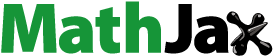
Abstract
Previous laboratory studies of Ebola virus aerosols have used a variety of different sampling devices to collect viral material for analysis. However, sampling devices vary in their ability to collect aerosol particles and preserve the infectivity of collected viruses. If not accounted for, this variability has the potential to bias study outcomes and confound comparisons between studies in which different samplers are used. In the present study, five laboratory-scale aerosol samplers were assessed for their ability to collect and preserve the infectivity of Ebola virus at low and high relative humidities. Relative humidity did not affect physical collection efficiencies, but infectivity was preserved better at high relative humidity for most samplers. Overall, 25-mm gelatin filters were identified as the optimal sampler among those tested based on their physical and biological efficiency, ease of use, lack of glass components, and ability to be recovered in small volumes to produce a more concentrated final sample. Tests demonstrated that 25-mm gelatin filters recovered in 2.5-mL media and assayed by microtitration using an Ebola reporter cell line resulted in an approximately 10-fold improvement in sensitivity compared to other published methods. The data provided by this study will be useful to facilitate comparisons between different studies utilizing aerosolized Ebola virus and to inform sampler selection in future studies.
Introduction
Human-to-human transmission of Ebola virus (EBOV) is thought to occur primarily through direct contact with body fluids of infected patients (Chowell and Nishiura Citation2014; Mate et al. Citation2015). While there is little epidemiological evidence that transmission via aerosols contributes to the spread of the virus during outbreaks, some human Ebola virus disease (EVD) cases are documented with no such instances of close contact (Roels et al. Citation1999). While these cases may simply reflect incomplete case documentation, it is also possible that they reflect a subset of transmission events mediated by small droplets or aerosols. EBOV RNA has been detected in air samples in biocontainment laboratories housing infected non-human-primates (Harbourt et al. Citation2017), and laboratory animals have become infected with EBOV after being housed in the same facility as infected animals but with no direct contact, suggesting the possibility of aerosol transmission (Jaax et al. Citation1995; Weingartl et al. Citation2012). Additionally, laboratory studies have shown that the virus can remain infectious in aerosols for over an hour (Belanov et al. Citation1996; Piercy et al. Citation2010; Schuit et al. Citation2016), and that low doses of aerosolized virus can produce lethal infection in nonhuman primates. While it is unknown if EBOV-containing aerosols can be generated from the respiratory tract of infected patients, certain medical interventions, and even routine actions such as flushing toilets, have the potential to generate virus-containing aerosols that may spread disease from infected to uninfected individuals (Alsved et al. Citation2020; Davies et al. Citation2009; Johnson et al. Citation2013; Judson and Munster Citation2019). The incomplete epidemiological record, combined with the documented transmission and prolonged survival in aerosols in laboratory settings, suggest that aerosol transmission may be feasible under some circumstances. Furthermore, the high rates of morbidity and mortality associated with human cases of EVD have contributed to concerns that this virus could be used as an agent of bioterrorism, with historical precedents for attempted weaponization of both EBOV and the closely related Marburg virus (MARV) (Alibek Citation2008; Borio et al. Citation2002; Cenciarelli et al. Citation2015). A bioterrorism incident involving EBOV has the potential for infection via inhalation outside of natural transmission pathways.
However, assessment of the possibility of EBOV aerosol transmission and its associated risk is complicated by the difficulty of quantifying low levels of infectious virus in aerosols, whether in laboratory or clinical settings, due to limitations of commonly used methodologies. While inhaled doses of both MARV and EBOV as low as two plaque forming units (PFU) have been shown to be lethal in nonhuman primate models of disease (Alves et al. Citation2010; Reed et al. Citation2011), it has not been possible to determine the complete dose-response relationship for either virus due to the inability to quantify lower levels of infectious virus using standard methods. This problem also has ramifications for studies examining the decay of EBOV infectivity in aerosols (Fischer et al. Citation2016; Piercy et al. Citation2010; Smither et al. Citation2011), especially under environmental conditions where the decay is rapid and accurate determination of decay rates depends on quantifying of increasingly low levels of virus.
Studies of biological aerosols depend upon the use of aerosol samplers to collect particles from the air into a suitable medium for assay. However, aerosol samplers can vary widely in their abilities to collect and retain aerosol particles and preserve the biological activity of collected microorganisms. This variability may be due to factors affecting the physical collection of particles, which are often size dependent, or microorganism-specific sensitivities to the stresses imparted by different sampling methodologies, such as desiccation on a dry substrate, rapid rehydration in a liquid substrate, or impaction on a solid substrate (Dybwad, Skogan, and Blatny Citation2014; Fabian et al. Citation2009; May and Harper Citation1957; Terzieva et al. Citation1996). These factors can significantly affect the results of the down-stream assays employed in a study, and, if not accounted for, may bias or confound the conclusions of a study. For example, Fabian et al. (Citation2009) demonstrated that titers of infectious influenza virus in aerosols measured with the SKC Biosampler, which collects into a liquid medium, are approximately an order of magnitude higher than titers measured for the same aerosol with a cascade impactor, a gelatin filter, or a Teflon filter. Thus, in a study with aerosolized influenza virus, the measured concentration of the aerosol could differ by nearly ten-fold depending on the sampler type utilized. A difference of this magnitude has the potential to affect the results of a variety of different studies involving bioaerosol sampling, including determinations of challenge doses delivered to animal models of disease and conclusions regarding the presence or absence of infectious viral aerosols in clinical settings.
Aerosol sampler selection should also take into account interactions between system variables such as relative humidity, the samplers involved, and the specific microorganism being sampled. Tseng et al. found that recovery from samplers varied as a function of relative humidity for several bacteriophages, in some cases changing which sampler would be considered optimal for quantifying virus concentrations (Tseng and Li Citation2005). With T7 phage, recovery at 55% relative humidity was highest with gelatin filters relative to three other samplers, and improved by approximately an order of magnitude when relative humidity was increased to 85%. However, recovery with an all glass impinger (AGI) improved by nearly 2.5 orders of magnitude with the same increase in relative humidity, making the AGI a better sampler for T7 under higher relative humidity conditions. In the same study, Φ6 phage displayed no such dependence on relative humidity. Other studies have demonstrated similar interactions between microorganisms, aerosol samplers, and the conditions under which they are operated (Dybwad, Skogan, and Blatny Citation2014; Henningson and Ahlberg Citation1994; May and Harper Citation1957). Such differences may confound comparisons between studies and have the potential to affect risk or hazard assessments which incorporate such data. In the context of a biological threat agent, such differences could have ramifications for hazard assessment, development of response protocols, and decisions regarding the development of countermeasures.
Previous studies examining the inhalational infectivity and persistence of aerosolized filoviruses have relied on liquid impingers to collect samples (Alves et al. Citation2010; Fischer et al. Citation2016; Piercy et al. Citation2010; Reed et al. Citation2011; Smither et al. Citation2015; Twenhafel et al. Citation2015). However, the efficiency of impingers relative to other samplers is variable and dependent on many of the aforementioned factors (Verreault, Moineau, and Duchaine Citation2008). Therefore, it is important to characterize the performance of potential sampling devices with the specific microorganism involved in a study to maximize sampling efficiency and to provide data that can be utilized to bridge to the results of studies utilizing different sampling devices. To date, no study directly comparing the performance of laboratory aerosol samplers with any filovirus has been published. Furthermore, no assessment on the effect of relative humidity on aerosol sampler performance for filoviruses has been reported. Therefore, the present study was conducted to compare the ability of several different common laboratory aerosol samplers to collect and preserve the infectivity of aerosolized EBOV at both low and high relative humidity levels. Data from the present study will be useful to inform the design of future EBOV aerosol studies, and to facilitate comparisons between previous studies that have used different types of aerosol samplers.
Methods
Virus
A passage one stock of Ebola virus/H. sapiens-tc/GIN/2014/Makona C07 (EBOV/Mak) in cell culture supernatant was kindly provided by Dr. Heinz Feldmann, Rocky Mountain Laboratory (RML)/NIAID/NIH. This material was passaged twice in Vero E6 cells to generate a passage 3 stock that was used for all testing in this study.
Cells and viral assays
Vero E6 cells (Chlorocebus sabaeus kidney; ATCC Vero C1008) were used to passage virus and production of virus stocks. Cells were maintained in complete growth media (gMEM) consisting of Minimum Essential Medium (MEM; Life Technologies) supplemented with 10% heat-inactivated fetal bovine serum (FBS; Atlanta Biologicals), 2 mM Glutamax (Life Technologies), 0.1 mM non-essential amino acids solution(NEAA; Life Technologies), 1 mM sodium pyruvate (Life Technologies) and 1% antibiotic-antimycotic solution (Life Technologies).
In most experiments, viral titers were measured by microtitration assay using a Vero E6 Ebola reporter cell line, Vero-piggy-EboZ ZSG min 5′ mg clone B8 (referred to as Vero-Ebola-reporter hereafter), kindly provided by Dr. Markus Kainulainen (Centers for Disease Control and Prevention, Atlanta, GA). These cells express a Zs-Green reporter following infection by Ebolaviruses and can be used for detection and quantitation of infectious EBOV in samples. Cells were maintained in DMEM (Life Technologies) supplemented as with gMEM, exempting 2 mM Glutamax and with the addition of 30 µg/mL of puromycin (Life Technologies) for positive selection of cells containing the integrated reporter construct. Cells plated for microtitration assays were supplied with Fluorobrite DMEM (Life Technologies), a medium with low background fluorescence. When used, Fluorobrite DMEM was supplemented with 10% heat-inactivated FBS, 1 mM sodium pyruvate, and 1% antibiotic-antimycotic solution (FB DMEM).
Microtitration assays were conducted by serially diluting samples on Vero-Ebola-reporter cells in black-walled 96 well plates using a dilution plating robot (BioTek Precision Microplate Pipetting System) with 100 µL of liquid per well. Plates were read with a Spectramax Paradigm plate reader following 14 days of incubation at 37 °C and 5% CO2. A sample well was considered positive for virus infection if its fluorescence value was above a threshold of four standard deviations above the mean fluorescence of the negative control wells. This threshold was selected during assay development as a value that maximized correlations between plate reader analyses and manual scoring by fluorescence microscopy. In some tests, plates were also read manually by brightfield and fluorescence microscopy, with wells scored positive for virus infection when the monolayer exhibited viral cytopathic effects (CPE) or at least one cell expressing Zs-Green, respectively. The median tissue culture infectious dose (TCID50) was calculated for each plate according to the method of Karber and Spearman (Finney Citation1964).
In a sub-set of experiments conducted to compare two different combinations of aerosol sampling and virus assay methods, a plaque assay developed by the Filovirus Animal Non-Clinical Group (FANG) and described by Shurtleff et al. (Shurtleff et al. Citation2012; Shurtleff et al. Citation2016) was used to quantify infectious EBOV in some samples. A lineage of Vero E6 cells (BEI Resources Cat NR-596), different from those used for virus stock production, was used for these assays as recommended by Shurtleff et al. (Citation2012). Plaque assays were conducted in six well plates as described previously (Shurtleff et al. Citation2012; Shurtleff et al. Citation2016). For this plaque assay protocol, it is recommended that virus titers be calculated using data from assay wells containing 10-150 plaques to ensure a robust correlation between the assay results and the actual virus titer within the sample. However, in the present study, no lower bound on the countable plaques was set in order to assess the linearity and lower limit of detection of this method of virus quantification. As a result, virus titers were calculated using as few as one plaque in three undiluted plaque assay wells for some samples.
Aerosol samplers
Five aerosol samplers commonly utilized in laboratory studies were compared in the present study: AGIs (Ace Glass Inc. Model 7541), operated at 6 L/min; Biosamplers (SKC Inc., PN: 225-9595), operated at 12.5 L/min; Midget Impingers (SKC Inc., PN: 225-36-1), operated at 1 L/min; 25 mm Gelatin Filters (Sartorius Stedim Biotech GmbH, PN:12602-25-ALK) in delrin filter holders (Pall Corp., PN 1109), operated at 1 L/min except where otherwise indicated; and the NIOSH BC251 Personal Bioaerosol Cyclone Sampler, operated at 3.5 L/min. The NIOSH BC251 was kindly provided by Dr. William Lindsley of the CDC/NIOSH. AGIs were selected as they have been commonly utilized to quantify aerosol concentrations in many studies examining either inhalational virulence or aerosol persistence (Alves et al. Citation2010; Fischer et al. Citation2016; Lin et al. Citation2000; Reed et al. Citation2011). Biosamplers are a cyclonic impinger specifically designed for collecting biological aerosols. While no comparative data exists for their performance with filoviruses, studies with other microorganisms have suggested that their performance is superior to that of other impingers (Burton, Grinshpun, and Reponen Citation2007; Fabian et al. Citation2009; Kesavan, Schepers, and McFarland Citation2010). Midget impingers are more compact than AGIs and have been used to quantify filovirus concentrations in a previous filovirus aerosol persistence study (Piercy et al. Citation2010). In contrast to both AGIs and Biosamplers, midget impingers do not require critical airflow during sampling. This lowers the velocity of collected particles and has the potential to reduce shear and impaction forces, potentially allowing for gentler collection of bioaerosols. Although similarly lacking comparative data with filoviruses, gelatin filters have been used for sampling virus aerosols in both field and laboratory bioaerosol studies (Tseng and Li Citation2005; Verreault et al. Citation2011; Zuo et al. Citation2013). Gelatin filters also contain no glass components, which is a significant safety consideration in the BSL-4 biocontainment environment required for work with filoviruses. The NIOSH BC251 was developed as an aerosol sampler for field and clinical settings and has been used successfully in several such applications (Bertran et al. Citation2017; Lindsley et al. Citation2010). It also contains no glass, and has an added functionality of fractionating the collected particles into three size ranges. Finally, an Aerodynamic Particle Sizer (APS; TSI Inc. Model 3321) equipped with a 1:20 diluter (TSI, Inc., Model 3302 A) was used to monitor the aerosol concentration and size distribution throughout the course of each experiment.
For EBOV sampler comparison tests, impinger samplers were loaded with FB DMEM prepared as described above, except without the addition of FBS, as the presence of FBS in an impinger can lead to significant foam production during operation. AGIs and midget impingers were each loaded with 10 mL, and Biosamplers were loaded with 20 mL. Impingers were weighed before and after sampling to assess evaporative losses and allow a more accurate estimation of the concentration of virus in each impinger post-sampling. For consistency across samplers, the gelatin filters and the stages of the BC251 sampler were also recovered using gMEM without FBS. The first two stages of the BC251 consist of a 15 mL conical tube and a 1.5 mL conical tube. Material collected on these stages was recovered by adding 5 mL and 1 mL of recovery media, respectively. The tubes were then capped, inverted, and vortexed for ten seconds to re-suspend collected material. The third stage of the BC251 was loaded with a 37-mm gelatin filter (Sartorius Stedim Biotech GmbH, PN:225-955112602-37-ALK). These filters, as well as the 25-mm gelatin filters used as independent samplers, were recovered in 10 mL of media unless otherwise specified. Filters were recovered by placing the filter into the recovery medium and warming briefly at 37 °C to dissolve the filter. For all sample types, FBS was added back to a final concentration of 10% after collection or sample recovery, but prior to plating for EBOV infectivity analysis.
Comparison of five low-flow aerosol samplers for collection of EBOV aerosols
A test system was constructed to facilitate simultaneous sampling of an aerosol by up to six low-flow aerosol samplers at humidity levels ranging from 15-75% RH (). The six sampling devices already described were attached around the circumference of the chamber. Aerosols were generated with an air-assist nozzle (PN: IAZA5200415K, The Lee Co.). This type of nozzle has been used for aerosol generation by our laboratory in other recent bioaerosol studies (Dabisch et al. Citation2020; Schuit et al. Citation2020) due to its small size, constant output, and ability to produce a wide range of particle sizes depending on the air pressure and liquid feed rate supplied to the device. In the present study, this nozzle was supplied with dry compressed air at 13-14 L/min and with suspensions of either 100-nm fluorescent Polystyrene Latex (PSL) microspheres (ThermoFisher Scientific, PNG0100B) in gMEM or EBOV/Mak in gMEM at 0.1 mL/min. PSL microspheres were used as a non-labile tracer to assess the physical efficiency of the sampling devices independent of their effect on viral infectivity, with fluorescence in samples measured using a Promega Glomax Multi Jr florescence reader. Aerosols were generated with target mass median aerodynamic diameters (MMADs) between 1-2 µm, as EBOV animal model challenge studies frequently report either aerosols in this range and/or use the Collison nebulizer, an aerosol generator known to produce aerosols in this range (Herbert et al. Citation2013; Johnson et al. Citation1995; Lever et al. Citation2012; Pratt et al. Citation2010; Reed et al. Citation2011; Smither et al. Citation2015; Twenhafel et al. Citation2013). For each test, aerosol was generated for one minute prior to initiation of sampling to allow the chamber concentration to reach a steady state. Following this equilibration period, flow to the aerosol samplers was engaged and the devices simultaneously sampled the generated aerosol for four minutes. Both virus and PSL-microsphere tests were conducted at room temperature and at target relative humidity levels of either 20% or 70% to assess the effect of relative humidity on the performance of the samplers. Temperature and relative humidity were measured with a Vaisala HM40 probe near the sampling chamber outlet and recorded at the mid-point of each test.
Figure 1. Depiction of the sampler characterization system. Relative humidity-conditioned air (a) was directed around the nozzle, which was supplied with dry compressed air (b) and liquid (c). A HEPA-filtered passive inlet (d) allowed for dissipation of any pressure buildup due to slight differences in the supply and exhaust airflows. The generated aerosol (e, in cut-away view) was carried through a 10.2 cm diameter stainless steel duct (f) before being directed by a 3.8 cm diameter 90-degree bend into the sampling chamber. Perforated stainless steel plates (g) at the top and bottom of the chamber facilitated even distribution of the air stream throughout the chamber volume. The aerosol was sampled at ports located around the circumference of the chamber (h, and H inset). Aerosol samplers were positioned as follows: (1) Gelatin filter, (2) AGI, (3) Midget impinger, (4) BC251, (5) Biosampler, and (6) APS with 1:20 diluter (H inset). A Vaisala HM40 probe (i) measured the temperature and relative humidity of the air stream before it was exhausted from the chamber through a HEPA filter (j).
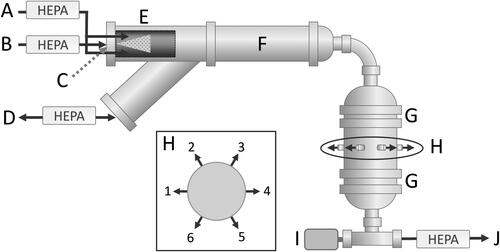
Evaluation of gelatin filter flow rate on EBOV aerosol concentration determinations
In a separate set of tests, the samplers were replaced with gelatin filters operated at the same flow rate as the samplers themselves. The filters in this configuration were used to collect aerosols of 100-nm PSL microspheres to assess the impact of differences in sampler airflow at each port on the measured aerosol concentration. Gelatin filters were selected for this purpose as they have been shown previously to collect with near 100% physical efficiency in the size range being generated (Burton, Grinshpun, and Reponen Citation2007). Tests were also conducted with aerosols containing EBOV/Mak and sampled with gelatin filters operating at a range of flows to determine if concentrations of EBOV/Mak measured with gelatin filters were affected by airflow, and, potentially, desiccation resulting from higher airflow rates.
Evaluation of gelatin filter recovery volume on EBOV assay results
The manufacturer-recommended liquid recovery volume for the 25-mm gelatin filters used in the present study is 10 mL. It is possible to dissolve the filters in smaller volumes and thereby obtain a more concentrated sample, which may be useful for detection and quantification of infectious virus in dilute aerosols. However, it was not known whether the resulting higher concentrations of gelatin would adversely affect either the virus or cells used for viral assays. To assess this, aliquots of virus in FB DMEM were prepared in volumes of 2.5, 5.0, and 10.0 mL, each containing 100 µl of a 1:1000 dilution of EBOV/Mak stock. A single gelatin filter was added to each tube, warmed briefly at 37 °C to dissolve, and the contents assayed by microtitration using the Vero-Ebola-reporter cells. Volume-matched negative controls with virus but without gelatin were also prepared and assayed. Assay plates were read by plate reader and fluorescence microscopy for evaluation of Zs-Green expression and by brightfield microscopy for virus-induced CPE and gelatin filter-induced cytotoxic effects (CTE). Nine replicate tests evenly distributed over 3 days were conducted with each sample type.
Comparison of two different aerosol sampler and virus assay combinations for measuring EBOV aerosol concentrations
In animal model inhalation challenge studies, EBOV aerosol concentrations are often quantified using an AGI for aerosol sampling and a plaque assay to quantify infectious virus in samples (Duy et al. Citation2016; Geisbert et al. Citation2008; Herbert et al. Citation2013; Johnson et al. Citation1995; Reed et al. Citation2011; Twenhafel et al. Citation2013). To determine whether improvements in sensitivity could be obtained using alternate methods, a series of tests were conducted to compare the AGI/plaque assay combination with an alternate method consisting of a gelatin filter recovered in 2.5 mL and assayed with a microtitration assay using the Vero-Ebola-reporter cells. These tests were conducted in the same test system used for the aerosol sampler comparison experiments but used only three of the six sampling ports. To assess system uniformity in this configuration, an APS and two gelatin filters operating at 6 L/min were positioned at sampling positions 1, 3, and 5 on the sampling chamber (). 100-nm PSL microspheres in FB DMEM were aerosolized into the chamber and sampled with gelatin filters in six replicate tests to determine if aerosol concentrations were biased by sampling port location. Following this uniformity assessment, a set of tests were conducted with EBOV/Mak aerosols. For these tests, dilutions of virus stock in 0.5-Log increments ranging from 10−2.5 to 10−5 were prepared in FB DMEM and used to generate aerosols in the test system. An APS, AGI, and gelatin filter were positioned at locations 1, 3, and 5 on the sampling chamber (). AGIs containing 10 mL of gMEM without FBS were operated at 6 L/min. Gelatin filters were operated at 6 to 8 L/min and were recovered in in 2.5 mL FB DMEM. Following each test, gelatin filter samples were assayed by microtitration on Vero-Ebola-reporter cells, and the contents of the AGI were assayed by plaque assay. To account for the difference between the units of the two virus assays so that results could be directly compared, microtitration results in units of TCID50 were converted to Infectious Units (IU) by multiplying TCID50 values by 0.69 to account for the difference between units (Bryan Citation1957). Each PFU was assumed to be equivalent to one IU. A limit of quantitation (LOQ) for each combination of sampler and assay was determined based on the nominal flow rate of the sampler and the smallest amount of virus detected by the assay that could be used to calculate a titer. For microtitrations, this was detection of one positive well in an assay plate. For plaque assays, two LOQ thresholds were used: detection of one plaque in three wells (the minimum needed to calculate a titer in PFU), and 10 plaques per well at the 100 dilution, the minimum threshold recommended for the FANG plaque assay.
Data analysis
For each test, the aerosol concentration of either virus or PSL microspheres measured by each sampler (Ca,measured) was calculated according to Equation (1). The aerosol concentration expected based on the output of the nozzle and system airflow (Ca,expected) was calculated according to Equation (2). For each sampler comparison test, the ratio of the measured aerosol concentration for each sampler to the expected aerosol concentration, representing the total efficiency of the test system (ηsys), was calculated according to Equation (3).
Equation (1). Calculation of Measured Aerosol Concentration (Ca,measured). Cs is the concentration of fluorescence or virus recovered in the sampler, Vs is the total recovery volume of the sampler, Qs is the sampler flow, and t is the sample duration.
Equation (2). Calculation of Expected Aerosol Concentration (Ca,expected). Cg is the concentration of fluorescence or virus in the suspension being aerosolized, qg is the liquid output rate of the air assist nozzle, Qsys is the system airflow.
Equation (3). Calculation of System Efficiency (ηsys). The system efficiency (ηsys) was calculated as the ratio of the measured aerosol concentration to the expected aerosol concentration, expressed as a percentage.
The relative physical efficiency (ηphys) for each sampler was calculated as the ratio of the system efficiency for a given sampler with the PSL microspheres to the system efficiency measured using a gelatin filter flowing at the same flow rate as the sampler with the PSL microspheres (Equation (4)). This parameter is a measure of the sampler’s ability to collect and retain aerosol particles in the size range generated, regardless of whether they contain any biological material. The relative biological efficiency of each sampler (ηbio), defined as the ability of each sampler to preserve the infectivity of collected virus, was expressed as the ratio of the system efficiency for a given sampler measured with EBOV to the mean system efficiency for that sampler measured with the PSL microspheres (Equation (5)). This term normalizes the amount of infectious virus collected by the sampler by its ability to collect and retain particles of equivalent size.
Equation (4). Calculation of Sampler Relative Physical Efficiency (ηMphys). Sampler relative physical efficiency was calculated as the system efficiency with 100-nm PSL microspheres measured with the samplers (ηsys,PSL), normalized for physical losses within the sampler by dividing by the system efficiency measured using gelatin filters operating at the same flow rate (ηsys,PSL(filter)).
Equation (5). Calculation of Sampler Biological Efficiency (ηbio). Biological sampler efficiency is calculated as the system efficiency measured for the virus (ηsys, Virus) normalized for physical losses by dividing by the system efficiency measured at the same conditions with 100-nm PSL microspheres as a physical tracer (ηsys, PSL).
Finally, the total efficiency for each sampler (ηtot), incorporating both physical and biological efficiencies, was calculated as the product of the biological efficiency and the mean physical efficiency value determined for that sampler (Equation (6)). Any infectivity losses reflected by ηbio and ηtot may be due to inactivation of the virus in the sampler, inactivation during the aerosol generation and droplet equilibration process, or both. Because the samplers were tested in parallel in the same system, any losses during aerosol generation and droplet equilibration represent a constant value across all samplers. However, these terms do not distinguish between the potential sources of viral inactivation, and, therefore, should be considered relative measurements.
Equation (6). Calculation of Sampler Total Efficiency (ηTot). The total efficiency is calculated as the product of the sampler’s physical and biological efficiencies, or the system efficiency measured for the virus (ηsys, Virus) normalized for both physical and biological losses within the sampler by dividing by the system efficiency measured using gelatin filters set to identical flow rates (ηsys,PSL(filter)).
Data from sampler comparison tests were analyzed by two-way ANOVA tests with relative humidity and either sampler type or flow rate as factors, with a Tukey’s multiple-comparisons post-test to determine the significance of specific comparisons. One-way ANOVA was used to compare virus titers in tests of the effect of different gelatin filter recovery volumes. A paired t-test was used to compare aerosol concentrations of PSL microspheres in experiments to determine system uniformity. Finally, linear regression analysis was used to determine the degree to which EBOV aerosol concentrations measured by the two different sampler/assay combinations correlated with the concentration of virus in the suspensions used to generate the aerosols. Specific comparisons between concentration data at the same dilution were made by t-tests. Because TCID50 data result from an assay based on a ten-fold dilution series and tend to be log-normally distributed (Wulff, Tzatzaris, and Young Citation2012), all statistical comparisons of virus titers and virus aerosol concentrations were performed on log10-transformed data. For consistency, PSL microsphere aerosol concentrations were also log10-transformed for statistical comparisons as well. All statistical analyses were performed using GraphPad Prism version 8.3.1.
Results
Environmental conditions
The mean temperature across all tests was 23.0 ± 1.1 °C. For tests with low and high relative humidity, the mean values were 17.2 ± 2.0% and 73.5 ± 7.0%, respectively. For tests comparing the AGI/plaque assay to the gelatin filter/microtitration assay, the mean relative humidity was 13.5 ± 2.5%.
Particle size distributions
The mean MMAD values measured with the APS for tests at low relative humidity with 100-nm PSL microspheres and EBOV/Mak were 1.68 ± 0.01 µm and 1.58 ± 0.06 µm, respectively. At high relative humidity, the mean MMAD values for tests with 100-nm PSL microspheres and EBOV/Mak were 1.92 ± 0.04 µm and 1.88 ± 0.07 µm, respectively. This increase in MMAD from low to high relative humidity was statistically significant (P < 0.001), but there were no significant differences in mean geometric standard deviations (GSD) between the different relative humidity levels and aerosol type when compared by two-way ANOVA. The mean GSD across all sampler comparison tests was 1.73 ± 0.15. For tests comparing the AGI/plaque assay to the gelatin filter/microtitration assay, the mean MMAD and GSD values were 1.63 ± 0.03 µm and 1.65 ± 0.02, respectively.
Comparison of low-flow aerosol samplers for collection of EBOV aerosols
Aerosol concentrations of 100-nm PSL microspheres measured with gelatin filters operating at a range of airflows equivalent to those of the other samplers are shown in . Concentrations were significantly affected by relative humidity, but not sampling port location or the interaction between relative humidity and sampling port (P < 0.001, P = 0.069, and P = 0.500, respectively). Aerosol concentrations of either 100-nm PSL microspheres or EBOV/Mak measured with each sampler are shown in and , expressed as either relative fluorescence units (RFU) per liter of air or TCID50 per liter of air, respectively. For 100-nm PSL microsphere aerosol concentrations, sampler type and the interaction between sampler type and RH were significant factors, but relative humidity alone was not significant (P < 0.001, P = 0.002, and P = 0.228, respectively). For EBOV/Mak aerosol concentrations, relative humidity, sampler type, and their interaction were all significant factors (P = 0.038, P < 0.001, and P = 0.025, respectively). The largest difference in mean EBOV/Mak concentrations was observed between the gelatin filters and midget impingers at low relative humidity, with a difference of 1.2 Log TCID50/L-air. The differences between PSL microsphere aerosol concentrations measured by these samplers was only 0.4 Log RFU/L-air.
Figure 2. EBOV/Mak and PSL-microsphere aerosol concentrations. Data from low relative humidity tests are shown in white, and data from high relative humidity tests are shown in gray. MI = midget impinger, BC251 = total collected across all stages of the NIOSH BC251, AGI = Model 7541 All Glass Impinger, BS = Biosampler, GF = 25 mm gelatin filter. Sampler flow rates, in L/min, are shown in parentheses below each sampler type. Data are presented as the mean ± standard deviation of 6-7 replicate tests. (a) Samples were collected using gelatin filters set to flow at rates matching those of the other samplers to normalize for physical collection efficiecy at each port and allow evaluation of the uniformity of aerosol within the chamber. Two separate ports had filters flowing at 1.0 L/min, as both the midget impinger and the gelatin filter were operated at that flow rate during subsequent sampler comparison tests. Aerosol concentrations of 100-nm PSL microspheres (b) and EBOV/Mak (c) measured with the various samplers were significantly affected by sampler type and the interaction between RH and sampler. However, RH as an independent factor was only significant for EBOV/Mak concentrations.
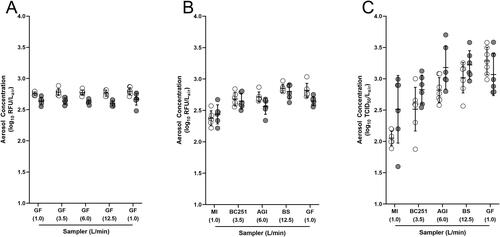
The physical, biological, and total efficiencies determined for the samplers tested in the present study are presented in . Sampler type was a significant factor for all efficiencies (P < 0.020 for all analyses), but relative humidity was only significant for biological and total efficiency (P = 0.007 and P = 0.004, respectively). There were no significant differences between the biological and total efficiencies of any of the samplers in tests at high relative humidity (P > 0.082 for all comparisons using Tukey’s multiple comparisons post-test). At low relative humidity, the biological efficiency of the gelatin filters for EBOV/Mak was 5.7 ± 3.3%, approximately twice as high as those of the Biosampler (2.7 ± 1.1%) and the AGI (2.2 ± 0.7%), four times higher than the BC251 (1.5 ± 1.3%), and seven times higher than the midget impinger (0.8 ± 0.3%). Comparisons between the total efficiency values of the samplers for low relative humidity tests followed the same pattern as the biological efficiency values. However, for both data sets, the only significant differences between samplers at low relative humidity were between the midget impinger and the AGI, Biosampler, and gelatin filter (P < 0.037 for all comparisons) and between the gelatin filter and the BC251 (P < 0.023 for both comparisons).
Figure 3. Sampler Efficiencies. Data from tests at low relative humidity are shown in white, and data from tests at high relative humidity are shown in gray. MI = midget impinger, BC251 = total collected across all stages of the NIOSH BC251, AGI = Model 7541 All Glass Impinger, BS = Biosampler, GF = 25 mm gelatin filter. Data are presented as the mean ± standard deviation of 6-7 replicate tests. Relative physical efficiency values measured for all samplers are shown in (a), relative biological efficiency values are shown in (b), and total efficiency values are shown in (c).
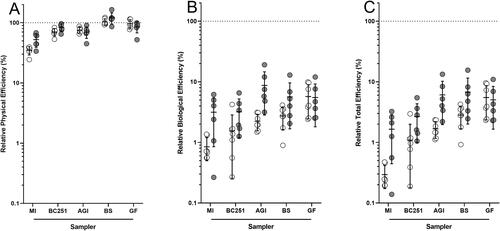
Evaluation of gelatin filter flow rate on EBOV aerosol concentration determinations
The results of tests using gelatin filters operating at different flow rates to sample EBOV/Mak aerosols are presented in , with the data presented as both the total virus in each sample () and the resulting aerosol concentrations, which factor in the amount of air sampled by the filter (). The total amount of virus in a sample was significantly affected by the airflow rate of the filter (P < 0.001), but neither relative humidity nor the interaction between relative humidity and flow rate were significant factors (P = 0.468 and P = 0.199). Since relative humidity was not a significant factor, data at the different humidity levels were pooled and a Tukey’s multiple comparisons post-test used to assess the effect of flow rate on virus concentrations. Viral titers in the samples () increased by a significant amount as airflow rate increased from 1 L/min to 6 L/min, but there was no significant increase in virus titer between 6 L/min and 12.5 L/min. When these titers were normalized for sampler airflow by calculating aerosol concentrations of infectious virus (), the aerosol concentrations from 12.5 L/min filters were lower than those of 6.0 L/min filters, but this difference did not reach statistical significance (P = 0.079). Neither relative humidity nor airflow rate significantly affected EBOV aerosol concentrations across the range of airflows tested.
Figure 4. EBOV/Mak in samples from gelatin filters with varied airflow rates. Data from low and high RH tests are shown in white and gray, respectively. The virus titers for each sample set are shown in (a), and the airflow normalized aerosol concentrations are shown in (b). Data are presented as the mean ± standard deviation of 4 replicate tests.
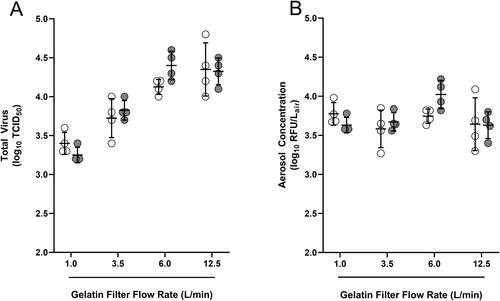
Evaluation of gelatin filter recovery volume on EBOV assay results
In tests examining whether gelatin filters could be recovered in volumes less than the manufacturer-recommended 10 mL, significant CTE was observed under brightfield microscopy in all undiluted assay wells for gelatin filters dissolved in 2.5 mL of FB DMEM. This CTE was observed in negative control wells containing dissolved gelatin filters but no virus, and was indistinguishable from viral CPE in sample wells that contained virus. Mild CTE, distinguishable from CPE, was observed in undiluted wells for gelatin filters dissolved in 5.0 mL. However, both manual observation by fluorescent microscopy and plate reader analysis of Zs-Green fluorescence enabled identification of infected wells for all recovery volumes, despite the confounding effects of CTE on CPE determinations by brightfield microscopy. By one-way ANOVA, there were no differences in the total amount of virus present between samples with different gelatin filter recovery volumes (P = 0.684 and P = 0.823 for automated and manual reads, respectively), indicating that the tested gelatin filter concentrations did not inhibit viral infection or Zs-Green expression.
Comparison of two different aerosol sampler and virus assay combinations for measuring EBOV aerosol concentrations
In tests to determine system uniformity when only an APS and two samplers were sampling from the chamber, 100-nm PSL microsphere concentrations measured at each port were not significantly different from each other by paired t-test (P = 0.732). This indicated that the aerosol concentration was uniform within the chamber, and that any differences in concentration measured by samplers placed at those ports would be a function of either physical or biological losses within the sampler, unbiased by the position of the sampler within the test system.
Results from subsequent tests in which EBOV/Mak aerosols were quantified by two different sampling and assay combinations are shown in . The gelatin filter/microtitration combination was able to detect and quantify infectious virus in all but one test, and there was a strong correlation between measured aerosol concentrations and the titer of the virus suspensions used for aerosol generation across all tested dilutions. Linear regression analysis of these data returned a slope of 0.97 with an R2 of 0.98. The AGI/plaque assay combination was able to detect infectious virus in some tests at all dilutions of input virus stock. However, in four tests, it failed to detect any infectious virus at all, and the mean aerosol concentrations for input dilutions of 10−3.5 to 10−5 differed by only 0.1 log IU/L-air despite the 1.5 log difference in input virus concentrations. Linear regression analysis of aerosol concentrations determined by the AGI/plaque assay combination across all input dilutions returned a slope of 0.55 with an R2=0.75. This relationship was improved by restricting analysis to input concentrations of 10−3.5 to 10−2.5, where there appeared to be a linear response of measured aerosol concentrations to input titer. For these three dilutions, there was a corresponding linear regression slope of 1.3 with an R2=0.92.
Figure 5. EBOV/Mak aerosol concentrations determined by two sampling and assay pathways. EBOV/Mak was aerosolized from virus stock dilutions ranging from 10−2.5 to 10−5. Viral concentrations are presented in infectious unit equivalents to facilitate comparison between different assays. (A) Aerosol concentrations of EBOV/Mak determined with gelatin filters flowing at 6-8 L/min, recovered in 2.5 mL FB DMEM, and assayed by microtitration on Vero-Ebola-reporter cells. The dotted line indicates the LOQ, calculated using one positive well in the undiluted assay row. (B) Aerosol concentrations of EBOV/Mak determined using Model 7541 AGIs and a plaque assay. The dotted line designated “High LOQ” was determined using ten plaques per well in the undiluted assay wells, and the dotted line designated “Low LOQ” was determined using one plaque per three wells in the undiluted assay wells. Overall, the gelatin filter/microtitration combination was more sensitive and had a wider range over which it had a linear relationship with the titers of the diluted stock material than the AGI/plaque assay combination.
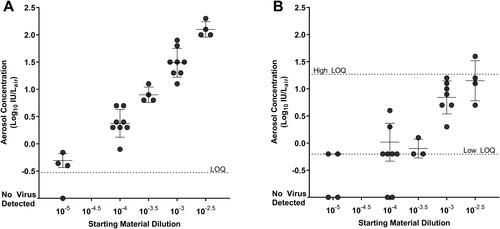
Across all dilutions of the virus stock used for aerosol generation, the aerosol concentrations measured by the gelatin filter/microtitration assay combination were on average 0.6 log IU/L-air greater than those measured by the AGI/plaque assay combination. However, this difference was 0.9 log IU/L-air when comparing only across input dilutions of 10−2.5 to 10−3.5, where there was a better correlation between input titer and the aerosol concentration measured by the AGI/plaque assay combination. Notably, for all but one of 28 tests, EBOV aerosol concentrations determined with the AGI/plaque assay pathway had to be calculated based on fewer than ten plaques counts per well, the limit recommended for the FANG plaque assay (Shurtleff et al. Citation2012), as even in undiluted assay wells there were insufficient plaques to meet this threshold.
Discussion
The efficiency with which an aerosol sampler collects and preserves the viability or infectivity of a microorganism or virus has the potential to bias aerosol concentration data and complicate comparisons between studies in which different samplers are used. Data comparing the performance of different sampling devices can be used to inform sampler selection in future studies and serve as a bridge between studies that utilize different methods. To-date, no such data have been reported for filoviruses, including EBOV. Therefore, the present study was conducted to evaluate the relative performance of five commonly used aerosol sampling devices for sampling aerosols containing EBOV. The results demonstrate that sampler performance with EBOV/Mak was affected by relative humidity, with high relative humidity (mean value: 73.5 ± 7.0%) tending to result in higher total efficiency values. Furthermore, at high relative humidity, all of the samplers had statistically indistinguishable total efficiencies for EBOV/Mak, suggesting that any of the tested samplers for EBOV/Mak could be used to estimate EBOV/Mak concentrations with equivalent sensitivity under similar conditions. Differences in sampler performance were identified in tests at low relative humidity, with the AGI, Biosampler, and 25-mm gelatin filters performing significantly better than the midget impinger and BC251. Unlike the AGI, Biosampler, or midget impinger, gelatin filters do not contain any glass components, making them a safer choice than a glass impinger in biocontainment applications. Additionally, gelatin filters can be operated across a range of airflow rates and can be installed in any orientation, making them a versatile choice for a variety of sampling applications. Finally, gelatin filters can be recovered in small liquid volumes, leading to a more concentrated aerosol sample and an increased likelihood of detecting infectious virus in dilute air samples. Based on these factors, gelatin filters were identified as a preferred sampling device for EBOV aerosols and used for direct comparison tests against traditional EBOV sampling and assay methods. Combining gelatin filters and a microtitration assay using a fluorescent reporter cell line for sampling and quantifying EBOV/Mak resulted in an improvement in sensitivity of nearly ten-fold relative to the more common AGI and plaque assay methodology used to quantify EBOV in aerosols in previous studies. While the gelatin filter was identified as a preferred sampler in the present study, it should also be noted that other samplers may still be preferable for certain applications, such as size-fractionated collection by the BC251 in clinical or field sampling settings.
All sampling data in the present study were generated from tests of a single duration, and it is possible that different sampling durations could produce different results. For example, longer test durations could result in desiccation of the virus on the surface of the filter. In the present study, virus concentration increased with higher airflow rates from 1 to 6 L/min but not between 6 and 12.5 L/min, which suggests that desiccation of EBOV/Mak may be occurring at the higher flow rates. Additionally, longer operating durations may have the potential to cause gelatin filters to become brittle, making recovery difficult and potentially causing cracks during sampling that could lower collection efficiency (Burton et al. Citation2005).
Longer test durations could also affect results from the liquid impingers, either by longer exposure to mechanical agitation or osmotic shifts in the collection media as liquid evaporates. Increasing the volume of the impinger collection liquid may reduce the potential for this to occur, and potentially also improve collection efficiency. However, increasing the liquid volume would result in a lower virus concertation in the final liquid sample, which may be inconsequential for high concentration samples but which may be problematic for low concentration samples unless the entire volume of the sample is assayed. It should also be noted that AGIs are available in multiple configurations, with different stem heights and operating airflow rates. Further tests would be necessary to determine the degree to which these factors could affect EBOV aerosol concentrations determined with AGIs or other impingers. Other factors not tested in the present study, but that have the potential to affect sampler results, include the particle size of the test aerosols, the composition of the collection medium in impingers, and the composition of the liquid suspensions used for aerosol generation. Additional tests incorporating an evaluation of these factors would be useful to better understand the parameters that affect sampler performance for EBOV aerosols generally.
Tests with gelatin filters operating at sampler flow rates to collect PSL microsphere aerosols demonstrated that the six-port sampler characterization system delivered uniform concentrations of aerosol particles to all sampling ports, and that the different airflows at each sampling port did not bias the concentrations of aerosol within the chamber. PSL microsphere concentrations measured both with the gelatin filters operating at sampler flow rates and with the samplers themselves tended to be higher at low relative humidity than at high relative humidity. The mean MMAD of aerosols in low RH tests was smaller by approximately 0.2 µm than the mean MMAD in high RH tests, likely a result of the particles retaining more moisture in higher RH conditions. It is possible that the humidity-dependent difference in aerosol concentration may result from this difference in particle size, as larger particles are more likely to be lost to bends and settle faster than smaller particles (Hinds Citation1999).
Although relative humidity affected the physical transmission of particles through the test system, it did not significantly affect the relative physical efficiency of the samplers themselves. However, relative humidity did significantly affect sampler biological efficiencies, with samplers tending to preserve infectivity better at high relative humidity by an average of 2.6-fold. Humidity-dependent differences in sampler comparisons results have been reported for other viruses, although in contrast to the results of the present study, lower humidity is generally found to better for enveloped viruses than higher humidity (Kim et al. Citation2007; Liu et al. Citation2012; Tseng and Li Citation2005). However, some enveloped viruses have displayed high stability at both low and high relative humidity, with a stability minimum at mid-range relative humidity (Schaffer, Soergel, and Straube Citation1976; Yang, Elankumaran, and Marr Citation2012). The sensitivity of viruses to relative humidity differences in aerosols is thought to be dependent on the composition of the surrounding particle, its hydration state, and the rate at which particle rehydration occurs (Benbough Citation1971; Marr et al. Citation2019; Yang and Marr Citation2012). In the present study, EBOV aerosols for both low and high relative humidity tests were generated from the same suspension of virus in cell culture medium. However, the humidity-dependent difference in particle size observed in the present study suggests that particles were more hydrated at high relative humidity than at low relative humidity, and it is possible that this more hydrated state enhanced preservation of EBOV infectivity during sampling. This observation is consistent with data from a previous study which found that EBOV/Mak retained infectivity in surface-deposited droplets for longer periods of time in high relative humidity compared with low relative humidity conditions (Schuit et al. Citation2016). Additional tests incorporating more target relative humidity values and an evaluation of the effect of particle composition would be useful to better understand the effect of humidity on aerosol sampler performance with EBOV. Such data would also provide insight into the relative performance of different samplers for a wider range of laboratory and clinical applications, including those with relative humidity levels different from those tested in the present study.
Despite performing better at high relative humidity, total efficiency values across all samplers were low, with no mean value higher than seven percent. As mentioned previously, infectivity losses leading to relative biological and total efficiency values less than 100% may be due either to processes occurring in the samplers themselves or to inactivation in the aerosol generation or equilibration process. A relative biological efficiency of 100% for a given sampler would imply that all of the virus had survived the aerosol generation, equilibration, and sampling processes. In that case, the biological and total efficiencies of the other samplers tested in the same system could be considered absolute, but in the present study no sampler had a relative biological efficiency of 100%. Therefore, while the resulting biological and total efficiencies for EBOV in the present study are useful for making comparisons between the tested samplers, they should be considered relative measurements.
The combination of 25-mm gelatin filters recovered in 2.5 mL of media and a microtitration assay using Vero-Ebola-Reporter cells was able to detect and quantify aerosol concentrations of infectious EBOV/Mak with nearly ten-fold better sensitivity than the combination of the AGI and the FANG plaque assay. These results demonstrate the dependence of aerosol concentration on both the sampling device and assay methodology utilized, and suggest that studies that use an AGI and plaque assay to quantify EBOV in aerosols may underestimate the amount of infectious virus present in experimental aerosols. Furthermore, the results of the present study underscore the importance of characterizing the methods used in bioaerosol studies for determining concentrations of active microorganisms or viruses in aerosols.
Sampler efficiency, assay sensitivity, and the interplay between these and other components of the aerosol quantification process can have profound implications for the conclusions of a study or comparisons between studies. Therefore, bioaerosol studies may benefit from including a characterization phase early in their execution to evaluate the performance of the sampling and assay methods that are to be used. The approach taken in the present study for characterizing aerosol samplers with EBOV may be useful for such studies with other viruses or microorganisms. However, any such extensions of this approach may need to account for other variables that were not applicable in the present study, including bacterial growth phase, spore germination state, or the presence of viable but not culturable (VBNC) organisms.
While the AGI/plaque assay combination was able to detect infectious virus in some tests at the most dilute concentration tested, the concentrations measured in those tests were not significantly different than the concentrations measured in tests with aerosols that were 1.5 log less concentrated. This is most likely due to the inclusion of plaque assay data with no lower bound on the number of countable plaques, with the resulting data being more reflective of random chance than a true indication of the amount of virus in the sampler. Setting a higher threshold for plaque counts would likely result in more reliable data, but the tradeoff for doing so would be a higher LOQ. This would have the potential to increase the number of circumstances in which it is possible to detect but not quantitate infectious virus. For example, in the present study there were enough plaques to meet the threshold of 10 plaques per well recommended for the FANG plaque assay (Shurtleff et al. Citation2016) in only one out of the 28 tests that were conducted. While aerosol concentrations could be calculated using these plaque counts, the resulting values did not correlate well with the titer of the virus stock used to generate the aerosols, suggesting that the aerosol concentrations determined by this method were not representative of the true concentration of infectious EBOV present in the aerosols in those tests. This problem could potentially be overcome by plating a greater volume of the sample. However, this approach would present an additional logistical burden, especially in studies requiring the processing of multiple samples per day for extended durations.
Aerosol sampler comparison data from the present study will be useful to inform sampler selection and system design for future studies, and to facilitate comparisons between EBOV aerosol studies that utilize different sampling devices. The increased sensitivity of the gelatin filter paired with the Vero-Ebola-Reporter microtitration assay could be useful for applications where quantitation of very low concentrations of infectious EBOV is desired, including studies examining the inhalational infectivity and/or lethality of low concentrations of aerosolized EBOV in animal models of disease, detection or high-confidence non-detection of infective virus in the air surrounding infected patients and/or animals, and assessment of the decay of viral infectivity in aerosols. Such studies would provide empirical data to corroborate the epidemiology observed in natural outbreak settings and its transmission potential both within and outside the endemic region, and would also be useful for biodefense hazard assessment and response preparedness planning.
Additional information
Funding
References
- Alibek, K. 2008. Biohazard. New York: Random House.
- Alsved, M., L. Bourouiba, C. Duchaine, J. Löndahl, L. C. Marr, S. T. Parker, A. J. Prussin, and R. J. Thomas. 2020. Natural sources and experimental generation of bioaerosols: Challenges and perspectives. Aerosol Sci. Technol. 54 (5):547–71. doi:https://doi.org/10.1080/02786826.2019.1682509.
- Alves, D., A. Glynn, K. Steele, M. Lackemeyer, N. Garza, J. Buck, C. Mech, and D. Reed. 2010. Aerosol exposure to the angola strain of marburg virus causes lethal viral hemorrhagic fever in cynomolgus macaques. Vet. Pathol. 47 (5):831–51. doi:https://doi.org/10.1177/0300985810378597.
- Belanov, E., V. Muntianov, V. Kriuk, A. Sokolov, N. Bormotov, O. P'iankov, and A. Sergeev. 1996. Survival of marburg virus infectivity on contaminated surfaces and in aerosols. Vopr. Virusol. 41 (1):32–4.
- Benbough, J. 1971. Some factors affecting the survival of airborne viruses. J. Gen. Virol. 10 (3):209–20. doi:https://doi.org/10.1099/0022-1317-10-3-209.
- Bertran, K., C. Balzli, Y.-K. Kwon, T. M. Tumpey, A. Clark, and D. E. Swayne. 2017. Airborne transmission of highly pathogenic influenza virus during processing of infected poultry. Emerg. Infect. Dis. 23 (11):1806–14. doi:https://doi.org/10.3201/eid2311.170672.
- Borio, L., T. Inglesby, C. J. Peters, A. L. Schmaljohn, J. M. Hughes, P. B. Jahrling, T. Ksiazek, K. M. Johnson, A. Meyerhoff, T. O'Toole, et al. 2002. Hemorrhagic fever viruses as biological weapons: Medical and public health management. Jama 287 (18):2391–405., doi:https://doi.org/10.1001/jama.287.18.2391.
- Bryan, W. R. 1957. Interpretation of host response in quantitative studies on animal viruses. Ann .N Y Acad .Sci. 69 (4):698–728. doi:https://doi.org/10.1111/j.1749-6632.1957.tb49710.x.
- Burton, N. C., A. Adhikari, S. A. Grinshpun, R. Hornung, and T. Reponen. 2005. The effect of filter material on bioaerosol collection of bacillus subtilis spores used as a bacillus anthracis simulant. J. Environ. Monit. 7 (5):475–80. doi:https://doi.org/10.1039/b500056d.
- Burton, N. C., S. A. Grinshpun, and T. Reponen. 2007. Physical collection efficiency of filter materials for bacteria and viruses. The Annals of Occupational Hygiene 51:143–51.
- Cenciarelli, O., V. Gabbarini, S. Pietropaoli, A. Malizia, A. Tamburrini, G. M. Ludovici, M. Carestia, D. Di Giovanni, A. Sassolini, L. Palombi, et al. 2015. Viral bioterrorism: Learning the lesson of ebola virus in west africa 2013-2015. Virus Res. 210:318–26. doi:https://doi.org/10.1016/j.virusres.2015.09.002.
- Chowell, G., and H. Nishiura. 2014. Transmission dynamics and control of ebola virus disease (evd): A review. BMC Med. 12:196 doi:https://doi.org/10.1186/s12916-014-0196-0.
- Dabisch, P., M. Schuit, A. Herzog, K. Beck, S. Wood, M. Krause, D. Miller, W. Weaver, D. Freeburger, and I. Hooper. 2020. The influence of temperature, humidity, and simulated sunlight on the infectivity of sars-cov-2 in aerosols. Aerosol Sci. Technol. 1–15. doi:https://doi.org/10.1080/02786826.2020.1829536.
- Davies, A., G. Thomson, J. Walker, and A. Bennett. 2009. A review of the risks and disease transmission associated with aerosol generating medical procedures. J. Infect. Prev. 10 (4):122–6. doi:https://doi.org/10.1177/1757177409106456.
- Duy, J., J. W. Koehler, A. N. Honko, R. J. Schoepp, N. Wauquier, J.-P. Gonzalez, M. L. Pitt, E. M. Mucker, J. C. Johnson, A. O'Hearn, et al. 2016. Circulating microrna profiles of ebola virus infection. Sci. Rep. 6:24496– 13. doi:https://doi.org/10.1038/srep24496.
- Dybwad, M., G. Skogan, and J. M. Blatny. 2014. Comparative testing and evaluation of nine different air samplers: End-to-end sampling efficiencies as specific performance measurements for bioaerosol applications. Aerosol Sci. Technol. 48 (3):282–95. doi:https://doi.org/10.1080/02786826.2013.871501.
- Fabian, P., J. J. McDevitt, E. A. Houseman, and D. K. Milton. 2009. Airborne influenza virus detection with four aerosol samplers using molecular and infectivity assays: Considerations for a new infectious virus aerosol sampler. Indoor Air. 19 (5):433–41. doi:https://doi.org/10.1111/j.1600-0668.2009.00609.x.
- Finney, D. 1964. The spearman-karber method. Statistical methods in biological assay. 524–30.
- Fischer, R. J., T. Bushmaker, S. Judson, and V. J. Munster. 2016. Comparison of the aerosol stability of 2 strains of zaire ebolavirus from the 1976 and 2013 outbreaks. J. Infect. Dis. 214 (suppl 3):S290–S293. doi:https://doi.org/10.1093/infdis/jiw193.
- Geisbert, T. W., K. M. Daddario-Dicaprio, J. B. Geisbert, D. S. Reed, F. Feldmann, A. Grolla, U. Ströher, E. A. Fritz, L. E. Hensley, S. M. Jones, et al. 2008. Vesicular stomatitis virus-based vaccines protect nonhuman primates against aerosol challenge with ebola and marburg viruses. Vaccine 26 (52):6894–900., doi:https://doi.org/10.1016/j.vaccine.2008.09.082.
- Harbourt, D. E., S. C. Johnston, J. Pettitt, T. K. Warren, and W. R. Dorman. 2017. Detection of ebola virus rna through aerosol sampling of animal biosafety level 4 rooms housing challenged nonhuman primates. J. Infect. Dis. 215 (4):554–8. doi:https://doi.org/10.1093/infdis/jiw610.
- Henningson, E. W., and M. S. Ahlberg. 1994. Evaluation of microbiological aerosol samplers: A review. J. Aerosol Sci. 25 (8):1459–92. doi:https://doi.org/10.1016/0021-8502(94)90219-4.
- Herbert, A. S., A. I. Kuehne, J. F. Barth, R. A. Ortiz, D. K. Nichols, S. E. Zak, S. W. Stonier, M. A. Muhammad, R. R. Bakken, L. I. Prugar, et al. 2013. Venezuelan equine encephalitis virus replicon particle vaccine protects nonhuman primates from intramuscular and aerosol challenge with ebolavirus. J. Virol. 87 (9):4952–64., doi:https://doi.org/10.1128/JVI.03361-12.
- Hinds, W. C. 1999. Aerosol technology: Properties, behavior, and measurement of airborne particles. New York: John Wiley & Sons.
- Jaax, N., P. Jahrling, T. Geisbert, J. Geisbert, K. Steele, K. McKee, D. Nagley, E. Johnson, G. Jaax, and C. Peters. 1995. Transmission of ebola virus (zaire strain) to uninfected control monkeys in a biocontainment laboratory. The Lancet 346 (8991-8992):1669–71. doi:https://doi.org/10.1016/S0140-6736(95)92841-3.
- Johnson, D., R. Lynch, C. Marshall, K. Mead, and D. Hirst. 2013. Aerosol generation by modern flush toilets. Aerosol Sci. Technol. 47 (9):1047–57. doi:https://doi.org/10.1080/02786826.2013.814911.
- Johnson, E., N. Jaax, J. White, and P. Jahrling. 1995. Lethal experimental infections of rhesus monkeys by aerosolized ebola virus. Int. J. Exp. Pathol. 76 (4):227–36.
- Judson, S. D., and V. J. Munster. 2019. Nosocomial transmission of emerging viruses via aerosol-generating medical procedures. Viruses 11 (10):940. doi:https://doi.org/10.3390/v11100940.
- Kesavan, J., D. Schepers, and A. R. McFarland. 2010. Sampling and retention efficiencies of batch-type liquid-based bioaerosol samplers. Aerosol Sci. Technol. 44 (10):817–29. doi:https://doi.org/10.1080/02786826.2010.497513.
- Kim, S. W., M. Ramakrishnan, P. C. Raynor, and S. M. Goyal. 2007. Effects of humidity and other factors on the generation and sampling of a coronavirus aerosol. Aerobiologia. (Bologna) 23 (4):239–48. doi:https://doi.org/10.1007/s10453-007-9068-9.
- Lever, M. S., T. J. Piercy, J. A. Steward, L. Eastaugh, S. J. Smither, C. Taylor, F. J. Salguero, and R. J. Phillpotts. 2012. Lethality and pathogenesis of airborne infection with filoviruses in a129 α/β−/− interferon receptor-deficient mice. J. Med. Microbiol. 61 (1):8–15. doi:https://doi.org/10.1099/jmm.0.036210-0.
- Lin, X., T. Reponen, K. Willeke, Z. Wang, S. A. Grinshpun, and M. Trunov. 2000. Survival of airborne microorganisms during swirling aerosol collection. Aerosol Sci. Technol. 32 (3):184–96. doi:https://doi.org/10.1080/027868200303722.
- Lindsley, W. G., F. M. Blachere, R. E. Thewlis, A. Vishnu, K. A. Davis, G. Cao, J. E. Palmer, K. E. Clark, M. A. Fisher, R. Khakoo, et al. 2010. Measurements of airborne influenza virus in aerosol particles from human coughs. PloS One 5 (11):e15100., doi:https://doi.org/10.1371/journal.pone.0015100.
- Liu, K., Z. Wen, N. Li, W. Yang, J. Wang, L. Hu, X. Dong, J. Lu, and J. Li. 2012. Impact of relative humidity and collection media on mycobacteriophage d29 aerosol. Appl. Environ. Microbiol. 78 (5):1466–72. doi:https://doi.org/10.1128/AEM.06610-11.
- Marr, L. C., J. W. Tang, J. Van Mullekom, and S. S. Lakdawala. 2019. Mechanistic insights into the effect of humidity on airborne influenza virus survival, transmission and incidence. J. R Soc. Interface 16 (150):20180298 doi:https://doi.org/10.1098/rsif.2018.0298.
- Mate, S. E., J. R. Kugelman, T. G. Nyenswah, J. T. Ladner, M. R. Wiley, T. Cordier-Lassalle, A. Christie, G. P. Schroth, S. M. Gross, G. J. Davies-Wayne, et al. 2015. Molecular evidence of sexual transmission of ebola virus. N. Engl. J. Med. 373 (25):2448–54., doi:https://doi.org/10.1056/NEJMoa1509773.
- May, K., and G. Harper. 1957. The efficiency of various liquid impinger samplers in bacterial aerosols. Br. J. Ind. Med. 14 (4):287–97. doi:https://doi.org/10.1136/oem.14.4.287.
- Piercy, T., S. Smither, J. Steward, L. Eastaugh, and M. Lever. 2010. The survival of filoviruses in liquids, on solid substrates and in a dynamic aerosol. J. Appl. Microbiol. 109 (5):1531–9. doi:https://doi.org/10.1111/j.1365-2672.2010.04778.x.
- Pratt, W. D., D. Wang, D. K. Nichols, M. Luo, J. Woraratanadharm, J. M. Dye, D. H. Holman, and J. Y. Dong. 2010. Protection of nonhuman primates against two species of ebola virus infection with a single complex adenovirus vector. Clin Vaccine Immunol . 17 (4):572–81. doi:https://doi.org/10.1128/CVI.00467-09.
- Reed, D. S., M. G. Lackemeyer, N. L. Garza, L. J. Sullivan, and D. K. Nichols. 2011. Aerosol exposure to zaire ebolavirus in three nonhuman primate species: Differences in disease course and clinical pathology. Microbes Infect. 13 (11):930–6. doi:https://doi.org/10.1016/j.micinf.2011.05.002.
- Roels, T. H., A. S. Bloom, J. Buffington, G. L. Muhungu, W. R. Mac Kenzie, A. S. Khan, R. Ndambi, D. L. Noah, H. R. Rolka, C. J. Peters, et al. 1999. Ebola hemorrhagic fever, kikwit, democratic republic of the congo, 1995: Risk factors for patients without a reported exposure. J. Infect. Dis. 179 (s1):S92–S97. doi:https://doi.org/10.1086/514286.
- Schaffer, F., M. Soergel, and D. Straube. 1976. Survival of airborne influenza virus: Effects of propagating host, relative humidity, and composition of spray fluids. Arch. Virol. 51 (4):263–73. doi:https://doi.org/10.1007/BF01317930.
- Schuit, M., D. M. Miller, M. S. Reddick-Elick, C. B. Wlazlowski, C. M. Filone, A. Herzog, L. A. Colf, V. Wahl-Jensen, M. Hevey, and J. W. Noah. 2016. Differences in the comparative stability of ebola virus makona-c05 and yambuku-mayinga in blood. PLoS One. 11 (2):e0148476 doi:https://doi.org/10.1371/journal.pone.0148476.
- Schuit, M., S. Ratnesar-Shumate, J. Yolitz, G. Williams, W. Weaver, B. Green, D. Miller, M. Krause, K. Beck, S. Wood, et al. 2020. Airborne sars-cov-2 is rapidly inactivated by simulated sunlight. J. Infect. Dis. 222 (4):564–71. doi:https://doi.org/10.1093/infdis/jiaa334.
- Shurtleff, A. C., J. E. Biggins, A. E. Keeney, E. E. Zumbrun, H. A. Bloomfield, A. Kuehne, J. L. Audet, K. J. Alfson, A. Griffiths, G. G. Olinger, et al. 2012. Standardization of the filovirus plaque assay for use in preclinical studies. Viruses 4 (12):3511–30. doi:https://doi.org/10.3390/v4123511.
- Shurtleff, A., H. Bloomfield, S. Mort, S. Orr, B. Audet, T. Whitaker, M. Richards, and S. Bavari. 2016. Validation of the filovirus plaque assay for use in preclinical studies. Viruses 8 (4):113 doi:https://doi.org/10.3390/v8040113.
- Smither, S., T. Piercy, L. Eastaugh, J. Steward, and M. Lever. 2011. An alternative method of measuring aerosol survival using spiders' webs and its use for the filoviruses. J. Virol. Methods. 177 (1):123–7. doi:https://doi.org/10.1016/j.jviromet.2011.06.021.
- Smither, S. J., M. Nelson, L. Eastaugh, A. Nunez, F. J. Salguero, and M. S. Lever. 2015. Experimental respiratory infection of marmosets (callithrix jacchus) with ebola virus kikwit. J. Infect. Dis. 212 (suppl 2):S336–S345. doi:https://doi.org/10.1093/infdis/jiv371.
- Terzieva, S., J. Donnelly, V. Ulevicius, S. A. Grinshpun, K. Willeke, G. N. Stelma, and K. P. Brenner. 1996. Comparison of methods for detection and enumeration of airborne microorganisms collected by liquid impingement. Appl. Environ. Microbiol. 62 (7):2264–72. doi:https://doi.org/10.1128/AEM.62.7.2264-2272.1996.
- Tseng, C.-C., and C.-S. Li. 2005. Collection efficiencies of aerosol samplers for virus-containing aerosols. J Aerosol Sci . 36 (5):593–607. doi:https://doi.org/10.1016/j.jaerosci.2004.12.004.
- Twenhafel, N. A., M. E. Mattix, J. C. Johnson, C. G. Robinson, W. D. Pratt, K. A. Cashman, V. Wahl-Jensen, C. Terry, G. G. Olinger, L. E. Hensley, et al. 2013. Pathology of experimental aerosol zaire ebolavirus infection in rhesus macaques. Vet. Pathol. 50 (3):514–29. doi:https://doi.org/10.1177/0300985812469636.
- Twenhafel, N., C. Shaia, T. Bunton, J. Shamblin, S. Wollen, L. Pitt, D. Sizemore, M. Ogg, and S. Johnston. 2015. Experimental aerosolized guinea pig-adapted Zaire ebolavirus (variant: Mayinga) causes lethal pneumonia in guinea pigs. Vet. Pathol. 52 (1):21–5. doi:https://doi.org/10.1177/0300985814535612.
- Verreault, D., L. Gendron, G. M. Rousseau, M. Veillette, D. Massé, W. G. Lindsley, S. Moineau, and C. Duchaine. 2011. Detection of airborne lactococcal bacteriophages in cheese manufacturing plants. Appl. Environ. Microbiol. 77 (2):491–7. doi:https://doi.org/10.1128/AEM.01391-10.
- Verreault, D., S. Moineau, and C. Duchaine. 2008. Methods for sampling of airborne viruses. Microbiol. Mol. Biol. Rev. 72 (3):413–44. doi:https://doi.org/10.1128/MMBR.00002-08.
- Weingartl, H. M., C. Embury-Hyatt, C. Nfon, A. Leung, G. Smith, and G. Kobinger. 2012. Transmission of ebola virus from pigs to non-human primates. Sci. Rep. 2 (1):811. doi:https://doi.org/10.1038/srep00811.
- Wulff, N. H., M. Tzatzaris, and P. J. Young. 2012. Monte carlo simulation of the spearman-kaerber tcid50. J. Clin. Bioinforma. 2 (1):5 doi:https://doi.org/10.1186/2043-9113-2-5.
- Yang, W., S. Elankumaran, and L. C. Marr. 2012. Relationship between humidity and influenza a viability in droplets and implications for influenza’s seasonality. PloS One 7 (10):e46789. doi:https://doi.org/10.1371/journal.pone.0046789.
- Yang, W., and L. C. Marr. 2012. Mechanisms by which ambient humidity may affect viruses in aerosols. Appl. Environ. Microbiol. 78 (19):6781–8. doi:https://doi.org/10.1128/AEM.01658-12.
- Zuo, Z.,. T. H. Kuehn, H. Verma, S. Kumar, S. M. Goyal, J. Appert, P. C. Raynor, S. Ge, and D. Y. Pui. 2013. Association of airborne virus infectivity and survivability with its carrier particle size. Aerosol Sci. Technol. 47 (4):373–82. doi:https://doi.org/10.1080/02786826.2012.754841.