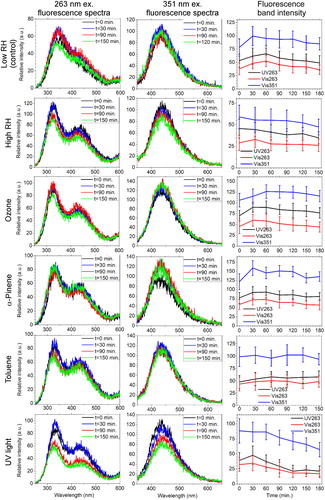
Figure 4. Time-dependent change of fluorescence spectra and the corresponding integrated band intensities from aerosolized E. coli cells under various conditions in the rotational drum with two variables applied, these variables were high relative humidity (high RH∼75%), ozone (∼100 ppb), α-pinene (∼5 ppb), toluene (∼45 ppb), or SS illumination. The drum is kept at RH∼25% if no high RH is applied. The three fluorescence bands are the integrated intensity from fluorescence defined as UV263: band 280–400 nm excited by 263 nm laser; Vis263: band 400–580 nm excited by 263 nm laser; and Vis351: band 380–650 nm excited by 351 nm laser. The fluorescence spectra are the averaged data from one of the replicated tests. The band intensities are the averaged data from all measurements (from different days’ tests at the same conditions), and each test was collected from 200 individual E. coli aerosol particles.
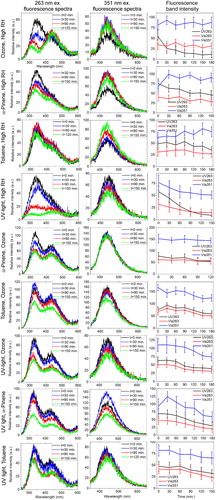
Figure 5. Time-dependent change of fluorescence spectra and the corresponding integrated band intensities from aerosolized E. coli cells under various conditions in the rotational drum with the combinations of three or four variables applied, these variables were high relative humidity (high RH∼75%), ozone (∼100 ppb), α-pinene (∼5 ppb), toluene (∼45 ppb), or SS illumination. The three fluorescence bands are the integrated intensity from fluorescence defined as UV263: band 280–400 nm excited by 263 nm laser; Vis263: band 400–580 nm excited by 263 nm laser; and Vis351: band 380–650 nm excited by 351 nm laser. The fluorescence spectra are the averaged data from one of the replicated tests. The integrated band intensities are the averaged data from all measurements (from different days’ tests at the same conditions), and each test was collected from 200 individual E. coli aerosol particles.
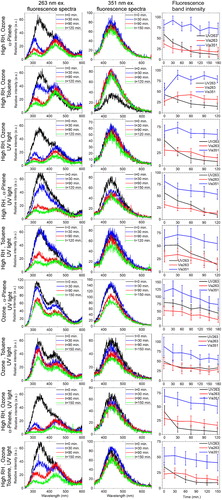
Initially, the time-dependent change of fluorescence spectra and the integrated band intensities during experiments where E. coli particles were exposed to only one variable (ozone, RH, VOC, or simulated solar radiation [SS]). The spectra in (all experiments with only one variable applied) show limited spectral profile changes, but different intensity variations during the test period. There was a strong intensity decrease in all three fluorescence bands when SS irradiation was applied, but only small degree of change in the other cases. Therefore, SS irradiation had the strongest individual degradation effect on the fluorescence of E. coli aerosols. The fluorescence intensity in the visible band excited at 351 nm (Vis351) showed a slight increase when ozone, or VOCs were applied. In addition, the UV fluorescence intensity excited at 263 nm (UV263) slightly increased when toluene was applied. However, it has been reported that toluene (Zabeti et al. Citation2015) and α-pinene (Lee et al. Citation2013) exhibit fluorescence emission in UV and visible, respectively, so the observed increases may be due to the fluorescence emission from the VOCs themselves and not totally necessarily representative of a reaction with the E. coli particles.
The time-dependent change of fluorescence spectra and integrated band intensities where two variables were applied in combination are shown in . These data show that the combination of two variables had more pronounced and combinatory effects on the fluorescence. The first four rows in show how ozone, VOCs, and SS irradiation changed the fluorescence when combined with high RH. Combination of “ozone + high RH” showed the change similar to that observed from other studies (Pan et al. Citation2014; Ratnesar-Shumate et al. Citation2015; Santarpia et al. Citation2012) where UV263 dramatically decreased, but Vis263 increased slightly and Vis351 increased more prominently. The fluorescence in the UV263 band is mainly due to tryptophan, which is oxidized by ozone at high RH and transformed into NF and KY (Fujimori Citation1985; Ignatenko Citation1988; Pan et al. Citation2014; Ratnesar-Shumate et al. Citation2015; Santarpia et al. Citation2012), which has strong visible fluorescence emission by 351 nm excitation (Vis351). When either VOC was combined with high RH, both UV263 and Vis263 bands decreased slightly, but the Vis351 band increased in intensity. For “SS irradiation + high RH,” all three fluorescence bands decreased, especially UV263. The last five rows in showed the effects from the combination of ozone, VOCs, and SS irradiation. The UV263 and Vis263 fluorescence bands in the five cases decreased considerably with similar small spectral profile changes. However, fluorescence spectra excited by 351 nm excitation changed differently, depending on conditions, suggesting that multiple, different processes could be involved. There was little intensity and profile change during “α-pinene + ozone” experiments, but some decrease for “toluene + ozone” experiments. The decrease in the intensity of Vis351 was similar for “toluene + SS” and “ozone + SS,” but the strongest decrease was observed for “α-pinene + SS.”
Finally, the time-dependent change of fluorescence spectra and integrated band intensities with three or four variables are shown in . For the conditions with “ozone + high RH + one variable” as shown in row 1, 4, and 5, the effects were similar to the condition with “ozone + high RH” shown at row 1 in . This indicates that in these three cases, ozone and high RH, in combination, dominated the aging process, even with another variable added. In these three cases, the UV263 bands significantly decreased, but with only minor changes in Vis263 bands. The Vis351 bands had small changes for “ozone + high RH + SS” and “ozone + high RH + α-pinene” (rows 1 and 5), but had a significant increase for “ozone + high RH + toluene” (row 4). For these conditions with “high RH + VOC + SS,” as shown in row 2 and 3, the fluorescence intensities from all bands decreased, especially the UV263 band. For the “ozone + VOC + SS” conditions, as shown in row 6 and 7, the fluorescence intensities from all bands decreased at a similar rate, but the spectral profiles had little change during the aging process. This implies three variables: ozone, VOC, and SS light at low RH worked in combination to impact all the fluorescent molecules at a similar rate. Rows 8 and 9 in show the results from the most complex experimental conditions with four variables (SS + ozone + high RH + VOC) applied. The fluorescence intensities from all bands decreased; however, the UV263 bands still showed the largest decrease, while the Vis263 and Vis351 fluorescence bands had a similar smaller rate of decrease.
3.2. Changes in viability and qPCR signal
During the same experiments, the viability and the qPCR signal intensity of E. coli aerosol particles were monitored by assaying the samples collected by the AGI-30. Similar to the fluorescence measurements, the viability and qPCR signal intensity displayed small changes in experiments with only one variable applied (. Overall, small amounts of viability loss (average values between −0.42 and −0.85 (log CFU/h) were measured with limited differences between them. However, the qPCR signal intensity decreased more rapidly when exposed to SS and ozone (−0.20 and −0.16 quantification cycle per hour (Cq/h) as compared to −0.06 to −0.07 Cq/h for other variables applied).
Figure 6. (a) The averaged log rates and standard deviations for the change of E. coli viability and the qPCR signal intensity. (b) Time-averaged change rates and standard deviations for the three fluorescence bands UV263, Vis263, and Vis351 under various conditions in the rotating drum with one, the combinations of two, three, or four of the following variables applied: high RH (RH∼75%), ozone (∼100 ppb), α-pinene (∼5 ppb), toluene (∼45 ppb), and SS (simulated solar illumination from mercury halide lamp).
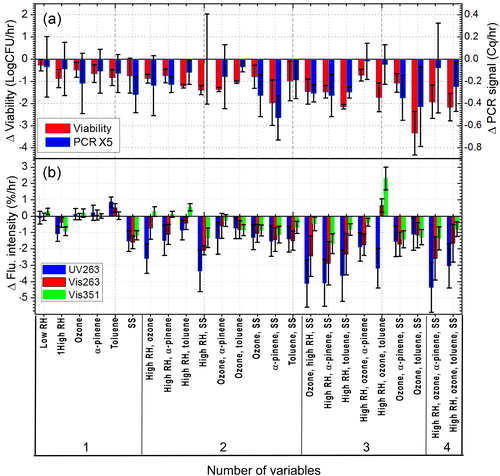
In the experiments with a combinations of two variables applied, both viability and qPCR signal displayed more pronounced changes than when only one variable was applied (. Average decreases in viability between −0.62 and −1.78 log CFU/h were observed, with the combination of SS and toluene producing the largest decrease. The qPCR signal intensity contained more variability, ranging between an increase of 0.02 Cq/h to a maximum decrease of −0.34 Cq/h. The largest decreases were from the combinations of SS and VOCs (either toluene [−0.34 Cq/h] or α-pinene [−0.23 Cq/h]).
Finally, experiments combining 3 and 4 variables produced even stronger changes in the viability and qPCR signal intensity (. Average decreases in viability ranged from −0.45 to −2.02 log CFU/h for the nine experiments. The combination of SS, toluene, and high RH produced the largest decreases in viability with the presence of ozone (−1.96 log CFU/h) and without ozone (−2.02 log CFU/h). However, due to the variability in replicate experiments, these two cannot be discriminated from one another. The highest rate of decrease in qPCR signal was observed for experiments with the combinations of SS, α-pinene, and high RH (−0.29 Cq/h).
3.3. Comparison of biological and fluorescence measurements
To quantitatively compare these changes under different conditions and to make more direct comparisons with the biological aging results, the intensity change rates of the three fluorescence bands were calculated as,
(3)
(3)
where I is the integrated fluorescence band intensity; t is the time point for the last measurement in the experiment, which is equal to the aging time of aerosol within the chamber; and the subscripts 0 and 1 indicates the initial and final aerosol sampling time point, respectively. It is important to note that this is the change averaged over the entire interaction time, which does not precisely reflect the nonlinear aging process where most aging occurs in the first 30 min (). However, it is still valuable for comparing aging between fluorescence, viability, and qPCR sensitivity. summarizes the average intensity change of the three fluorescence bands under the different experimental conditions. There is considerable variability, but several trends are apparent. The UV263 band typically decreased at the highest rate, and its intensity only slightly increased during limited single variable experiments (ozone or VOC). The Vis263 band typically followed the changes in UV263 (increase or decrease simultaneously) but with a smaller rate of change. Vis263 and UV263 only changed in opposite directions during ozone + high RH + toluene experiments. The Vis351 bands had the smallest rates of change, and in quite a few cases, their intensities increased, especially when ozone, high RH, and VOC were applied.
Additional visual examination of provides additional information. First, the decay of fluorescence and qPCR signals did not appear directly related to a loss in viability. Attempts to correlate the decay rates of both fluorescence and qPCR signal to the decay rates of viability produced disappointing results (R2=0.23, 0.14, 0.08, and 0.17 for UV263, Vis263, Vis351, and qPCR, respectively). The UV263 fluorescence is primarily due to tryptophan and other aromatic amino acids (Santarpia et al. Citation2012; Pan Citation2015), and as such should be a good marker for protein degradation, and therefore offers some predictability of viability degradation. Vis263 fluorescence has contribution of fluorescence due to amino acids but is primarily related to flavins (flavin mononucleotide (FMN) and flavin adenine dinucleotide [FAD]) and metabolic by-products. Vis351 fluorescence is dominated by the reduced form of nicotinamide adenine dinucleotide (NADH) and its phosphate (NADPH), flavins, and metabolic products (Santarpia et al. Citation2012; Pan Citation2015). Therefore, both Vis263 and Vis351 bands may not directly correlate to the viability of the organism when it is not active metabolically. When comparing the viability decay to fluorescence decay, it is also important to note that residual media components may also be fluorescent, which could impact this comparison. Decay in the qPCR signal intensity should also be a good marker for DNA damage, and therefore be related to viability. However, the lack of a statistically relationship between fluorescence, qPCR, and viability decay rates is likely due to the variety of factors including the complex nature of the particles and the complexity of environmental processes occurring in these experiments, but may indicate that decay in viability is not associated with degradation of the genomic material.
To better understand the decay processes of E. coli particles involved in these experiments, the role of each variable was examined across all experiments. Since each variable had only two levels, test data could be pooled so that the role of each variable could be compared across combinations of all the other variables. Paired value t tests, allowing a two-tailed p value, were performed for each variable (and select pairs of variables) across all experimental conditions where the only difference in the experiments being compared was a change in the variable being tested. The calculated p values are indicative of the significance of each variable or variable pair to the observed decay across the experimental conditions (). It is clear that SS plays the dominant role in the decay of qPCR signal. DNA and protein damage due to UV exposure is a well-known mechanism, our observations are in good agreement with the published literature (Tak et al. Citation2011). There are variable combinations involving SS that also appear significant or potentially significant (SS + ozone and SS + toluene) but it is unclear if their importance is exclusively related to the presence of SS. Viability and both fluorescence bands excited at 263 nm indicate that multiple processes are responsible for their decay. Given the significance of some of the combined variables, it is possible that other factors not measured in these experiments may be involved in the observed decay. For instance, the combination of high RH and SS would produce more OH radicals which, in turn, would lead to the loss of viability. As well, it has been established by previous studies (Pan et al. Citation2014) that high RH is necessary to complete hydrolysis of the ozonolysis product of tryptophan and reduce the fluorescence of the UV263 band, so the significance of this combination, in the decay of UV263, is also not surprising. The organic vapors (toluene and α-pinene) significantly contributed to the decay of viability and the 263-nm excited fluorescence bands when combined with SS. This indicates that either the formation of secondary organic aerosol or the generation of ozone (or possibly both) was involved in the decay. It is also noteworthy that the difference between aging with toluene or α-pinene does not appear significant, indicating the chemical process responsible for the observed aging between these compounds is likely similar, even though their functions and concentrations were different.
Table 2. Summary of p values from t tests examining the impact of single variables and pairs of variables on the observed changes in all fluorescence and biological measurements.
The decay rates of the three fluorescence bands, the viability, the qPCR signature and the inconsistencies between them for the E. coli aerosol particles in these experiments clearly indicate that multiple processes are responsible for the decay of proteins, DNA, and ultimately organism viability, and that neither qPCR nor fluorescence measurements is a clear indicator of viability. Even using qPCR-based detection for enumeration may be skewed if light, ozone, VOCs, or other atmospheric constituents destroy extracellular DNA more rapidly than intracellular materials. Additional studies are needed to further understand the mechanisms by which atmospheric chemical processes impact viability and detectability of biological aerosol. The changes in fluorescence and viability observed in the presence of high RH, ozone, VOC, and SS light indicate that biological aerosols can be dramatically altered while remaining aloft in the atmospheric environment. The fluorescence amplitudes and spectral profiles of bioaerosols could be significantly changed in tens of minutes after they are released into the atmosphere. Therefore, the aging processes under different atmospheric conditions must be taken into consideration for any fluorescence-based technologies aimed at detecting and characterizing bioaerosols.
4. Summary
The detection and characterization of biological aerosols in the atmosphere continues to be a challenging task. Most biosensors rely on the presence of specific molecules in bioaerosol particles, such as antigens on the surface, DNA sequences, or the common fluorescent molecules tryptophan, flavins, or NADH. However, the detection signatures from any of these technologies can change significantly when the bioaerosols are released into the atmosphere, and the changes are dependent upon the local environmental conditions. Detection signals will decrease in most cases, with various rates, but can also increase (as in the case of visible fluorescence by 351 nm excitation). In developing and testing bioaerosol detection methods, researchers have to account for the potential changes in the physical, chemical, and biological properties of bioaerosols caused by various atmospheric conditions. Testing infectious bioaerosols in a controlled BSL-2 or BSL-3 laboratory environments may underestimate the complex environmental conditions that VOCs and combinatory effects of VOCs and other environmental factors have. The experimental results presented here show how the fluorescence spectral profile and intensity, the viability, and the qPCR signal intensity of E. coli aerosol particles change with time in the presence of one, or the combinations of two, three, or four of the following variables: low and high relative humidity, ozone at 100 ppb, the VOCs α-pinene (∼5 ppb) or toluene (∼45 ppb), and SS radiation (light illumination). These variable levels are typical in urban or suburban areas, they can result in observed changes such as UV fluorescence intensity (UV263) excited by 263 nm laser reducing to be <1/10 of its initial value, or the ratio of UV/visible fluorescence intensity (UV263/Vis263) changing from 2 to ½ within 3 h. Additionally, based on the relationship between increasing environmental complexity and the decay rate of the biological properties, the decay rate of biological aerosol in real atmosphere may be significantly higher than observed in these studies. Such a correlation also tells us that the presence of additional chemical mechanisms for the aging process should be identified. Further, no clear relationship between E. coli aerosol viability and fluorescence or qPCR signal intensity could be identified in this study, indicating that detectability and infectivity are often not linked. The atmospheric trace gas species and concentrations used in this study are intended to be representative of what might be found in urban and near-urban environments in the U.S. and throughout the world. Therefore, the changes in biological properties demonstrated in this study should be considered typical and possibly conservative estimates of aging in real atmosphere. The community should work to further understand the breadth of mechanisms responsible for the observed decay, include these aging effects in the interpretation of ambient data and algorithm development for instrumentation, and incorporate it into predictive models and look for ways to improve the robustness of ambient biological measurement.
Additional information
Funding
References
- Aptowicz, K. B., Y. L. Pan, S. D. Martin, E. Fernandez, R. K. Chang, and R. G. Pinnick. 2013. Decomposition of atmospheric aerosol phase function by particle size and asphericity from measurements of single particle optical scattering patterns. J. Quant. Spectrosc. Radiat. Transf. 131:13–23. doi:10.1016/j.jqsrt.2013.03.020.
- Ariya, P. A., and M. Amyot. 2004. New directions: the role of bioaerosols in atmospheric chemistry and physics. Atmos. Environ. 38(8):1231–1232. doi:10.1016/j.atmosenv.2003.12.006.
- Cataldo, F. 2006. DNA degradation with ozone. Int. J. Biol. Macromol. 38(3–5):248–254. doi:10.1016/j.ijbiomac.2006.02.029.
- Cliff, J. B., K. H. Jarman, N. B. Valentine, S. L. Golledge, D. J. Gaspar, D. S. Wunschel, and K. L. Wahl. 2005. Differentiation of spores of Bacillus subtilis grown in different media by elemental characterization using time-of-flight secondary ion mass spectrometry. Appl. Environ. Microbiol. 71(11):6524–6530. doi:10.1128/AEM.71.11.6524-6530.2005.
- Cox, C. S., A. M. Hood, and J. Baxter. 1973. Method for comparing concentrations of the open-air factor. Appl. Microbiol. 26(4):640–642.
- Deguillaume, L., M. Leriche, P. Amato, P. A. Ariya, A.-M. Delort, U. Poschl, N. Chaumerliac, H. Bauer, A. I. Flossmann, and C. E. Morris. 2008. Microbiology and atmospheric processes: chemical interactions of primary biological aerosols. Biogeosciences 5(4):1073–1084. doi:10.5194/bg-5-1073-2008.
- Dennis-Smither, B. J., F. H. Marshall, R. E. Miles, T. C. Preston, and J. P. Reid. 2014. Volatility and oxidative aging of aqueous maleic acid aerosol droplets and the dependence on relative humidity. J. Phys. Chem. A 118(30):5680–5691. doi:10.1021/jp504823j.
- Fan, L., J. Song, P. D. Hildebrand, and C. F. Forney. 2002. Interaction of ozone and negative air ions to control micro-organisms. J. Appl. Microbiol. 93(1):144–148.
- Fujimori, E. 1985. Changes induced by ozone and ultraviolet light in type i collagen. Bovine achilles tendon collagen versus rat tail tendon collagen. Eur. J. Biochem. 152(2):299–306. doi:10.1111/j.1432-1033.1985.tb09198.x.
- Gatzche, K., Y. Iinuma, A. Tilgner, A. Mutzel, T. Berndt, and R. Wolke. 2017. Kinetic modeling studies of SOA formation from a-pinene ozonolysis. Atmos. Chem. Phys. 17:13187–13211. doi:10.5194/acp-17-13187-2017.
- Goodwin, R. D. 1989. Toluene thermophysical properties from 178 to 800k at pressures to 1000 bar. J. Phys. Chem. Ref. Data 18(4):1565–1636. doi:10.1063/1.555837.
- Hildebrandt Ruiz, L., A. L. Paciga, K. M. Cerully, A. Nenes, N. M. Donahue, and S. N. Pandis. 2015. Formation and aging of secondary organic aerosol from toluene: changes in chemical composition, volatility and hygroscopy. Atmos. Chem. Phys. 15(14):8301–8313. doi:10.5194/acp-15-8301-2015.
- Hinds, W. C. 1999. Aerosol technology: properties, behavior and measurement of airborne particles. New York: Wiley.
- Huang, R.-J., Y. Zhang, C. Bozzetti, K.-F. Ho, J.-J. Cao, Y. Han, K. R. Daellenbach, J. G. Slowik, S. M. Platt, F. Canonaco, P. Zotter, R. Wolf, S. M. Pieber, E. A. Bruns, M. Crippa, G. Ciarelli, A. Piazzalunga, M. Schwikowski, G. Abbaszade, J. Schnelle-Kreis, R. Zimmermann, Z. An, S. Szidat, U. Baltensperger, I. E. Haddad, and A. S. H. Prévôt. 2014. High secondary aerosol contribution to particulate pollution during haze events in china. Nature 514(7521):218–222. doi:10.1038/nature13774.
- Ignatenko, A. V. 1988. Use of the method of tryptophan fluorescence to characterize disruptions of the structure of ozonized proteins. J. Appl. Spectrosc. 49(1):691–695. doi:10.1007/BF00662905.
- Kanaani, H., M. Hargreaves, Z. Ristovski, and L. Morawska. 2007. Performance assessment of UVAPS: influence of fungal spore age and air exposure. J. Aerosol Sci. 38(1):83–96. doi:10.1016/j.jaerosci.2006.10.003.
- Krieger, U. K., C. Marcolli, and J. P. Reid. 2012. Exploring the complexity of aerosol particle properties and processes using single particle techniques. Chem. Soc. Rev. 41(19):6631–6662. doi:10.1039/c2cs35082c.
- Lee, H. J., A. Laskin, J. Laskin, and S. A. Nizkorodov. 2013. Excitation-emission spectra and fluorescence quantum yields for fresh and aged biogenic secondary organic aerosols. Environ. Sci. Technol. 47(11):5763–5770. doi:10.1021/es400644c.
- Loh, N. D., C. Y. Hampton, A. V. Martin, D. Starodub, R. G. Sierra, A. Barty, A. Aquila, J. Schulz, L. Lomb, J. Steinbrener, R. L. Shoeman, S. Kassemeyer, C. Bostedt, J. Bozek, S. W. Epp, B. Erk, R. Hartmann, D. Rolles, A. Rudenko, B. Rudek, L. Foucar, N. Kimmel, G. Weidenspointner, G. Hauser, P. Holl, E. Pedersoli, M. Liang, M. S. Hunter, L. Gumprecht, N. Coppola, C. Wunderer, H. Graafsma, F. R. Maia, T. Ekeberg, M. Hantke, H. Fleckenstein, H. Hirsemann, K. Nass, T. A. White, H. J. Tobias, G. R. Farquar, W. H. Benner, S. P. Hau-Riege, C. Reich, A. Hartmann, H. Soltau, S. Marchesini, S. Bajt, M. Barthelmess, P. Bucksbaum, K. O. Hodgson, L. Struder, J. Ullrich, M. Frank, I. Schlichting, H. N. Chapman, and M. J. Bogan. 2012. Fractal morphology, imaging and mass spectrometry of single aerosol particles in flight. Nature 486(7404):513–517. doi:10.1038/nature11222.
- Lu, J., T. L. Gerke, H. Y. Buse, and N. J. Ashbolt. 2014. Development of an Escherichia coli k12-specific quantitative polymerase chain reaction assay and DNA isolation suited to biofilms associated with iron drinking water pipe corrosion products. J. Water Health 12(4):763–771. doi:10.2166/wh.2014.203.
- May, K. R., H. A. Druett, and L. P. Packman. 1969. Toxicity of open air to a variety of microorganisms. Nature 221(5186):1146–1147. doi:10.1038/2211146a0.
- Pan, Y. L. 2015. Detection and characterization of biological and other organic-carbon aerosol particles in atmosphere using fluorescence. J. Quant. Spectrosc. Radiat. Transf. 150:12–35. doi:10.1016/j.jqsrt.2014.06.007.
- Pan, Y. L., J. L. Santarpia, S. Ratnesar-Shumate, E. Corson, J. Eshbaugh, S. C. Hill, C. C. Williamson, M. Coleman, C. Bare, and S. Kinahan. 2014. Effects of ozone and relative humidity on fluorescence spectra of octapeptide bioaerosol particles. J. Quant. Spectrosc. Radiat. Transf. 133:538–550. doi:10.1016/j.jqsrt.2013.09.017.
- Ratnesar-Shumate, S.,. Y. L. Pan, S. C. Hill, S. Kinahan, E. Corson, J. Eshbaugh, and J. L. Santarpia. 2015. Fluorescence spectra and biological activity of aerosolized bacillus spores and ms2 bacteriophage exposed to ozone at different relative humidities in a rotating drum. J. Quant. Spectrosc. Radiat. Transf. 153:13–28. doi:10.1016/j.jqsrt.2014.10.003.
- Redding, B., M. Schwab, and Y. L. Pan. 2015. Raman spectroscopy of optically trapped single biological micro-particles. Sensors (Basel) 15(8):19021–19046. doi:10.3390/s150819021.
- Rosenfeld, D., S. Sherwood, R. Wood, and L. Donner. 2014. Atmospheric science. Climate effects of aerosol-cloud interactions. Science 343(6169):379–380. doi:10.1126/science.1247490.
- Santarpia, J. L., Y. L. Pan, S. C. Hill, N. Baker, B. Cottrell, L. McKee, S. Ratnesar-Shumate, and R. G. Pinnick. 2012. Changes in fluorescence spectra of bioaerosols exposed to ozone in a laboratory reaction chamber to simulate atmospheric aging. Opt. Express 20(28):29867–29881. doi:10.1364/OE.20.029867.
- Selma, M. V., A. M. Ibanez, M. Cantwell, and T. Suslow. 2008. Reduction by gaseous ozone of salmonella and microbial flora associated with fresh-cut cantaloupe. Food Microbiol. 25(4):558–565. doi:10.1016/j.fm.2008.02.006.
- Tak, Y. K., W. Y. Kim, E. Han, M. J. Kim, J. A. Kim, C. Y. Lim, and J. M. Song. 2011. Determination of UV-induced DNA damages to suppress protein expression using reporter gene assay-based single cell cotransfection imaging cytometry. Toxicology Lett. 204(1):25–31. doi:10.1016/j.toxlet.2011.03.032.
- Zabeti, S., A. Drakon, S. Faust, T. Dreier, O. Welz, M. Fikri, and C. Schulz. 2015. Temporally and spectrally resolved UV absorption and laser-induced fluorescence measurements during the pyrolysis of toluene behind reflected shock waves. Appl. Phys. B 118(2):295–307. doi:10.1007/s00340-014-5986-8.