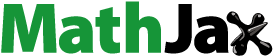
Abstract
While condensation particle counters (CPCs) are routinely used to measure particle number concentrations in the size range from 3 to 1000 nm, the detection efficiency for sub-3 nm particles remains relatively poor. In this study, the performance of an ultrafine butanol based CPC for the measurement of alkyl ammonium halide ions, flame-generated particles of titanium dioxide (TiO2) and soot is evaluated. Homogenous nucleation of butanol vapors, and detection efficiency of THAB ions are systematically evaluated for a range of saturator temperatures (39–45 °C) and capillary flow rates (30–70 sccm). The optimal operating conditions with minimal background interferences was found to be a saturator temperature of 45 °C and capillary flow rate of 70 sccm. Flame generated particles of TiO2 and soot were activated more readily than the alkyl ammonium halide salts of similar mobility diameters, especially in the below 1.6 nm mobility size range, whereas above 1.6 nm the detection efficiency is independent of particle material, but depends on particle size. The negative charge on the particles is found to promote activation with butanol vapors leading to higher activation efficiencies than positively charged particles. Finally, the importance of butanol as a working fluid, and its calibration for precise measurement of sub-3 nm particles is discussed. This will enable researchers to perform experiments using a ‘conventional’ CPC with boosted conditions for the understanding of early stages of particle formation and growth in different aerosol reactors.
Copyright © 2021 American Association for Aerosol Research
EDITOR:
1. Introduction
Early stages of particle formation and growth (1–5 nm) have attracted attention of aerosol scientists for several decades (Biswas, Wang, and Attoui Citation2018). For the atmospheric aerosol, it is important to study the nucleation of new particles from molecular clusters, as they play a crucial role in cloud formation, climate change and suspended particulate matter (Kulmala et al. Citation2007). Ultrafine particles generated through fossil fuel combustion involves both primary (Wang et al. Citation2013) as well as secondary formation pathways (Guo et al. Citation2014). The secondary aerosol formation from precursor vapors is not only limited to fossil fuel combustion, but also diesel/gasoline engines (Gentner et al. Citation2012) and 3 D printers (Zhang, Sharma, Wang, et al. Citation2018). On the other hand, gas-phase synthesis of nanoparticles in a ‘bottom-up’ approach has received tremendous attention due to their unique thermal, acoustic, electrical, and optical properties (Kruis, Fissan, and Peled Citation1998; Strobel and Pratsinis Citation2007). The material synthesis process typically includes precursor to particle conversion, which form the building blocks for larger particles (Li et al. Citation2016). Therefore, a better understanding of particle formation using real time measurements would envisage the development of future particle capture technologies to clean air pollution (Jung et al. Citation2018), as well as, synthesis of ultrafine particles with precise control of size, morphology, and chemical composition (Mädler et al. Citation2002).
The most common instrument used in aerosol research laboratories is an SMPS (scanning mobility particle spectrometer). To measure the particle size distribution, first the particles are given a known charge distribution through a neutralizer, these particles are then classified based on their electrical mobility, usually using a long/nano DMA; and then these particles are counted using a condensational particle counter (CPC). A conventional SMPS works fairly well for measurement of particles with sizes above 3 nm, but it is difficult to measure particles smaller than 3 nm, as all three major components of an SMPS: neutralizer, classifier-DMA, and CPC have their limitations for such small particles (Biswas, Wang, and Attoui Citation2018).
Several measurement tools have been developed to investigate sub-3 nm particles in the atmosphere, combustion sources, and aerosol reactors (Fernández de la Mora Citation2011; Junninen et al. Citation2010). For the measurement of particle size distribution, different types of state-of-the-art differential mobility analyzers have been developed which can classify particles as small as 1 nm in mobility diameter with high resolution (Fernández de la Mora Citation2017; Fernández de la Mora and Kozlowski Citation2013; Jiang, Attoui, et al. Citation2011). Once classified, these particles can be counted using a CPC by growing them through condensation of super-saturated vapors (Stolzenburg and McMurry Citation1991). Another approach to count the particles is to measure total current from the charged particles using an electrometer. An electrometer can only detect charged particles, and requires fairly high particle number concentration for accurate measurements, as they are limited by the minimum current that they can detect. Moreover, if there are charged particles of opposite polarity are present, the electrometer will report an erroneous value of the concentration, as it reports total current. In contrast, CPCs are highly sensitive and have high signal-to-noise ratio, as they count individual particles, and can count neutral as well as charged particles. The major limitation of the CPC is the detection limit vis a vis the minimum particle size.
In order to extend the detection limit of CPCs, several modifications to conventional designs have been proposed, such as use of different working fluids, two-stage growth with a ‘booster stage’, and others. In a typical CPC, butanol (Stolzenburg and McMurry Citation1991) or water (Hering et al. Citation2005) are used as the working fluids. As illustrated by the Kelvin equation, the use of a high surface tension fluid can help lower the minimum size detection limit of the CPCs (Magnusson et al. Citation2003). Iida, Stolzenburg, and McMurry (Citation2009) proposed that this detection limit can be lowered by using a two-stage CPC, where in first stage, particle activation and growth takes place, and in second stage, particles grow further so that they can be detected by optical scattering. It was found that particles below 2 nm can be activated and measured using diethylene glycol (DEG), and oleic acid as the working fluid for the first ‘booster stage’. Jiang, Attoui, et al. (Citation2011) combined a 2-stage DEG/butanol CPC with TSI DMA 3085 to measure the particle size distribution of particles down to 1 nm in mobility diameter. Another instrument was developed using the same 2-stage concept, but without DMA, using Airmodus PSM, which can measure particle size distribution down to 1 nm (Kangasluoma et al. Citation2013). In different experimental settings, these two instruments have been deployed to measure sub-3 nm particles of different materials like ammonium sulfate, sodium chloride, silver, tungsten oxide, etc. In these studies (Kangasluoma et al. Citation2013, Jiang, Attoui, et al. Citation2011), the activation efficiency of these particles was found to be chemistry dependent. It was concluded that sound characterization of particle source and particle counter with respect to a classifier and electrometer set-up is essential for precise measurement of sub-3 nm particle size distribution.
In contrast, several researchers have instead focused on the existing CPCs with Stolzenburg’s desgin, with a goal to measure sub-3 nm particles. In this approach, two parameters, super-saturation and residence time, are adjusted by changing saturator/condenser temperatures, and capillary aerosol flow rate in order to promote sub-3 nm particle/clusters activation, and detection by CPCs. Kuang et al. Citation2012 modified TSI CPC 3025 A by changing the saturator temperature from 37 °C to 44 °C, and capillary flow rate from 0.30 to 0.47 lpm, and measured 2 nm particles with 20–50% detection efficiency. Kangasluoma et al. (Citation2014) performed a detailed study by deploying a nano-CPC battery to compare four different types of CPCs, which were calibrated with seven different types of materials using three different working fluids, butanol, water, and DEG. It was concluded that different material is activated differently depending on the working fluid, and the activation efficiency is dependent on both material of the particle, as well as, the particle size. Very recently, it was reported that butanol is less chemistry dependent as compared to DEG, and particles as small as 1 nm can be detected using a butanol CPC Model 3776 in boosted conditions, but the detection efficiency was not quantified (Attoui Citation2018; Attoui and Kangasluoma Citation2019). Barmpounis et al. (Citation2018) showed that instead of increasing the saturator temperature, decreasing the condenser temperature can promote higher activation of sub-3 nm particles. It is important to note the difference between the 2-stage CPC, where a ‘booster stage’ is used, and 1-stage butanol based CPC in ‘boosted conditions’. 2-step CPC involves the first step to activate the particles, typically using DEG, and then second step to grow these particles so that the optical detectors can detect them in the CPC. On the other hand, using ultrafine butanol based CPC in ‘boosted conditions’ is done by changing the parameters in the CPC, like saturator temperature, condenser temperature, and capillary flow rates, so that it can detect sub-3 nm particles.
Therefore, it is important to calibrate the CPC with respect its operating conditions, as well as, particle material in order to achieve precise measurements. Recently, several researchers studying combustion systems have shown interest in the early stages (sub-3 nm) of particle formation and growth, for both the desirable (Sharma, Dhawan, et al. Citation2019; Wang et al. Citation2017a, Citation2017b; Wang, Sharma, et al. Citation2017) and undesirable particles (Carbone, Attoui, and Gomez Citation2016; Tang et al. Citation2017). To the best of our knowledge, CPCs in boosted conditions are not calibrated for flame-generated particles. In the absence of proper guidelines for the operation of boosted CPC, several researchers, in spite of the availability of butanol based CPCs, are unable to perform sub-3 nm particle measurements.
The focus of this work is to measure sub-3 nm flame-generated charged particles by using a butanol based CPC 3776, and report its detection efficiency. First, standard mobility ions of alkyl ammonium halides are generated using an electrospray to narrow down the optimal operating conditions for the butanol based CPC. Following this, combustion generated sub-3 nm particles are measured for both soot, using a McKenna burner, and titanium dioxide using a flame aerosol reactor. The effect of polarity of the particles, material properties, and operating conditions on the detection efficiency of the particles is compared.
2. Experimental set-up
shows the experimental set-up to characterize the detection efficiency of butanol CPC in boosted conditions with respect to an electrometer. This set-up has been widely used in previous works by several researchers (Attoui Citation2018; Barmpounis et al. Citation2018; Kangasluoma et al. Citation2014). First, particles/clusters were generated using different particle generation approaches, followed by particle classification using a high resolution Half-mini DMA. These size-classified particles were then measured by an electrometer and a butanol CPC in boosted conditions to calculate the particle detection efficiency.
2.1. Particle generation
The aerosol particles were generated using three different approaches. First, electrospray was used to generate alkyl ammonium halide ions. The solutions for electrospray were prepared using four different mobility standards, tetra-heptyl ammonium bromide (THAB), tetra-butyl ammonium iodide (TBAI), tetra-methyl ammonium iodide (TMAI), tetra-dodecyl ammonium bromide (TDDAB) (Ude and Fernandez de la Mora Citation2005). All these salts were purchased from Sigma Aldrich. The electrospray was operated with constant flow of air in the electrospray chamber, and the liquid was pumped by applying a constant pressure difference. Self-charged particles/clusters were generated through the capillary needle maintained at a voltage of 3 kV.
Flame aerosol reactor was used to synthesize titanium dioxide (TiO2) nanoparticles of controlled size distribution. A bypass flow of N2 was passed through a bubbler containing titanium isopropoxide (TTIP, Sigma-Aldrich Inc., >97%) at a stable temperature of 20 °C. Subsequently, this mixture was fed to the flame, where TiO2 is produced through thermal decomposition, hydrolysis, and combustion of TTIP. The bypass flow is tuned (2–6 lpm) to get the desired particle size distribution ranging from mobility diameter as small as 1.2 nm to particles as large as 5 nm in mobility diameter (Sharma, Dhawan, et al. Citation2019; Wang et al. Citation2017b). Blank flame ions were generated in the absence of any precursors, for both positive and negative polarity (Wang et al. Citation2020).
Soot particles are generated by a commercial McKenna burner with a stainless steel outer layer and 60-mm diameter bronze porous sintering plug. A shroud nitrogen gas, at 25–30 cm/s, shields the burner-stabilized-stagnation flame from surrounding air (Tang et al. Citation2017). A stainless steel sampling tube with a 0.16 mm sampling orifice is embedded in the stagnation plate. Soot particles are drawn into the sampling tube through the orifice and their particle size distribution is controlled by adjusting the flame equivalence ratio. The dilution sampling system consists of a stainless steel sampling tube with a 0.16 mm sampling orifice embedded in the stagnation plate on the burner. TiO2 and soot particles were drawn into the sampling tube through the orifice and diluted immediately by a 30 L/min nitrogen flow in the tube (Wang et al. Citation2020).
2.2. Particle measurement
The generated particles are classified using a closed-loop half-mini DMA, and are measured using an electrometer, and butanol CPC from TSI (Model 3776) in boosted conditions. The half-mini DMA used in this study was a cylindrical shape DMA (p-model), with long bullet of radius 0.4 cm, and outer cylinder of radius 0.7 cm. This p-model half-mini DMA was manufactured by Seadm (Fernández de la Mora Citation2017). Half-mini DMA was operated at high sheath flow rate (∼250 lpm) to classify particles based on their mobility, and was calibrated using THAB salt ions (Fernández de la Mora Citation2017; Fernández de la Mora and Kozlowski Citation2013; Zhang, Sharma, Wang, et al. Citation2018). A brief description of calibration and the resolution of half-mini DMA used in this work is included in the online supplementary information (SI). The classified particles were distributed in two parallel streams to electrometer and butanol CPC in boosted conditions. The electrometer (TSI 3068B) measured the current generated by the charged particles, and can be easily converted to the number concentration, which is used as the concentration reference. Electrometer inlet flow rate is maintained at 6–9 lpm depending on the experiment. The other stream to butanol CPC in boosted conditions (TSI 3776) measures the particle number concentration detected by the laser in optics section.
The flow rate entering the CPC is 1.5 lpm, of which 1.2 lpm is bypassed and the balance goes to the optics region. It is controlled by a critical orifice of fixed flow rate of 0.3 lpm, due to the operation constraint of nozzle design in optics region. In the default settings of the CPC, the sheath flow rate in the condenser is 250 sccm, and the capillary flow rate is 50 sccm. The sheath flow ensures that the particles are not lost due to radial diffusion in the condenser region. The variable orifice in line with the sheath flow allows the user to modify the capillary flow rate in the range from 30 to 70 sccm, and this flow is displayed on the main screen of butanol CPC.
The detection efficiency () of the CPC (%) is calculated as the ratio of the measured number concentration by butanol CPC in boosted conditions (
) to the reference concentration from electrometer (
), and is given by:
Here, and
denotes the diffusion losses in the sampling line for the two instruments.
It is important to note that the detection efficiency of the CPC is calculated through experiments, but is not the same as the activation efficiency of the particles. The detection efficiency includes both the activation of the particles as well as their diffusion loss in the capillary or tubing, and is given as the product of the two efficiencies.
Where, is the overall detection efficiency of the particles, calculated through direct experiments in this work,
is the activation efficiency of the particles which is promoted by high super-saturation, and
is the diffusive transmission efficiency of the particles, which can be reduced by reducing the residence time and preventing the diffusive losses.
2.3. Experimental plan
summarizes the plan for experiments for particles of different materials at different CPC operating conditions. First, homogeneous nucleation of butanol vapors is quantified as a function of boosted conditions for the CPC. Then, the optimal operating conditions for maximum detection efficiencies with minimal background from homogeneous nucleation are determined. This is followed by evaluating the effect of polarity, material properties on the detection efficiency of the butanol CPC in boosted conditions.
Table 1. Test plan for the experiments for different particles at different CPC operating conditions.
3. Results and discussion
3.1. Nucleation of butanol vapors
We initially measured the particles generated by homogenous nucleation of butanol vapors for different operating conditions of the butanol CPC. To assess the range of operating conditions that can be considered for our experiments, filtered dry air with zero humidity, and no particles was used at the inlet of the CPC. shows the measured number concentration for the filtered air at different saturator temperatures (39–45 °C) and capillary flow rates (30–70 sccm). It was found that the increase in saturator temperature from 39 to 45 °C increased the butanol nucleation as higher concentration of particles was detected. This is consistent with previous findings that as the difference between saturator temperature and the condenser temperature increases, the super-saturation increases, which promotes homogenous nucleation (Barmpounis et al. Citation2018). Moreover, increasing the capillary flow rate decreases the homogeneous nucleation due to lower residence time in the supersaturated region and lower saturation ratio (S) because the mixing ratio of the butanol saturated flow decreases. A value of 10 #/cm3 was considered as an acceptable homogeneous nucleation rate in this study, as shown in (Attoui Citation2018). Therefore, all the CPC operating conditions for less than 10 #/cm3 can be considered for the detection of particles from different aerosol generators. The value of 10 #/cm3 is a arbitrarily chosen as an acceptable limit in this work, in relation to the measured concentrations. Moreover, the saturation ratio is expected to decrease even further, when the particles are present as they take the vapors to grow; which will decrease the overall background. This background cannot be used, if the CPC is used with an inversion code in the SMPS where the inversion code is done with 0 #/cm3 threshold. As per user requirement for the % error, the level is acceptable when the CPC is used with the DMA if the concentrations coming from the DMA are high enough, relative to the background.
3.2. Effect of saturator temperature and capillary flow rate
shows the effect of increasing saturator temperature on the measurement of THAB salt ions for butanol CPC in boosted conditions in comparison with the electrometer. In these experiments, the capillary flow rate was kept fixed at the default setting of 50 sccm. Three different peaks in corresponds to THA+, (THAB)THA+, and (THAB)2THA+ with mobility sizes of 1.47, 1.78, and 1.97 nm, respectively (Ude and Fernandez de la Mora Citation2005). It was found that increasing the saturator temperature increases the CPC signal for all the three ions due to higher super-saturation. It is important to note here that even though the saturator temperature of 43 °C and above shows higher signal for CPC than lower saturator temperatures, the homogenous nucleation could influence the background more here, as highlighted in . Nevertheless, detection of sub-3 nm particles is observed in boosted conditions as compared to the default CPC settings.
Figure 3. Effect of increasing saturator temperature on the measurement of THAB salt ions for butanol CPC in boosted conditions in comparison with an electrometer. Capillary flow rate is kept fixed at 50 sccm.
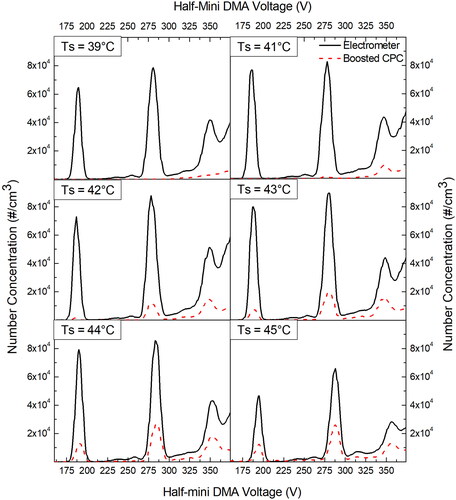
The particle number concentration measured from the butanol CPC in boosted conditions can be compared to the calculated number concentration from the electrometer current signal to get the CPC detection efficiency. shows the detection efficiency as a function of capillary flow rate at different saturator temperatures for THA+ ions. We found that as the saturator temperature was increased or the capillary flow was decreased, the CPC detection efficiency increased. consists of both the acceptable and unacceptable regions for the operation of butanol CPC in boosted conditions due to homogeneous nucleation. In the acceptable region, high saturator temperature of 44 °C and 45 °C, at high capillary flow rate of 60 and 70 sccm were found to have highest detection efficiency. Therefore, for further experiments, only three sets of operating conditions () for butanol CPC in boosted conditions were tested viz. Ts = 45 °C, f = 70 sccm; Ts = 44 °C, f = 70 sccm; and Ts = 44 °C, f = 60 sccm. It is important to note that the optimal settings for the instrument is based on the best signal with the least background noise, where the acceptable blank background noise should not be greater than 10 #/cm3.
Figure 4. Effect of capillary flow rate on the CPC Detection Efficiency (%) at different saturator temperature for the measurement of THA + ions.
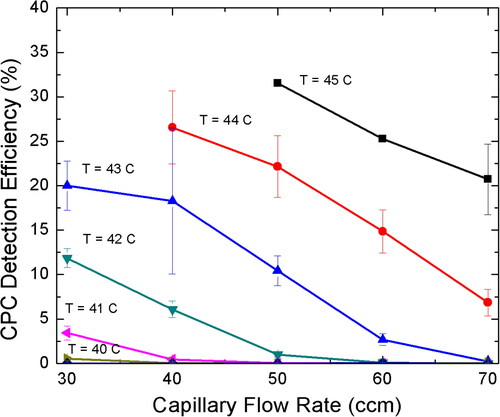
The overall detection efficiency depends on both the activation efficiency (), as well as, the penetration efficiency (
) of these ions/particles. High super-saturation due to high saturator temperature and high residence time due to low capillary flow rates increased the activation efficiency of these ions (
). On the other hand, low residence time due to high capillary flow reduces diffusion losses, and increases the diffusive transmission (
). A balance between the two parameters dictates the overall detection efficiency.
Residence time in the capillary region significantly influences the particle penetration efficiency. For instance, at a capillary flow rate of 30 sccm, 50% of 2 nm particles are lost in the capillary (Stolzenburg and McMurry Citation1991). Even at a capillary flow rate of 70 sccm, which is the highest that can be achieved in the existing design of the TSI CPC 3776, as many as 60% of 1 nm particles are lost (Attoui Citation2018; Friedlander Citation2000) before the activation process. Moreover, increasing the capillary flow rate decreased the sheath flow, which can lead to higher radial diffusion loss in the condenser region. In order to resolve this limitation, nozzle in the optical head needs to be designed to accommodate higher flow rates than 0.3 lpm, a longer condenser should be considered.
shows that the CPC detection efficiency increases as the mobility diameter increases. The monomer and dimer ions of alkyl ammonium halide ions were considered for the calculation of the CPC detection efficiency at three different CPC conditions. The reader is referred to for the different salt ions considered here. The detection efficiency was found to increase with increase in particle size due to ease of activation, as well as, high penetration of relatively larger particles. The best detection efficiency for all the particle sizes considered here was found for high capillary flow of 70 sccm and saturator temperature of 45 °C. Even though, it has been previously reported that increasing the capillary flow beyond 60 sccm could adversely affect the particle transmission due to lower sheath flow contributing to radial losses (Attoui Citation2018), but in this work, we found that higher capillary flow rate continues to increase the detection efficiency.
3.3. Effect of particle material
shows the CPC detection efficiency for two different flame generated particles of both the polarities. The butanol CPC in boosted conditions is operated at the capillary flow rate of 70 sccm and saturator temperature of 45 °C to ensure high detection efficiency. The particle size distribution for charged titanium dioxide and soot particles begins at 1.2 and 1.5 nm of mobility diameter, respectively. For the details on raw number concentrations measured by the electrometer and CPC in boosted conditions, the reader is referred to supplementary information Figure S2 in the SI. It was found that the CPC detection efficiency follows a typical ‘S-curve’ for both the flame generated particles, with ∼95% detection for 3 nm particles. The negatively charged particles activated slightly more than positively charged particles, especially for below 2 nm particles, probably due to butanol vapors preferential condensation on the former. Similar trends for polarity dependence have also been reported previously with tungsten oxide, sodium chloride and silver particles (Kangasluoma et al. Citation2013, Citation2014). The possible reasoning behind this difference due to polarity can be explained by quantum chemistry calculations, which is outside the scope of this work (Kangasluoma and Attoui Citation2019).
Figure 6. CPC Detection Efficiency (%) for titanium dioxide (TiO2) synthesized using a flame aerosol reactor, and soot particles synthesized using a McKenna burner for both polarities.
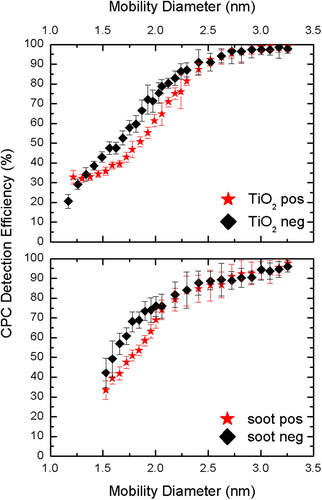
shows the comparison of the detection efficiency of positively charged particles composed of different materials from different aerosol generators. The CPC was operated at the capillary flow rate of 70 sccm and saturator temperature of 45 °C for all the particles. For the particles larger than 1.6 nm in mobility size, the CPC detection efficiency was found to be similar for alkyl ammonium halide ions, titania, and soot particles. In contrast, for particles smaller than 1.6 nm in mobility diameter, there were differences in the detection efficiencies of titanium dioxide, flame generated ions without any precursor, and alkyl ammonium halide salt ions. Alkyl ammonium halide ions, which are considered relatively difficult to activate due to less solubility in water were found to be least activated. On the other hand, titanium dioxide particles and flame-generated organic ions which are readily soluble in water were found to activate more easily. For more details on the particle size distributions, the reader is referred to the SI (Figure S3).
Figure 7. CPC Detection Efficiency (%) for four different types of positively charged particles/ions (<2 nm) as a function of their mobility diameter. Here, the saturator temperature is 45 °C and aerosol capillary flow rate is 70 sccm.
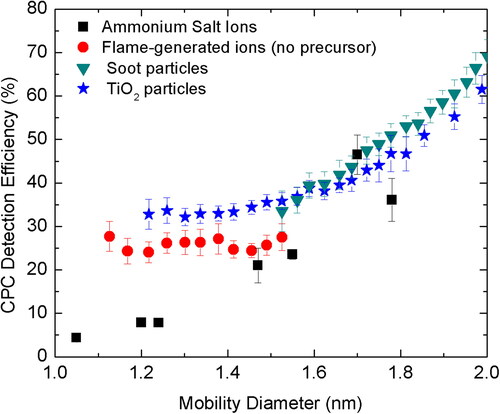
This clearly shows that butanol is not very chemistry dependent, especially for particles larger than 1.6 nm in mobility diameter. This has also been reported previously when candle generated particles, tungsten oxide, etc. were considered (Kangasluoma et al. Citation2014). While DEG is used in the 1 nm SMPS which involves a 2-stage CPC, it is reported to be more chemistry dependent than butanol (Kangasluoma, Ahonen, et al. Citation2015; Kangasluoma, Ahonen, et al. Citation2015; Kangasluoma et al. Citation2014). Nevertheless, proper calibration is quintessential for both butanol and DEG based CPCs to ensure accurate measurement of the particle size distribution for below 3 nm charged particles. As ultrafine design of butanol based CPCs are readily available as an ‘existing’ instrument in aerosol laboratories, the user can make some reversible changes in the operating conditions of the CPC to successfully measure sub-3 nm particles with minimal background.
4. Conclusions
The detection efficiency of a butanol CPC in boosted conditions has been systematically characterized for sub-3 nm alkyl ammonium halide ions and flame-generated particles. Optimal conditions for the operation of butanol CPC in boosted conditions are determined under the assumption that the acceptable homogenous nucleation limit is 10 #/cm3. The maximum detection efficiency for sub-3 nm particles is achieved at a saturator temperature of 45 °C and capillary flow rate of 70 sccm, with background homogeneous nucleation rate of <5 #/cc. Flame-generated particles, as small as 1.2 nm in mobility diameter for titanium dioxide, and 1.5 nm for soot were found to be activated at these optimal operating conditions. The negatively charged particles were activated slightly more than the positively charged due to difference in the interactive forces with the butanol vapors. Particles of different materials larger than 1.6 nm are activated with similar efficiency, whereas particles below 1.6 nm are dominated by their composition. This establishes the applicability of a butanol CPC under boosted modality for the measurement of sub-3 nm particles, but at the same time, reinforces the importance of appropriate calibration for their precise measurement. The researchers with existing ultrafine butanol CPCs can perform sub-3 nm measurements to study early stages of particle formation and growth in different aerosol reactors.
Supplemental Material
Download MS Word (1.7 MB)Acknowledgments
Dr. Juan Fernandez de la Mora is gratefully acknowledged for assistance with the setting up of the new half-mini DMA set-up.
Additional information
Funding
References
- Attoui, M. 2018. Activation of sub 2 nm singly charged particles with butanol vapors in a boosted 3776 TSI CPC. J. Aerosol Sci. 126:47–57. doi:https://doi.org/10.1016/j.jaerosci.2018.08.005.
- Attoui, M., and J. Kangasluoma. 2019. Activation of sub 2 nm water soluble and insoluble standard ions with saturated vapors of butanol in a boosted TSI ultrafine CPC. Atmosphere 10 (11):665. doi:https://doi.org/10.3390/atmos10110665.
- Barmpounis, K., A. Ranjithkumar, A. Schmidt-Ott, M. Attoui, and G. Biskos. 2018. Enhancing the detection efficiency of condensation particle counters for sub-2 nm particles. J. Aerosol Sci. 117:44–53. doi:https://doi.org/10.1016/j.jaerosci.2017.12.005.
- Biswas, P., Y. Wang, and M. Attoui. 2018. Sub-2nm particle measurement in high-temperature aerosol reactors: a review. Curr. Opin. Chem. Eng. 21:60–6. doi:https://doi.org/10.1016/j.coche.2018.03.004.
- Carbone, F., M. Attoui, and A. Gomez. 2016. Challenges of measuring nascent soot in flames as evidenced by high-resolution differential mobility analysis. Aerosol Sci. Technol. 50 (7):740–57. doi:https://doi.org/10.1080/02786826.2016.1179715.
- Fernández de la Mora, J. 2011. Sub-3 nm aerosol measurement with DMAs and CNCs, 697–722. New York: John Wiley & Sons.
- Fernandez de la Mora, J. 2017. Expanded flow rate range of high-resolution nanoDMAs via improved sample flow injection at the aerosol inlet slit. J. Aerosol Sci. 113:265–75. doi:https://doi.org/10.1016/j.jaerosci.2017.07.020.
- Fernandez de la Mora, J., and J. Kozlowski. 2013. Hand-held differential mobility analyzers of high resolution for 1–30 nm particles: Design and fabrication considerations. J. Aerosol Sci. 57:45–53. doi:https://doi.org/10.1016/j.jaerosci.2012.10.009.
- Friedlander, S. K. 2000. Smoke, dust, and haze: Fundamentals of aerosol dynamics. In Topics in chemical engineering. New York: Oxford University Press.
- Gentner, D. R., G. Isaacman, D. R. Worton, A. W. H. Chan, T. R. Dallmann, L. Davis, S. Liu, D. A. Day, L. M. Russell, K. R. Wilson, et al. 2012. Elucidating secondary organic aerosol from diesel and gasoline vehicles through detailed characterization of organic carbon emissions. Proc. Natl. Acad. Sci. USA. 109 (45):18318–23. doi:https://doi.org/10.1073/pnas.1212272109.
- Guo, S., M. Hu, M. L. Zamora, J. Peng, D. Shang, J. Zheng, Z. Du, Z. Wu, M. Shao, L. Zeng, et al. 2014. Elucidating severe urban haze formation in China. Proc. Natl. Acad. Sci. USA. 111 (49):17373–8. doi:https://doi.org/10.1073/pnas.1419604111.
- Hering, S. V., M. R. Stolzenburg, F. R. Quant, D. R. Oberreit, and P. B. Keady. 2005. A laminar-flow, water-based condensation particle counter (WCPC). Aerosol Sci. Technol. 39 (7):659–72. doi:https://doi.org/10.1080/02786820500182123.
- Iida, K., M. R. Stolzenburg, and P. H. McMurry. 2009. Effect of working fluid on sub-2 nm particle detection with a laminar flow ultrafine condensation particle counter. Aerosol Sci. Technol. 43 (1):81–96. doi:https://doi.org/10.1080/02786820802488194.
- Jiang, J., M. Attoui, M. Heim, N. A. Brunelli, P. H. McMurry, G. Kasper, R. C. Flagan, K. Giapis, and G. Mouret. 2011. Transfer functions and penetrations of five differential mobility analyzers for sub-2 nm particle classification. Aerosol Sci. Technol. 45 (4):480–92. doi:https://doi.org/10.1080/02786826.2010.546819.
- Jiang, J., M. Chen, C. Kuang, M. Attoui, and P. H. McMurry. 2011. Electrical mobility spectrometer using a diethylene glycol condensation particle counter for measurement of aerosol size distributions down to 1 nm. Aerosol Sci. Technol. 45 (4):510–21. doi:https://doi.org/10.1080/02786826.2010.547538.
- Jung, S., J. Fang, T. S. Chadha, and P. Biswas. 2018. Atmospheric pressure plasma corona enhanced by photoionizer for degradation of VOCs. J. Phys. D: Appl. Phys. 51 (44):445206. doi:https://doi.org/10.1088/1361-6463/aae133.
- Junninen, H., M. Ehn, T. Petäjä, L. Luosujärvi, T. Kotiaho, R. Kostiainen, U. Rohner, M. Gonin, K. Fuhrer, M. Kulmala, et al. 2010. A high-resolution mass spectrometer to measure atmospheric ion composition. Atmos. Meas. Tech. 3 (4):1039–53. doi:https://doi.org/10.5194/amt-3-1039-2010.
- Kangasluoma, J., L. Ahonen, M. Attoui, H. Vuollekoski, M. Kulmala, and T. Petäjä. 2015. Sub-3 nm particle detection with commercial TSI 3772 and Airmodus A20 fine condensation particle counters. Aerosol Sci. Technol. 49 (8):674–81. doi:https://doi.org/10.1080/02786826.2015.1058481.
- Kangasluoma, J., and M. Attoui. 2019. Review of sub-3 nm condensation particle counters, calibrations, and cluster generation methods. Aerosol Sci. Technol. 53 (11):1–34. doi:https://doi.org/10.1080/02786826.2019.1654084.
- Kangasluoma, J., M. Attoui, H. Junninen, K. Lehtipalo, A. Samodurov, F. Korhonen, N. Sarnela, A. Schmidt-Ott, D. Worsnop, M. Kulmala, et al. 2015. Sizing of neutral sub 3 nm tungsten oxide clusters using airmodus particle size magnifier. J. Aerosol Sci. 87:53–62. doi:https://doi.org/10.1016/j.jaerosci.2015.05.007.
- Kangasluoma, J., H. Junninen, K. Lehtipalo, J. Mikkilä, J. Vanhanen, M. Attoui, M. Sipilä, D. Worsnop, M. Kulmala, and T. Petäjä. 2013. Remarks on ion generation for CPC detection efficiency studies in sub-3-nm size range. Aerosol Sci. Technol. 47 (5):556–63. doi:https://doi.org/10.1080/02786826.2013.773393.
- Kangasluoma, J., C. Kuang, D. Wimmer, M. P. Rissanen, K. Lehtipalo, M. Ehn, D. R. Worsnop, J. Wang, M. Kulmala, and T. Petäjä. 2014. Sub-3 nm particle size and composition dependent response of a nano-CPC battery. Atmos. Meas. Tech. 7 (3):689–700. doi:https://doi.org/10.5194/amt-7-689-2014.
- Kruis, F. E., H. Fissan, and A. Peled. 1998. Synthesis of nanoparticles in the gas phase for electronic, optical and magnetic applications—A review. J. Aerosol Sci. 29 (5-6):511–35. doi:https://doi.org/10.1016/S0021-8502(97)10032-5.
- Kuang, C., M. Chen, P. H. McMurry, and J. Wang. 2012. Modification of laminar flow ultrafine condensation particle counters for the enhanced detection of 1 nm condensation nuclei. Aerosol Sci. Technol. 46 (3):309–15. doi:https://doi.org/10.1080/02786826.2011.626815.
- Kulmala, M., I. Riipinen, M. Sipilä, H. E. Manninen, T. Petäjä, H. Junninen, M. D. Maso, G. Mordas, A. Mirme, M. Vana, et al. 2007. Toward direct measurement of atmospheric nucleation. Science 318 (5847):89–92. doi:https://doi.org/10.1126/science.1144124.
- Li, S., Y. Ren, P. Biswas, and D. T. Stephen. 2016. Flame aerosol synthesis of nanostructured materials and functional devices: Processing, modeling, and diagnostics. Prog. Energy Combust. Sci. 55:1–59. doi:https://doi.org/10.1016/j.pecs.2016.04.002.
- Mädler, L., H. K. Kammler, R. Mueller, and S. E. Pratsinis. 2002. Controlled synthesis of nanostructured particles by flame spray pyrolysis. J. Aerosol Sci. 33 (2):369–89. doi:https://doi.org/10.1016/S0021-8502(01)00159-8.
- Magnusson, L.-E., J. A. Koropchak, M. P. Anisimov, V. M. Poznjakovskiy, and J. F. de la Mora. 2003. Correlations for vapor nucleating critical embryo parameters. J. Phys. Chem. Ref. Data. 32 (4):1387–410. doi:https://doi.org/10.1063/1.1555590.
- Sharma, G., S. Dhawan, N. Reed, R. Chakrabarty, and P. Biswas. 2019. Collisional growth rate and correction factor for TiO2 nanoparticles at high temperatures in free molecular regime. J. Aerosol Sci. 127:27–37. doi:https://doi.org/10.1016/j.jaerosci.2018.10.002.
- Stolzenburg, M. R., and P. H. McMurry. 1991. An ultrafine aerosol condensation nucleus counter. Aerosol Sci. Technol. 14 (1):48–65. doi:https://doi.org/10.1080/02786829108959470.
- Strobel, R., and S. E. Pratsinis. 2007. Flame aerosol synthesis of smart nanostructured materials. J. Mater. Chem. 17 (45):4743–56. doi:https://doi.org/10.1039/b711652g.
- Swihart, M. T. 2003. Vapor-phase synthesis of nanoparticles. Current Opinion in Colloid & Interface Science. 8 (1):127–33. doi:https://doi.org/10.1016/S1359-0294(03)00007-4.
- Tang, Q., R. Cai, X. You, and J. Jiang. 2017. Nascent soot particle size distributions down to 1 nm from a laminar premixed burner-stabilized stagnation ethylene flame. Proc. Combust. Inst. 36 (1):993–1000. doi:https://doi.org/10.1016/j.proci.2016.08.085.
- Ude, S., and J. F. Fernandez de la Mora. 2005. Molecular monodisperse mobility and mass standards from electrosprays of tetra-alkyl ammonium halides. J. Aerosol Sci. 36 (10):1224–37. doi:https://doi.org/10.1016/j.jaerosci.2005.02.009.
- Wang, M., G. Sharma, H. Zhang, X. You, and P. Biswas. 2020. Charge characteristics of sub-10 nm soot particles in premixed ethylene flames. Fuel 279:118511. doi:https://doi.org/10.1016/j.fuel.2020.118511.
- Wang, X., B. J. Williams, X. Wang, Y. Tang, Y. Huang, L. Kong, X. Yang, and P. Biswas. 2013. Characterization of organic aerosol produced during pulverized coal combustion in a drop tube furnace. Atmos. Chem. Phys. 13 (21):10919–32. doi:https://doi.org/10.5194/acp-13-10919-2013.
- Wang, Y., J. Kangasluoma, M. Attoui, J. Fang, H. Junninen, M. Kulmala, T. Petäjä, and P. Biswas. 2017a. The high charge fraction of flame-generated particles in the size range below 3nm measured by enhanced particle detectors. Combust. Flame. 176:72–80. doi:https://doi.org/10.1016/j.combustflame.2016.10.003.
- Wang, Y., J. Kangasluoma, M. Attoui, J. Fang, H. Junninen, M. Kulmala, T. Petäjä, and P. Biswas. 2017b. Observation of incipient particle formation during flame synthesis by tandem differential mobility analysis-mass spectrometry (DMA-MS). Proc. Combust. Inst. 36 (1):745–52. doi:https://doi.org/10.1016/j.proci.2016.07.005.
- Wang, Y., G. Sharma, C. Koh, V. Kumar, R. Chakrabarty, and P. Biswas. 2017. Influence of flame-generated ions on the simultaneous charging and coagulation of nanoparticles during combustion. Aerosol Sci. Technol. 51 (7):833–44. doi:https://doi.org/10.1080/02786826.2017.1304635.
- Zhang, H., G. Sharma, Y. Wang, S. Li, and P. Biswas. 2018. Numerical modeling of the performance of high flow DMAs to classify sub-2 nm particles. Aerosol Sci. Technol. 53 (1):106–118.