Abstract
To realize the environmental impacts of mineral dust from different sources, it is necessary to develop aerosol generation systems that can mimic the processes of aerosolization of sediments into dusts under controlled laboratory settings. Current laboratory dust generation systems would benefit from a critical evaluation of the mechanisms by which they generate dust beyond mere resuspension to include natural eolian processes such as saltation/sandblasting. Without realistically generated aerosols, laboratory-measured dust properties may not capture properties relevant to the natural environment. We describe the development of a benchtop system, the Constant Output Dust Generator (CODG), whose design takes into account the dominant natural physical processes of wind erosion and mineral dust production. The CODG’s major components include a wrist-action shaker, custom-built flask, dilution drum, cyclone, and neutralizer. A carrier gas provides flow through the system resulting in dust entrainment. We achieved constant output, typically <10% variation in aerosol surface area concentration, for both a commercial standard as well as environmental samples (saline crusts and loose sediments). We find that the composition of aerosols generated from the CODG is consistent with the composition of the parent source material. We further show that our system is suitable for determination of reaction rates on suspended dust aerosols. At similar mechanical energy inputs, it generates sufficient material (particle surface area concentrations between 10−5 − 10−3 cm2 cm−3) for many applications from both loose sandy sediment and cohesive evaporite crusts. The CODG represents a system potentially applicable for numerous applications of dust aerosol research.
Copyright © 2021 American Association for Aerosol Research
1. Introduction
There is interest in studying the particulate matter (PM) from mineral dust mobilized by desert soils, dried out lake beds (playas), and other arid landforms due to its impact on nutrient transport and biogeochemical cycles (Barkley et al. Citation2019; Jickells et al. Citation2005; Okin et al. Citation2004), ecology and water resources (Field et al. Citation2010; Wu et al. Citation2018), visibility (Mahowald et al. Citation2007), climate (Gaston et al. Citation2017; Haywood and Boucher Citation2000; Pratt et al. Citation2010), and human health (Cahill et al. Citation1996; Meng and Lu Citation2007; Prospero Citation1999; Prospero et al. Citation2014; Sjogren Citation1997; Szema et al. Citation2014). Dust emissions in many regions are predicted to increase with increased aridity and changing land and water use practices (Achakulwisut et al. Citation2019; AghaKouchak et al. Citation2015; Feng et al. Citation2020; Jones and Fleck Citation2020; Parajuli and Zender Citation2018; Tong et al. Citation2017). The repercussions and effects of dust can be better mitigated if the fate, nature, and properties of dust itself are well-known. Systems that generate dust under controlled conditions in the laboratory (summarized in Gill, Zobeck, and Stout Citation2006) are useful for such investigations. Bench scale dust generation systems offer flexibility in experimental design and can be assembled with low cost materials, and thus, have become a popular means to study dust mobilization in the laboratory. An ideal system would generate PM through simulating processes occurring in the ambient environment as accurately as possible.
Mineral dust is primarily formed by the mechanical fracture or wear of larger grains and aggregates. Several processes, either anthropogenic or naturally occurring, can increase dust mobilization. Mechanical energy from human activity results in fugitive dust. Eolian (wind-generated) dust, on the other hand, can come from several interacting processes, including saltation (Cahill et al. Citation1996), sandblasting (Gomes et al. Citation1990; Grini and Zender Citation2004; Grini, Zender, and Colarco Citation2002), and entrainment (Parajuli et al. Citation2016; Zender, Bian, and Newman Citation2003). Saltation (hopping of sand-sized particles) disaggregates sediment into clay-sized (<2 µm) and silt-sized (2–50 µm) particles, forming aerosolized material that is transported along with sand (>50 µm) in a near-surface saltation layer (Cahill et al. Citation1996). Soils that produce high concentrations of mineral dust generally have finer textural classes, such as clays and silts, occurring as aggregates weakly cemented or coated together by salts, other minerals, or organic materials until broken apart by the effects of saltation (Avecilla, Panebianco, and Buschiazzo Citation2016; Mirzamostafa et al. Citation1998; Tatarko et al. Citation2021). The dust emission potential of a soil depends on its dry aggregate stability (DAS) (Swet and Katra Citation2016), a measurement of how tightly soil aggregates remain intact under the influence of an external force (such as saltating particles) prior to breaking-up into their component pieces (i.e., dust). When disaggregation of particles in the saltation layer becomes intense under strong wind shear, sandblasting can further increase aerosol production by breaking up grain surface coatings and releasing them as aerosols (Gomes et al. Citation1990). These processes have been observed in the eolian abrasion of natural dune sands (Bullard, McTainsh, and Pudmenzky Citation2007; Swet et al. Citation2019). Direct aerodynamic entrainment is the immediate vertical (re)suspension of aerosols from the land surface through the action of wind alone without saltation (Zender, Bian, and Newman Citation2003), and, while less frequently observed than the other mechanisms, can be locally important, such as when loose salts covering a playa (exposed by a shrinking saline lake) are mobilized (Macpherson et al. Citation2008). By any of the three mechanisms, mineral dust aerosols that can escape the atmospheric boundary layer may have a long (days to weeks) airborne residence time (Goodman et al. Citation2019; Kandakji, Gill, and Lee Citation2020).
Laboratory production of mineral dust aerosol from sediment samples is used to quantify dust chemical and physical properties, evaluate DAS, or address its toxicology. For example, dusts have been tested in the laboratory to probe optical properties (Engelbrecht et al. Citation2016), particle size distributions as a function of soil properties (Zobeck, Gill, and Popham Citation1999), heterogeneous chemistry (Mitroo et al. Citation2019; Tang et al. Citation2012; Wagner et al. Citation2008), cloud droplet formation (Gaston et al. Citation2017; Sullivan et al. Citation2009), and for scoping of dust emission potential of newly investigated sources (Singer et al. Citation2003). Additionally, dust aerosol generated from surface soil or sediment samples have been collected on filter media and analyzed for elemental content (Engelbrecht et al. Citation2016; Hahnenberger and Perry Citation2015), morphology (Cambra-Lopez et al. Citation2011), mineralogy (Dobrzhinsky et al. Citation2012), and to determine biochemical and organic components of different dust-producing soils (Acosta-Martinez and Zobeck Citation2004). For purposes of environmental health research, dust generators have also been used to extract large quantities of fine particulate matter from masses of soil for bioaccessibility (fraction of dust available for absorption by the bloodstream) and toxicity testing (Gonzales et al. Citation2014; Goossens Citation2012) and to physically suspend dust from soil in inhalation exposure chambers (Bau et al. Citation2018). These latter studies, though, generally do not accurately mimic dust generation by wind-driven processes from their sources, and instead, simply resuspend the material collected.
To accurately generate mineral dust at the bench scale, it is important to simulate the natural processes of its emission while maintaining a constant output. Maintaining constant output eliminates time-dependent phenomena, such as concentration gradients, which can simplify data analysis. For these purposes, a bench-scale dry generation system is preferred. Wet generation techniques (e.g., dispersing sample in water and atomizing it) can lead to unrealistic measurement properties (Biskos et al. Citation2008; Pokharel et al. Citation2019). Bench-scale dry generation systems are summarized by Gill, Zobeck, and Stout (Citation2006), categorizing them in three broad classes based on aerosol production technique. We developed a mineral dust generator to match recommendations of Gill, Zobeck, and Stout (Citation2006). Our system generates a constant, stable output (measured in terms of aerosol surface area-weighed concentration and size distribution) using a methodology representing reasonable simulation of natural eolian processes. We focus our analysis on dusts emitted by playas, due to their increasing global presence (Hassani et al. Citation2020; Wang et al. Citation2018) and their role in both atmospheric chemistry (Gaston Citation2020; Gaston et al. Citation2017; Mitroo et al. Citation2019) and air quality (AghaKouchak et al. Citation2015; Cahill et al. Citation1996). However, the applications for our generator include, but are not limited to, mineral dusts from other sources and other outstanding research questions related to dust PM.
In this work, we first tested the reproducibility, size distribution, and stability of dust generated using a commercial standard. We then used environmental samples to generate dust aerosols and compared the amount and variance of dust aerosolized from different sediment types as well as the chemical composition of parent sediments to generated dust to assess aerosolization pathways potentially promoted by our generation system. Finally, we assessed the suitability of our system for studying heterogeneous reactions with environmental sediment samples from known dust sources. The development of this method and recommendations for further improvements are also discussed in this work.
2. Description of the Constant Output Dust Generator (CODG)
represents a diagram of the Constant Output Dust Generator (CODG) and shows its major components: a wrist-action shaker, a custom-built flask, a dilution drum, a cyclone, and a neutralizer. Typically, 50 cm3 of material is loaded into a 100 mL pyrex Erlenmeyer flask (Chemglass; Vineland, NJ, USA) modified (by Lillie Glass Blowers Inc.; Smyrna, GA, USA) to include a tangential inlet at its base for flowing carrier gas (see insert (a) in ) facilitating the production of cyclonic flow.
Figure 1. Schematic of the CODG. The flask (insert a) is modified from a 100 mL pyrex Erlenmeyer flask. The schematic shows the location of each major piece of equipment (not to scale) on the benchtop, excluding clamp stands and frames. Black lines represent black conductive tubing, and hollow lines represent stainless steel tubing. This assembly is designed to fit on any standard laboratory bench. Details of the dilution drum and manufacturer information are in the text.
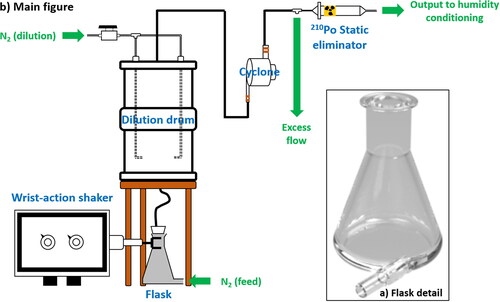
The flask is attached to a wrist-action shaker that supplies mechanical energy to the system. Sediment sample aggregates become broken up by contact with each other and, minimally, the glass walls, replicating the kinetic energy of saltation. The resulting fine disaggregated particles (i.e., aerosols) are then winnowed by the flow. A rubber stopper is secured in the mouth of the flask with elastic bands that prevent dislodging under positive pressure and vibrations from the shaker. A 6 mm hole in the stopper allows a tube to be inserted without leakage. The aerosol-laden flow is directed through this tube toward the bottom of a ∼17 L cylindrical drum. To minimize dead space, thereby stabilizing the flow, two additional angled jets of nitrogen carrier gas are introduced at the base of the drum to create a swirl. This design maximizes the residence time of aerosol in the drum, creating a homogeneous concentration with minimal fluctuations in particle concentrations, as discussed in Section 4. The outlet is at the top of the drum where the dust then enters a cyclone (Mesalabs; Model SCC 2.229) that impacts large particles. This cyclone can be operated to allow PM1, PM2.5, or PM10 downstream. The size-selected dust is then neutralized by two 210Po strips (NRD, LLC; Model 2U500) secured in series to custom housing (Brechtel Manufacturing), to remove static charge thereby increasing aerosol throughput. Finally, the dust aerosol mixes with a humidified nitrogen flow and is then delivered downstream, in our case, to a flow tube reactor. Compared to commercial dust generators, the main components (shaker, drum, neutralizer) of this setup are quite inexpensive.
Inspired by the designs of Lafon et al. (Citation2014), Sullivan et al. (Citation2009), and Vlasenko et al. (Citation2005), our system is optimized to promote entrainment of dust aerosol into an aerosol kinetics flow tube used to study heterogeneous reactions of trace gases on dust (Mitroo et al. Citation2019), while generating dust from sediment samples via energetic disaggregation simulating saltation or sandblasting. The primary design challenges in this system are the generation of a stabilized flow field, and the removal of charge from the aerosol to prevent wall losses in downstream applications. Vlasenko et al. (Citation2005) employed a commercial dust generator that uses a conveyor belt, thus having no actual in-situ dust production, rather, direct resuspension. Sullivan et al. (Citation2009) employed sonication, which is not a dust generation process in the natural environment and was found to be ineffective at generating stable total particle surface areas suitable for our application. Dust generated in the CODG is representative of eolian mineral dust, rather than fugitive dust resuspension by vehicular or industrial processes (for which a rotating brush or fluidized bed is preferred) (Gill, Zobeck, and Stout Citation2006).
3. Materials and methods
We used Arizona Test Dust (ATD; ISO 12103-1 Ultrafine standard; Powder Technology, Inc.) as a commercially available, well-studied dust standard (Tang, Schuster, and Crowley Citation2014; Vlasenko et al. Citation2005; Wagner, Schuster, and Crowley Citation2009) to test the initial development of our setup.Upon a satisfactory quantitative assessment of the dust output stability (<10% change in dust concentration), we generated dust from sediment collected from the surface of playas in Southwest North America: Black Rock Desert (Pershing County, Nevada), Great Salt Lake (Salt Lake County, Utah), Lordsburg Playa (Hidalgo County, New Mexico), Owens Lake (Inyo County, California), Salton Sea (Riverside County, California), and Sulphur Springs Draw (Howard County, Texas). Information about the playa dust samples is available in our previous publication (Mitroo et al. Citation2019). All samples were unaltered before dust was generated from them, except for the sample from the Great Salt Lake, which was dried at 45 °C for 48 h to remove excess moisture, a method mimicking how the perimeter of the Great Salt Lake dries out naturally into an evaporite crust (Wurtsbaugh et al. Citation2017). Visually, only the Great Salt Lake sample and Owens Lake “crust” sample were hard crusts; all other samples were loose sediments (mixtures of gravel, sand, silt, and clay), with Lordsburg and Sulphur Springs Draw playa appearing to be almost entirely sand-sized grains and aggregates (see Figure S1 of the online supplementary information for photos of each sediment sample). The Salton Sea sample was unique in that we observed feathers, bone fragments, and miscellaneous remnants of biological material amongst the sediment, indicative of agricultural practices and ecosystem development in the area (Heness, Simpson, and Bogner Citation2017).
For our application, accurate determination of gas-phase uptake on the aerosol requires that aerosol surface area size distributions produced by the CODG be stable for at least 15 min. To confirm this, dust aerosols generated using the CODG were monitored online with a scanning mobility particle sizer (SMPS; model 3082, TSI, Inc.) and aerosol particle sizer (APS; model 3321, TSI, Inc.) sampling in parallel. The SMPS scanned mobility diameters (Dm) in the range of 11.8–593.5 nm, and the APS measured aerodynamic diameters (Da) in the range of 542–18,810 nm. We also performed offline ion chromatography (IC) analysis to compare the sediment soluble ion composition to the generated aerosol composition (for particles collected in the catchment of the cyclone). This analysis was performed to determine how representative the aerosols generated by our CODG are. Our method for IC analysis can be found in Mitroo et al. (Citation2019). Briefly, ∼10 mg of crushed sediment was added to 30 mL Milli-Q water (>18 MΩ resistivity), and the solution aliquot was then sonicated at 60 °C for 75 min. The resulting suspension was filtered using a 0.22 µm syringe filter (Acrodisc, Pall Life Sciences) during injection into the ion chromatographs (Dionex ICS-1100 for cations and ICS-2100 for anions). Soluble inorganic ion concentrations were measured using two different IC systems: for anions (Cl-, NO2-, SO42-, Br-, NO3-, PO43-), and cations (Na+, NH4+, K+, Mg2+, Ca2+). All samples were run in triplicate. Finally, sample blanks were produced by filtering Milli-Q water in the absence of sediment grains and performing IC using the same method.
4. Results and discussion
4.1. Development of the dust generation system
Our system was optimized to promote entrainment of dust aerosol, after generating dust from sediment samples via energetic disaggregation as would occur during sandblasting. The method for generating mechanical energy to aerosolize our sediment samples was the major design factor in our system. We tested different methods using ATD as our sediment standard. For our application, we required particle surface area concentrations within the range of 10−5 − 10−3 cm2 cm−3 that were stable to within 10% over a 15 min period.
Initially, we immersed a flask containing the ATD sample in a sonicator (Bransonic Ultrasonic Bath M3800), a method successfully employed for studies of aerosol hygroscopicity that requires stable number concentrations at discrete particle sizes (Gaston et al. Citation2017; Sullivan et al. Citation2009). However, stable particle surface areas were not generated by this method (), as evidenced by the large standard error bars. Similarly, we found no significant improvement in aerosol output stability using a vortex shaker (Fisher Scientific) () or an orbital shaker (Lab-Line Instruments) at low speed (), neither having been used before for this application, to the best of our knowledge. We increased the energy output of the orbital shaker to observe if this would improve aerosol production (), and a significant improvement was achieved. We do note that the small error bars shown in confirms the stability of dust aerosol production; however, such stability with the orbital shaker was not obtained in repeated tests, showing a lack of reproducibility. In addition, the glass flask on the orbital shaker operated at high speeds experienced frequent dislodging, requiring constant attention.
Figure 2. The mean ATD aerosol size distributions are shown for different mechanical energy sources: (a) sonicator, (b) vortex shaker, (c) orbital shaker at low speed, (d) orbital shaker at high speed, and (e) wrist-action shaker. Red markers indicate SMPS data, whereas blue markers indicate APS data. Error bars represent ± 1σ. Mobility diameter (Dm) and aerodynamic diameter (Da) were converted to volume-equivalent diameter (DVE) using the calculations and parameters found in the online supplementary information of Mitroo et al. (Citation2019). The symbol nA refers to the aerosol surface area distribution. The selected distributions represent the best available data for each mechanical energy source. Note that for (a), the flow through the cyclone was set for a PM2.5 cutoff, whereas the rest of the data in this figure is for a PM1 cutoff. Considering the poor performance in (a), we did not replicate the experiment at PM1 cutoff and discarded the choice of a sonicator. Vertical lines are included to show the respective cutoffs using a short-dashed line (PM1) and long-dashed line (PM2.5).
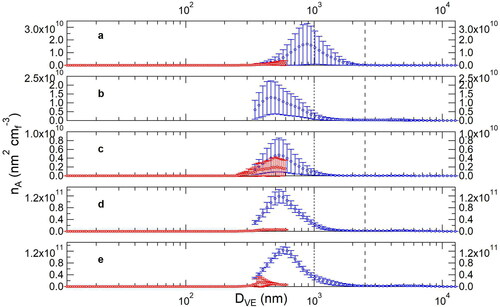
Lafon et al. (Citation2014) successfully employed the use of wrist-action shakers in the development of the Générateur d’Aérosol Minéral En Laboratoire (GAMEL) for the production of dust. Yet, a pronounced decay over the course of minutes was observed in their work. We employed a wrist-action shaker (Lab-Line Instruments) as a mechanical energy source into our system with success (). The stability of aerosol production is due to the wrist action shaker, the tangential inlet modification to the flask (insert (a) in ), and the dilution drum. The combination of mechanical agitation and tangential flow eased entrainment, and the swirl promoted in the dilution drum facilitated sample homogenization through a reduction in dead volume (increasing the mean residence time of the actively mixing volume). We were successful in generating a constant output of dust with surface area-weighed aerosol size distributions varying <10% (). An advantage of the wrist-action shaker over the orbital shaker at high energy inputs was the higher total surface area generated: appropriate for probing heterogeneous reactions.
4.2. On the aerosolization processes occurring in the CODG
After we optimized our system using ATD, we assessed the robustness of the CODG using playa sediment samples, which are more representative of natural terrestrial dust sources than ATD. compares how the CODG performed for different playa sediment material types, in this case, loose sediments and solidified crusts. Surprisingly, no additional mechanical energy (e.g. rotating brush) was necessary to generate dust from solidified crustal material. Despite cohesion of evaporites on the sediment grains, abrasion of the grains against each other (and, minimally, the glass walls) was sufficient to generate dust (see for photos of a hard crust sediment before and after dust generation). Moreover, solid salt crusts generated more surface area than loose sediment, which we attribute to the increased presence of brittle and soft evaporite minerals that are readily aerosolized. This is a major strength of the CODG: at a similar mechanical energy input, it can generate sufficient dust surface areas for our application (Section 4.3) from both loose sandy sediment and cohesive evaporite crusts.
Figure 3. Comparison of dust emission, reported as total surface area concentration, at the same wrist-action shaker setting (i.e., mechanical energy input) at 30% RH conditioning. Great Salt Lake and Owens Lake (cold colors) represent solid crusts, whereas Sulphur Springs Draw and Lordsburg (warm colors) represent loose sediment. “High dilution” refers to N2 (dilution)/N2 (feed) ≫1, whereas “low dilution” refers to N2 (dilution)/N2 (feed) ∼1. Please refer to for details.
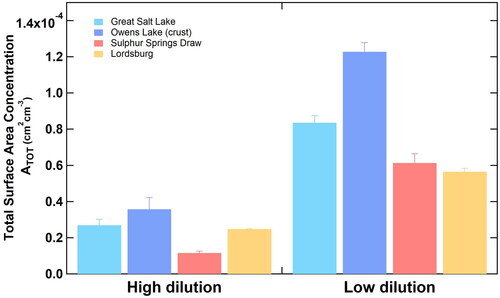
The CODG allowed several surface area concentrations to be achieved. illustrates the total particle surface area generated at 30% RH at different amounts of dilution for solid crusts and loose sediments. Small error bars (≤10%, except for Owens Lake crust at high dilution) indicate tight control over aerosol surface concentrations. A simple change in settings of the downstream dilution (either in the dilution drum or mixing with humidified air) can increase or decrease the aerosol concentrations, offering flexibility to the user.
Another important consideration was that the CODG could generate aerosols that were chemically representative of the wide range of minerals found in our different playa sediment samples, which are a complex mixture of clay minerals and evaporitic salts. Both sediment and aerosols (>PM1 aerosols collected in the cyclone bycatch) were analyzed via ion chromatography (IC), see . The soluble inorganic ion composition of Black Rock Desert and Great Salt Lake samples, representative of more compact, solidified crusts, are similar for both the source sediment and evolved aerosol, whereas for looser sediment, such as Sulphur Springs Draw, the soluble ion composition of source sediment and aerosol are markedly different. For the more chemically homogeneous evaporite crust samples (the result of crystal growth from evaporated brine) disaggregation and sandblasting cannot be distinguished ( and ). However, IC data suggests that for loose playa sediments, a sandblasting-like effect was achieved in the CODG, removing chemically distinct coatings on the grains.
Figure 4. Bulk soluble inorganic ion composition for sediment and aerosols > PM1 (collected in cyclone bycatch) for six samples, showing the mass fraction contributions to the total mass. Unmeasured, primarily insoluble, chemical components corresponded to 40–90% of the total sample masses.
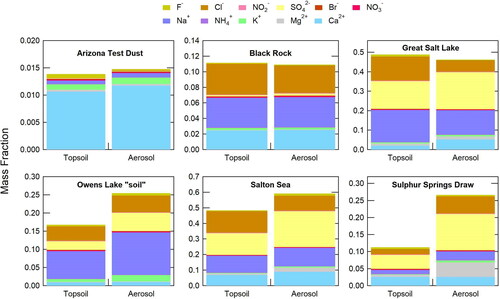
For example, sodium (Na+), magnesium (Mg2+), chloride (Cl-), and sulfate (SO42-) are more abundant in the Sulphur Springs Draw aerosol than the source material, composed primarily of clastic sediments (silicate mineral grains) with salt coatings. If dust source sediment grains are held together or coated by salts, such as at Sulphur Springs Draw (Frederick Citation1998), the evolved aerosol would be enhanced in the salt component (Pye Citation1989), enhancing the soluble fraction of the dust, such as in the case of the Sulphur Springs sample. This difference suggests an improved simulation of wind erosion occurring in our setup compared to those employing mere resuspensions. Using the CODG for size-resolved chemical composition studies of dusts could thus shed light on the physical processes generating mineral aerosols from different source materials.
4.3. Proof of concept: Application to heterogeneous dust chemistry
To test the usefulness of the CODG for atmospheric applications, we generated aerosolized playa dusts used to probe the reactive uptake of dinitrogen pentoxide (N2O5) and subsequent heterogeneous production of nitryl chloride (ClNO2) by coupling the CODG to a flow tube set up (Mitroo et al. Citation2019). To successfully use the CODG for this application, stable particle surface areas were essential for the duration of the experiment. is an example of total particle surface area measured from dust generated from the Lordsburg playa sample.
Figure 5. Timeseries showing the stability of the total dust aerosol surface area in our system. The symbol nA, labeling the y-axes of the inserts, refers to the aerosol surface area distribution. The insets represent the mean ±1σ of the size distribution during the last 10 min of each 15-min dust generation period.
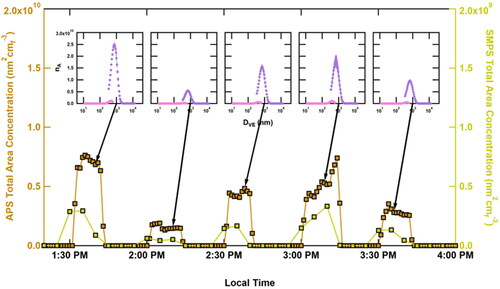
Typically, in an uptake experiment, the first five minutes of data are discarded due to lag associated with ramp-up of flow in the conical flask, residence time in the dilution drum and tubing, and instrument response time. A typical experiment cycled 15 min without dust (to ascertain wall losses of N2O5) and 15 min with dust for a total of five repetitions. The total surface area was varied intentionally (through varying dilution during RH conditioning) throughout the experiment to ensure linear kinetics (i.e., no surface saturation of N2O5) validating measurement of N2O5 uptake coefficients. Compared to the one-time success of the orbital shaker, the wrist-action shaker proved effective in the CODG development. As shown in , the wrist-action shaker generated stable particle surface areas for 15 min that were reproducible over the course of an N2O5 uptake experiment, involving five repetitions, for a total experiment duration of ∼3.5 h. This ability to produce stable output is the CODG’s primary strength making this system suitable for probing atmospheric reactions and, likely, other applications in the laboratory as well.
4.4. Summary and outlook
Several methods for aerosolizing dust from sediment samples have been made available: the GAMEL (Lafon et al. Citation2014), UC Davis Resuspension Chamber (Carvacho et al. Citation2001), cone abrader (Chandler et al. Citation2002), SyGAVib vibrating system (Qu et al. Citation2020), and Easy Dust Generator rotating bottle (Mendez, Panebianco, and Buschiazzo Citation2013) being noteworthy for their unique design for bench-top applications. The CODG described herein is capable of incorporating their strengths (reasonable simulation of natural processes of dust generation) with constant and stable output. The CODG’s theoretical applications are backed by testing it both with a commercially available dust (ATD) and natural playa sediment samples, which are particularly challenging to work with in the laboratory due to their heterogeneous nature. By demonstrating proof of concept on both types of samples, we show that the CODG is suitable for aerosol chemistry applications including reactive uptake experiments (e.g., heterogeneous chemistry). We point out that the CODG is also well-suited toward use in health and climate related applications on a wide variety of substances and sediment types.
Through the CODG, we achieved an average particle surface area that was stable (within 10%) for up to 15 min. Previous studies using different dust generation designs to probe the reactive uptake of gases to dust have noted the difficulty in generating stable particle surface areas. Notably, the limitation in our system to 15 min was not due to the system becoming unstable, but rather the accumulation of dust on the critical orifice that is the entrance to our mass spectrometer. The CODG also generated aerosol that was chemically representative of the diverse minerals found in our playa sediment samples. This is an important consideration because of the relevance of chemical composition in determining not only the reactive uptake kinetics probed in our application, but also the impact of dust composition on health and climate.
The CODG, and other impact-based bench-scale dust generators (Gill, Zobeck, and Stout Citation2006; Mockford Citation2013; Singer et al. Citation2003), as in most eolian processes in nature, produces dust aerosol in-situ by the comminution of much larger grains of the parent sediment sample through the application of mechanical energy.While the benchtop-scale CODG does not create saltation, it simulates it from the provision of mechanical energy in the wrist-action-shaken flask, in an analogous manner to room-scale systems generating dust in a barrel (Gill et al. Citation1999; Singer et al. Citation2003; Singh Citation1994; Zobeck, Gill, and Popham Citation1999). We have shown that dust generated from environmental sediment samples in the CODG produces stable particle surface areas, particles representative in composition to their parent sediments, and can be readily used in experiments probing atmospheric chemistry. Additional gaps in the understanding of radiative heat transfer, biogeochemical properties of dust, and health effects arising from dust exposure can be further constrained by application of laboratory-scale controlled studies using tools such as the CODG to address these research questions.
Supplemental Material
Download MS Word (1 MB)Acknowledgments
We thank one anonymous reviewer for comments that improved our manuscript. We are very grateful to the following individuals for collection of samples: Prof. Heather Holmes and Prof. Bernhard Bach (Black Rock Desert playa); Prof. Maura Hahnenberger (Great Salt Lake playa); Prof. Joanna Nield, Jana Lasser, and Lucas Goehring (Owens Lake playa); Prof. Roya Bahreini (Salton Sea playa); and Dr. R. Scott Van Pelt (Sulphur Springs Draw). We thank Prof. Andrew Baker for use of the orbital shaker, and Prof. Kimberly Popendorf for use of the vortex shaker in troubleshooting the development of the CODG.
Disclosure statement
No potential conflict of interest was reported by the author(s).
Additional information
Funding
References
- Achakulwisut, P., S. C. Anenberg, J. E. Neumann, S. L. Penn, N. Weiss, A. Crimmins, N. Fann, J. Martinich, H. Roman, and L. J. Mickley. 2019. Effects of increasing aridity on ambient dust and public health in the U.S. Southwest under climate change. Geohealth. 3 (5):127–44. doi:https://doi.org/10.1029/2019GH000187.
- Acosta-Martinez, V., and T. M. Zobeck. 2004. Enzyme activities and arylsulfatase protein content of dust and the soil source: Biochemical fingerprints? J. Environ. Qual. 33 (5):1653–61. doi:https://doi.org/10.2134/jeq2004.1653.
- AghaKouchak, A., H. Norouzi, K. Madani, A. Mirchi, M. Azarderakhsh, A. Nazemi, N. Nasrollahi, A. Farahmand, A. Mehran, and E. Hasanzadeh. 2015. Aral Sea syndrome desiccates Lake Urmia: Call for action. J. Great Lakes Res. 41 (1):307–11. doi:https://doi.org/10.1016/j.jglr.2014.12.007.
- Avecilla, F., J. E. Panebianco, and D. E. Buschiazzo. 2016. A wind-tunnel study on saltation and PM10 emission from agricultural soils. Aeolian Res. 22:73–83. doi:https://doi.org/10.1016/j.aeolia.2016.06.003.
- Barkley, A. E., J. M. Prospero, N. Mahowald, D. S. Hamilton, K. J. Popendorf, A. M. Oehlert, A. Pourmand, A. Gatineau, K. Panechou-Pulcherie, P. Blackwelder, et al. 2019. African biomass burning is a substantial source of phosphorus deposition to the Amazon, Tropical Atlantic Ocean, and Southern Ocean. Proc. Natl. Acad. Sci. U S A. 116 (33):16216–21. doi:https://doi.org/10.1073/pnas.1906091116.
- Bau, S., S. Bourrous, F. Gaie-Levrel, and O. Witschger. 2018. Characterization of aerosols generated from nine nanomaterial powders: reliability with regard to in vivo inhalation toxicology studies. J. Nanopart. Res. 20 (10):276. doi:https://doi.org/10.1007/s11051-018-4381-5.
- Biskos, G., V. Vons, C. U. Yurteri, and A. Schmidt-Ott. 2008. Generation and sizing of particles for aerosol-based nanotechnology. KONA 26:13–35. doi:https://doi.org/10.14356/kona.26.2008006.
- Bullard, J. E., G. H. McTainsh, and C. Pudmenzky. 2007. Factors affecting the nature and rate of dust production from natural dune sands. Sedimentology 54 (1):169–82. doi:https://doi.org/10.1111/j.1365-3091.2006.00827.x.
- Cahill, T. A., T. E. Gill, J. S. Reid, E. A. Gearhart, and D. A. Gillette. 1996. Saltating particles, playa crusts and dust aerosols at Owens (dry) Lake California. Earth Surf. Process. Landforms 21 (7):621–39. doi:https://doi.org/10.1002/(SICI)1096-9837(199607)21:7<621::AID-ESP661>3.0.CO;2-E.
- Cambra-Lopez, M., A. G. Torres, A. J. A. Aarnink, and N. W. M. Ogink. 2011. Source analysis of fine and coarse particulate matter from livestock houses. Atmos. Environ. 45 (3):694–707. doi:https://doi.org/10.1016/j.atmosenv.2010.10.018.
- Carvacho, O. F., L. L. Ashbaugh, M. S. Brown, and R. G. Flocchini. 2001. Relationship between San Joaquin Valley soil texture and PM10 emission potential using the UC Davis Dust Resuspension Test Chamber. Trans. ASAE 44 (6):1603–8.
- Chandler, D. G., K. E. Saxton, J. Kjelgaard, and A. J. Busacca. 2002. A technique to measure fine-dust emission potentials during wind erosion. Soil Sci. Soc. Am. J. 66 (4):1127–33. doi:https://doi.org/10.2136/sssaj2002.1127.
- Dobrzhinsky, N., E. Krugly, L. Kliucininkas, T. Prasauskas, M. Kireitseu, A. Zerrath, and D. Martuzevicius. 2012. Characterization of desert road dust aerosol from provinces of Afghanistan and Iraq. Aerosol Air Qual. Res. 12 (6):1209–16. doi:https://doi.org/10.4209/aaqr.2012.05.0112.
- Engelbrecht, J. P., H. Moosmuller, S. Pincock, R. K. M. Jayanty, T. Lersch, and G. Casuccio. 2016. Technical note: Mineralogical, chemical, morphological, and optical interrelationships of mineral dust re-suspensions. Atmos. Chem. Phys. 16 (17):10809–30. doi:https://doi.org/10.5194/acp-16-10809-2016.
- Feng, X. Y., R. Mao, D. Y. Gong, C. Zhao, C. L. Wu, C. F. Zhao, G. J. Wu, Z. H. Lin, X. H. Liu, K. C. Wang, et al. 2020. Increased dust aerosols in the high troposphere over the Tibetan plateau from 1990s to 2000s. J Geophys. Res.-Atmospheres 125 (13):e2020JD032807.
- Field, J. P., J. Belnap, D. D. Breshears, J. C. Neff, G. S. Okin, J. J. Whicker, T. H. Painter, S. Ravi, M. C. Reheis, and R. L. Reynolds. 2010. The ecology of dust. Front. Ecol. Environ. 8 (8):423–30. doi:https://doi.org/10.1890/090050.
- Frederick, C. D. 1998. Late Quaternary clay dune sedimentation on the Llano Estacado, Texas. Plains Anthropol. 43 (164):137–55. doi:https://doi.org/10.1080/2052546.1998.11931896.
- Gaston, C. J. 2020. Re-examining dust chemical aging and its impacts on earth's climate. Acc. Chem. Res. 53 (5):1005–13. doi:https://doi.org/10.1021/acs.accounts.0c00102.
- Gaston, C. J., K. A. Pratt, K. J. Suski, N. W. May, T. E. Gill, and K. A. Prather. 2017. Laboratory studies of the cloud droplet activation properties and corresponding chemistry of saline playa dust. Environ. Sci. Technol. 51 (3):1348–56. doi:https://doi.org/10.1021/acs.est.6b04487.
- Gill, T. E., T. M. Zobeck, and J. E. Stout. 2006. Technologies for laboratory generation of dust from geological materials. J. Hazard. Mater. 132 (1):1–13. doi:https://doi.org/10.1016/j.jhazmat.2005.11.083.
- Gill, T. E., T. M. Zobeck, J. E. Stout, and J. M. Gregory. 1999. Fugitive dust generation in the laboratory. In Proceedings of the Wind Erosion International Symposium-Workshop USDA-ARS, Wind Erosion Research Unit, Kansas State University, Manhattan, KS, 6.
- Gomes, L., G. Bergametti, G. Coudé-Gaussen, and P. Rognon. 1990. Submicron desert dusts - a sandblasting process. J. Geophys. Res. 95 (D9):13927–35. doi:https://doi.org/10.1029/JD095iD09p13927.
- Gonzales, P., O. Felix, C. Alexander, E. Lutz, W. Ela, and A. E. Saez. 2014. Laboratory dust generation and size-dependent characterization of metal and metalloid-contaminated mine tailings deposits. J. Hazard. Mater. 280:619–26. doi:https://doi.org/10.1016/j.jhazmat.2014.09.002.
- Goodman, M. M., G. T. Carling, D. P. Fernandez, K. A. Rey, C. A. Hale, B. R. Bickmore, S. T. Nelson, and J. S. Munroe. 2019. Trace element chemistry of atmospheric deposition along the Wasatch Front (Utah, USA) reflects regional playa dust and local urban aerosols. Chem. Geol. 530:119317. doi:https://doi.org/10.1016/j.chemgeo.2019.119317.
- Goossens, D. 2012. A method for dry extracting large volumes of fine particulate matter from bulk soil samples. Air Qual. Atmos. Health 5 (4):425–31. doi:https://doi.org/10.1007/s11869-011-0142-7.
- Grini, A., and C. S. Zender. 2004. Roles of saltation, sandblasting, and wind speed variability on mineral dust aerosol size distribution during the Puerto Rican Dust Experiment (PRIDE). J. Geophys. Res. 109 (D7):D07202. doi:https://doi.org/10.1029/2003JD004233.
- Grini, A., C. S. Zender, and P. R. Colarco. 2002. Saltation Sandblasting behavior during mineral dust aerosol production. Geophys. Res. Lett. 29 (18):15-1––4. doi:https://doi.org/10.1029/2002GL015248.
- Hahnenberger, M., and K. D. Perry. 2015. Chemical comparison of dust and soil from the Sevier Dry Lake, UT, USA. Atmos. Environ. 113:90–7. doi:https://doi.org/10.1016/j.atmosenv.2015.04.054.
- Hassani, A., A. Azapagic, P. D'Odorico, A. Keshmiri, and N. Shokri. 2020. Desiccation crisis of saline lakes: A new decision-support framework for building resilience to climate change. Sci. Total Environ. 703:134718. doi:https://doi.org/10.1016/j.scitotenv.2019.134718.
- Haywood, J., and O. Boucher. 2000. Estimates of the direct and indirect radiative forcing due to tropospheric aerosols: A review. Rev. Geophys. 38 (4):513–43. doi:https://doi.org/10.1029/1999RG000078.
- Heness, E. A., E. L. Simpson, and E. Bogner. 2017. The shoreline distribution and degradation of tilapia carcasses, Salton Sea, California: Taphonomic implications. Palaeogeogr. Palaeoclimatol. Palaeoecol. 468:276–86. doi:https://doi.org/10.1016/j.palaeo.2016.12.027.
- Jickells, T. D., Z. S. An, K. K. Andersen, A. R. Baker, G. Bergametti, N. Brooks, J. J. Cao, P. W. Boyd, R. A. Duce, K. A. Hunter, et al. 2005. Global iron connections between desert dust, ocean biogeochemistry, and climate. Science 308 (5718):67–71. doi:https://doi.org/10.1126/science.1105959.
- Jones, B. A., and J. Fleck. 2020. Shrinking lakes, air pollution, and human health: Evidence from California's Salton Sea. Sci. Total Env. 712:136490. doi:https://doi.org/10.1016/j.scitotenv.2019.136490.
- Kandakji, T., T. E. Gill, and J. A. Lee. 2020. Identifying and characterizing dust point sources in the southwestern United States using remote sensing and GIS. Geomorphology 353:107019. doi:https://doi.org/10.1016/j.geomorph.2019.107019.
- Lafon, S., S. C. Alfaro, S. Chevaillier, and J. L. Rajot. 2014. A new generator for mineral dust aerosol production from soil samples in the laboratory: GAMEL. Aeolian Res. 15:319–34. doi:https://doi.org/10.1016/j.aeolia.2014.04.004.
- Macpherson, T., W. G. Nickling, J. A. Gillies, and V. Etyemezian. 2008. Dust emissions from undisturbed and disturbed supply-limited desert surfaces. J. Geophys. Res 113 (F2):F02S04. doi:https://doi.org/10.1029/2007JF000800.
- Mahowald, N. M., J. A. Ballantine, J. Feddema, and N. Ramankutty. 2007. Global trends in visibility: implications for dust sources. Atmos. Chem. Phys. 7 (12):3309–39. doi:https://doi.org/10.5194/acp-7-3309-2007.
- Mendez, M. J., J. E. Panebianco, and D. E. Buschiazzo. 2013. A new dust generator for laboratory dust emission studies. Aeolian Res. 8:59–64. doi:https://doi.org/10.1016/j.aeolia.2012.10.007.
- Meng, Z. Q., and B. Lu. 2007. Dust events as a risk factor for daily hospitalization for respiratory and cardiovascular diseases in Minqin. China. Atmos. Environ. 41 (33):7048–58. doi:https://doi.org/10.1016/j.atmosenv.2007.05.006.
- Mirzamostafa, N., L. J. Hagen, L. R. Stone, and E. L. Skidmore. 1998. Soil aggregate and texture effects on suspension components from wind erosion. Soil Sci. Soc. Am. J. 62 (5):1351–61. doi:https://doi.org/10.2136/sssaj1998.03615995006200050030x.
- Mitroo, D., T. E. Gill, S. Haas, K. A. Pratt, and C. J. Gaston. 2019. ClNO2 Production from N2O5 uptake on saline playa dusts: New insights into potential inland sources of ClNO2. Environ. Sci. Technol. 53 (13):7442–52. doi:https://doi.org/10.1021/acs.est.9b01112.
- Mockford, T. W. 2013. Effect of soil texture and calcium carbonate on laboratory‐generated dust emissions from SW North America. [MS Masters Thesis]: Texas Tech University.
- Okin, G. S., N. Mahowald, O. A. Chadwick, and P. Artaxo. 2004. Impact of desert dust on the biogeochemistry of phosphorus in terrestrial ecosystems. Global Biogeochem. Cycles 18 (2):n/a–/a. doi:https://doi.org/10.1029/2003GB002145.
- Parajuli, S. P., and C. S. Zender. 2018. Projected changes in dust emissions and regional air quality due to the shrinking Salton Sea. Aeolian Res. 33:82–92. doi:https://doi.org/10.1016/j.aeolia.2018.05.004.
- Parajuli, S. P., T. M. Zobeck, G. Kocurek, Z. L. Yang, and G. L. Stenchikov. 2016. New insights into the wind-dust relationship in sandblasting and direct aerodynamic entrainment from wind tunnel experiments. J. Geophys. Res. Atmos. 121 (4):1776–92. doi:https://doi.org/10.1002/2015JD024424.
- Pokharel, L., P. Parajuli, L. Li, E. J. Chng, and R. Gopalakrishnan. 2019. An ultrasonic feeding mechanism for continuous aerosol generation from cohesive powders. Aerosol Sci. Technol. 53 (3):321–31. doi:https://doi.org/10.1080/02786826.2018.1559920.
- Pratt, K. A., C. H. Twohy, S. M. Murphy, R. C. Moffet, A. J. Heymsfield, C. J. Gaston, P. J. DeMott, P. R. Field, T. R. Henn, D. C. Rogers, et al. 2010. Observation of playa salts as nuclei in orographic wave clouds. J. Geophys. Res. 115 (D15):D15301. doi:https://doi.org/10.1029/2009JD013606.
- Prospero, J. M. 1999. Assessing the impact of advected African dust on air quality and health in the eastern United States. Human Ecol. Risk Assess. 5 (3):471–9. doi:https://doi.org/10.1080/10807039.1999.10518872.
- Prospero, J. M., F. X. Collard, J. Molinie, and A. Jeannot. 2014. Characterizing the annual cycle of African dust transport to the Caribbean Basin and South America and its impact on the environment and air quality. Global Biogeochem. Cycles 28 (7):757–73. doi:https://doi.org/10.1002/2013GB004802.
- Pye, K., 1989. Processes of fine particle formation, dust source regions, and climatic changes. In Paleoclimatology and paleometeorology: Modern and past patterns of global atmospheric transport, ed. M. Leinen, and M. Sarnthein, Vol. 282, 3–30. NATO ASI Series C. Dordrecht: Kluwer Academic Publishers.
- Qu, Z., A. Trabelsi, R. Losno, F. Monna, S. Nowak, M. Masmoudi, and J. P. Quisefit. 2020. A laboratory dust generator applying vibration to soil sample: Mineralogical study and compositional analyses. J. Geophys. Res.-Atmospheres 125 (7):e2019JD032224.
- Singer, A., T. Zobeck, L. Poberezsky, and E. Argaman. 2003. The PM10 and PM2.5 dust generation potential of soils/sediments in the Southern Aral Sea Basin, Uzbekistan. J. Arid Environ. 54 (4):705–28. doi:https://doi.org/10.1006/jare.2002.1084.
- Singh, U. B. 1994. Wind erosion: Mechanics of saltation and dust generation. [PhD Doctoral Thesis]. Texas Tech University.
- Sjogren, B. 1997. Occupational exposure to dust: Inflammation and ischaemic heart disease. Occup. Environ. Med. 54 (7):466–9. doi:https://doi.org/10.1136/oem.54.7.466.
- Sullivan, R. C., M. J. K. Moore, M. D. Petters, S. M. Kreidenweis, G. C. Roberts, and K. A. Prather. 2009. Timescale for hygroscopic conversion of calcite mineral particles through heterogeneous reaction with nitric acid. Phys. Chem. Chem. Phys. 11 (36):7826–37. doi:https://doi.org/10.1039/b904217b.
- Swet, N., T. Elperin, J. F. Kok, R. L. Martin, H. Yizhaq, and I. Katra. 2019. Can active sands generate dust particles by wind-induced processes? Earth Planet. Sci. Lett. 506:371–80. doi:https://doi.org/10.1016/j.epsl.2018.11.013.
- Swet, N., and I. Katra. 2016. Reduction in soil aggregation in response to dust emission processes. Geomorphology 268:177–83. doi:https://doi.org/10.1016/j.geomorph.2016.06.002.
- Szema, A. M., R. J. Reeder, A. D. Harrington, M. Schmidt, J. X. Liu, M. Golightly, T. Rueb, and S. A. Hamidi. 2014. Iraq dust is respirable, sharp, and metal-laden and induces lung inflammation with fibrosis in mice via IL-2 upregulation and depletion of regulatory T cells. J. Occup. Environ. Med. 56 (3):243–51.
- Tang, M. J., G. Schuster, and J. N. Crowley. 2014. Heterogeneous reaction of N2O5 with illite and Arizona test dust particles. Atmos. Chem. Phys. 14 (1):245–54. doi:https://doi.org/10.5194/acp-14-245-2014.
- Tang, M. J., J. Thieser, G. Schuster, and J. N. Crowley. 2012. Kinetics and mechanism of the heterogeneous reaction of N2O5 with mineral dust particles. Phys. Chem. Chem. Phys. 14 (24):8551–61. doi:https://doi.org/10.1039/c2cp40805h.
- Tatarko, J., M. Kucharski, H. Li, and H. Li. 2021. PM2.5 and PM10 emissions by breakage during saltation of agricultural soils. Soil & Tillage Research 208:104902. doi:https://doi.org/10.1016/j.still.2020.104902.
- Tong, D. Q., J. X. L. Wang, T. E. Gill, H. Lei, and B. Y. Wang. 2017. Intensified dust storm activity and Valley fever infection in the southwestern United States. Geophys. Res. Lett. 44 (9):4304–12. doi:https://doi.org/10.1002/2017GL073524.
- Vlasenko, A., S. Sjogren, E. Weingartner, H. W. Gaggeler, and M. Ammann. 2005. Generation of submicron Arizona test dust aerosol: Chemical and hygroscopic properties. Aerosol Sci. Technol. 39 (5):452–60. doi:https://doi.org/10.1080/027868290959870.
- Wagner, C., F. Hanisch, N. Holmes, H. de Coninck, G. Schuster, and J. N. Crowley. 2008. The interaction of N2O5 with mineral dust: aerosol flow tube and Knudsen reactor studies. Atmos. Chem. Phys. 8 (1):91–109. doi:https://doi.org/10.5194/acp-8-91-2008.
- Wagner, C., G. Schuster, and J. N. Crowley. 2009. An aerosol flow tube study of the interaction of N2O5 with calcite. Arizona dust and quartz. Atmosp. Environ. 43 (32):5001–8. doi:https://doi.org/10.1016/j.atmosenv.2009.06.050.
- Wang, J. D., C. Q. Song, J. T. Reager, F. F. Yao, J. S. Famiglietti, Y. W. Sheng, G. M. MacDonald, F. Brun, H. M. Schmied, R. A. Marston, et al. 2018. Recent global decline in endorheic basin water storages. Nat. Geosci. 11 (12):926–32. doi:https://doi.org/10.1038/s41561-018-0265-7.
- Wu, C. L., X. H. Liu, Z. H. Lin, S. R. Rahimi-Esfarjani, and Z. Lu. 2018. Impacts of absorbing aerosol deposition on snowpack and hydrologic cycle in the Rocky Mountain region based on variable-resolution CESM (VR-CESM) simulations. Atmos. Chem. Phys. 18 (2):511–33. doi:https://doi.org/10.5194/acp-18-511-2018.
- Wurtsbaugh, W. A., C. Miller, S. E. Null, R. J. DeRose, P. Wilcock, M. Hahnenberger, F. Howe, and J. Moore. 2017. Decline of the world's saline lakes. Nature Geosci. 10 (11):816–21. doi:https://doi.org/10.1038/ngeo3052.
- Zender, C. S., H. S. Bian, and D. Newman. 2003. Mineral Dust Entrainment and Deposition (DEAD) model: Description and 1990s dust climatology. J. Geophys. Res. 108 (D14):4416. doi:https://doi.org/10.1029/2002JD002775.
- Zobeck, T. M., T. E. Gill, and T. W. Popham. 1999. A two-parameter Weibull function to describe airborne dust particle size distributions. Earth Surf. Process. Landforms 24 (10):943–55. doi:https://doi.org/10.1002/(SICI)1096-9837(199909)24:10<943::AID-ESP30>3.0.CO;2-9.