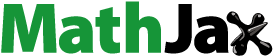
Abstract
Hygroscopicity is one of the most important physicochemical properties for nanoparticle science and engineering. Hygroscopicity determines the ability of a particle to swell or contract in the presence of water vapor. The hygroscopicity for organic aerosol is a known function of the solute molar volume. This is particularly true for low-molecular weight (<200 g mol−1) organic compounds used for drug delivery. In this work, four commonly used biodegradable hydrophilic compounds, mannitol, lactose, gelatin and polyethylene glycol are measured with a fast and unique hygroscopicity-cloud condensation nuclei activation technique. Flory-Huggins Köhler theory is used to estimate the single parameter hygroscopicity. Results show that for the high molecular weight compounds, a water-polymer interaction parameter must also be considered and the water-polymer interaction parameter for nanoparticles can be determined in the supersaturated regime. The water affinity becomes essential to nanoparticle hygroscopicity, and the organic molar volume contribution starts to decrease as the molecular weight increases. Furthermore, we provide a new size-dependent parameterization for hygroscopicity of polymers. In summary, the water affinity of the interaction parameter can be measured for nano-particles and plays an important role of the water uptake of organic nano-particles.
Copyright © 2021 American Association for Aerosol Research
EDITOR:
1. Introduction
Organic aerosol is ubiquitous and regularly inhaled by the human body. Indeed, organic particles can contribute up to 30%–80% of the atmospheric aerosol mass burden (Zhang et al. Citation2007) including short dicarboxylic acids to long polymeric chains (Kalberer et al. Citation2004). Additionally, organic nano-particles are intentionally produced and inhaled for aerosol therapy (Muralidharan et al. Citation2015; Azarmi, Roa, and Löbenberg Citation2008; Gill et al. Citation2007; Agnihotri, Mallikarjuna, and Aminabhavi Citation2004; Malcolmson and Embleton Citation1998). The health effects of inhaled particles depend on the deposition in the respiratory system; the final particle deposition is controlled by particle size (Gill et al. Citation2007; Malcolmson and Embleton Citation1998). These particles might not stay at nano-size but likely up-take water and grow to larger particles after exposure to high relative humidity in the respiratory system (Kim, Xi, and Si Citation2013; Shelly, Lloyd, and Park Citation1988). Larger particles have greater gravitational settling and impaction; thus affecting the drug delivery efficiency or potentially causing unwanted health effects (Vu, Delgado-Saborit, and Harrison Citation2015; Longest and Hindle Citation2012; Longest, Tian, and Hindle Citation2011; Hindle and Longest Citation2010; Broday and Georgopoulos Citation2001). Longest and Hindle Citation2012 proposed that nanoparticle enhanced condensational growth (ECG) could reduce the number of exhaled particles and increase the deposition efficiency of drug particles in respiratory tract. Longest and Hindle Citation2012 used a non-hygroscopic drug, budesonide, and hygroscopic excipient mimics (sodium chloride, citric acid and mannitol) in the study. The ECG simulations showed that larger wet particles can be ∼ 3 to 4 times greater than the initial dry particle diameter and the ability of modeled inhaled organic nanoparticles to swell was governed by aerosol size and hygroscopicity (Longest and Hindle Citation2012). Hence an improved understanding and measurement of aerosol water-uptake is needed to predict inhaled particle deposition.
Hygroscopicity is related to but different than solubility (Sullivan et al. Citation2009). It is an important property in aerosol science, which can be used with suitable thermodynamic theory to predict aerosol droplet formation (Man et al. Citation2008) and drug efficiencies in pharmaceutical science (Anbarasan et al. Citation2018; Visalakshi et al. Citation2005). Hygroscopicity can be measured with bulk and nano-scale measurements. However, the experimental methods to measure hygroscopicity differ vastly between nano and macro scales (Tang et al. Citation2019). Bulk (macro-scale) hygroscopicity measurements require significant amounts of substance (∼milligrams) and are usually measured by gravimetric sorption methods that weigh the mass difference before and after the water sorption (Anbarasan et al. Citation2018; Weinmüller et al. Citation2006; Visalakshi et al. Citation2005). Several aerosol, particularly nanoparticle, methods to measure hygroscopicity exist and have been recently reviewed (Tang et al. Citation2019). One of the most versatile methods to estimate hygroscopicity exposes particles to supersaturated water humidities using a Cloud Condensation Nuclei counter (CCNc) [e.g. but not limited to CCN (Vu et al. Citation2019; Kumar, Nenes, and Sokolik Citation2009; Dinar, Anttila, and Rudich Citation2008; Petters and Kreidenweis Citation2007)]. Bulk and nano-scale methods produce different hygroscopicity estimates; the total surface area of nanoparticles can be larger than bulk substances and the water vapor liquid equilibrium occurs on significantly different time scales. Furthermore, as water vapor condenses on nanoparticle seeds, the concentration of solute at equilibrium could be much more dilute than the equilibrium concentration of a bulk scale experiment. Indeed, the mechanism of nano-particle enhanced condensation growth in human respiratory system parallels that of nano-particle hygroscopic growth in the atmosphere. Thus single-parameter hygroscopicity estimates proposed for atmospheric nano-particles (Petters and Kreidenweis Citation2007) may be applied to predict the final droplet size of inhaled nano-particles.
The water-soluble inorganic solutes behaves ideally in solution and the water activity of dilute droplets can be approximated with Raoult’s law and Köhler theory (Sullivan et al. Citation2009; Petters and Kreidenweis Citation2007). Hence the derived thermodynamic hygroscopicity is simply the ratio of the molecular volume of the solute to water, multiplied with the van’t Hoff disassociation coefficient (Sullivan et al. Citation2009). However, organic interactions with water can be non-ideal. The predicted hygroscopicity derived from Raoult’s-Köhler theory deviates from measurements as the molecular weight of the solute surpasses 300 g mol−1. This is particularly true for large molecular weight organic polymers (Petters et al. Citation2009).
Polymer nanoparticles are common drug delivery materials (Muralidharan et al. Citation2015; Azarmi, Roa, and Löbenberg Citation2008) and are also formed in the atmosphere (Kalberer et al. Citation2004). Thus, there exists thermodynamic theory to account for the polymeric water-uptake of nanoparticles (Petters et al. Citation2006; Petters et al. Citation2009). To account for polymer nano-particle behavior in a solvent, previous work by Petters et al. Citation2006 combined the water activity from Flory-Huggins theory with Köhler theory to predict the final wet droplet size of organic particles. Flory-Huggins theory (Flory Citation1942) assumes the interaction parameter is independent of organic volume fraction if the solvent readily dissolves the solute. Petters et al. Citation2006 applied the model of Wolf (Citation2003) into Flory Huggins-Köhler (FHK) theory, transforming the interaction parameter as a function of volume fraction and three additional fitted parameters. FHK theory (Petters et al. Citation2006) also assumes that the molar volume of the solute approaches infinity, the contribution of the molecular weight is negligible and the water polymer interaction defined by three fitted parameters dominates.
The water polymer interaction parameter has been previously measured with bulk gravimetric sorption techniques (Weinmüller et al. Citation2006; Karimi et al. Citation2005). However as with hygroscopicity measurements, the equilibrium concentration and water polymer interactions derived from bulk measurements do not account for curvature at the particle air-interface and thus assumptions at nano-scales (Tang et al. Citation2019). The equilibrium water uptake on a nanoparticle is large; the wet particle diameter can be 10 to 100 times greater than the dry particle diameter thus forming a dilute solution. Conversely, for macro-scale measurements water absorbs on the surface of the material. Visalakshi et al. Citation2005 measured the water uptake behavior of 0.1 g of different antituberculosis drugs by a gravimetric method; the water content was sub-saturated and ranged from 2% to 55% at high relative humidity (∼93%). For polymer hydrogels, Weinmüller et al. Citation2006 used water vapor sorption method with hydrogel layer 1 to 3 μm, and the sorption water activity ranged from 0.077 to 0.85. Again, Flory-Huggins theory assumes the interaction parameter is constant for very dilute solutions. Hence the Flory-Huggins theory assumption may be inadequate for bulk hygroscopic estimates but appropriate for hydrophilic substances at nano-scale.
Four common hydrophilic organic inhalable drug delivery materials are experimentally tested in this study with atmospheric CCN activation measurement and modeling techniques. Mannitol, lactose, gelatin and polyethylene glycol (PEG) have significantly different molecular weights (182, 342, ∼75,000, and ∼100,000 g mol−1, respectively; see ). The range in properties will be used to examine and derive physical meaning to the relationship of nano-particle hygroscopicity and the water-interaction parameter. Furthermore, experimental results will be compared to a combination of existing theory, explicit and empirical droplet models.
Table 1. Nano-particle properties of compounds used in this study.
2. Materials and method
Dry nanoparticles particles were generated from atomized organic solutions. Specifically, 0.01 g of mannitol (Sigma Aldrich, 99+), lactose (Sigma Aldrich, 99+), gelatin (Sigma Aldrich, Type A, BioReagent, powder, gel strength ∼300 g) , and polyethylene glycol (PEG, also known as polyethylene oxide, PEO, Sigma Aldrich, quality level 200, powder), were dissolved into 250 ml of ultra-purified water (Milipore® 18MΩ). Additional properties of these compounds are presented in . A Collison type atomizer then generated wet droplets from the solution. The droplets were subsequently heated to 100 °C via a heated coil and dried via a silicon dryer. The relative humidity after the silicon dryer has been measured and is than 5% RH. The dry poly-disperse ultrafine aerosols are then sampled at 0.8 liter per minute with a scanning mobility particle sizer (SMPS). The SMPS consists of an Electrostatic Classifier, Differential Mobility Analyzer (TSI DMA 3080) and Condensation Particle Counter (TSI CPC 3776). The DMA size selects the poly dispersed dry aerosols by electrical mobility and produces mono-dispersed aerosols. The mono-disperse aerosols were then split into the CPC and Cloud Condensation Nuclei Counter (TSI CCNC-100) (Roberts and Nenes Citation2005) with a 0.3 liter per minute and a flowrate 0.5 liter per minute flowrate, respectively, to analyze the CCN activity. The total particle concentration (CN) is counted by the CPC and the concentration of particles that form droplets at a selected size and constant supersaturation (CCN) is counted by the CCNC. The ratio of the CCN to CN is computed with Scanning Mobility CCN Analysis Method (SMCA) (Moore, Nenes, and Medina Citation2010) and a critical diameter (Dc) is computed. The CCN to CN ratio is a normalized value and particles of the same composition will activate the same regardless of number concentration. Furthermore, we generate particles to maintain good counting statistics (above 50 particles per cm3) and below limits where water depletion effects may occur (less than 10,000 particles per cm3 (Fofie, Castelluccio, and Asa-Awuku Citation2018; Lathem and Nenes Citation2011). For a given constant supersaturation, s, and compound, the experiment is repeated four times. By measuring the CCN/CN ratio at several different supersaturations, supersaturation and dry diameter (s-Dc) data is obtained. The supersaturation is then varied from 0.2% to 1.2%. (s-Dc) measurements are required to calculate nanoparticle hygroscopicity.
3. Theory and calculations
Droplet formation is a function of S and can be can be described with Köhler theory (Köhler Citation1936) as follows:
(1)
(1)
where is the water activity of the solution and D is the wet diameter of the droplet. A is a coefficient related to the droplet properties and equals
(2)
(2)
where
is the molecular weight of water,
is the gas constant,
is the temperature and
is the density of water.
is the surface tension of the droplet which is assumed to be the same as pure water. Although solute dissolves in the solution, the droplet is assumed to be dilute and have the properties of pure water.
For the simple Köhler theory (Köhler Citation1936), is approximated by Raoult’s law and is equal to the molar fraction of water,
Henceforth, we refer to this model as Raoult-Köhler (RK) theory, as introduced by Petters and Kreidenweis in Citation2007. For organic polymers in RK theory, the van’t Hoff coefficient, i = 1 and the single-parameter hygroscopicity, κ is the ratio of the molecular volume of water and the molecular volume of solute (Sullivan et al. Citation2009). If saturation, S, and particle diameter, Dc are experimentally known, κ- hygroscopicity with RK assumptions is determined from experimental data as follows (Petters and Kreidenweis Citation2007):
(3)
(3)
Flory-Huggins-Köhler (FHK) theory expands RK to account for polymer behavior (Petters et al. Citation2006, Citation2009) (see Appendix) such that:
(4)
(4)
where
is the volume fraction of the polymer,
is the reciprocal of the chain segments of the polymer equal to the ratio of the molecular volume of water and the solute and
is the Flory-Huggins interaction parameter. The interaction parameter,
is related to the solubility of the solute and solvent. The droplet will separate into two phases if the interaction parameter is larger than 0.5. The solvent is considered a good solvent if the interaction parameter is smaller than 0.5. The interaction parameter for a given solute is often unknown and it is assumed constant. Furthermore, water is assumed to dissolve the solute and is thus assumed to be a good solvent in droplet studies.
is obtained from the curve fittings of the (s-Dc) data. EquationEquation (5)
(5)
(5) is combined with the single-parameter hygroscopicity, κ (Petters and Kreidenweis Citation2007) in EquationEquation (4)
(4)
(4) , and the term for Flory-Huggins-Köhler hygroscopicity, κFHK, is as follows:
(5)
(5)
EquationEquation (6)(6)
(6) accounts for entropic and enthalpic changes of polymers in droplet solutions. Specifically, the first term in the exponent [(1-F)φ] relates to the entropic disorder of the molecules mixing and particle sizes that relate to the processes of mixing. The second term in the exponent [χφ2] is the enthalpy term and molecular interactions are represented by the interaction parameter. The above equation can be simplified (see Appendix) such that,
(6)
(6)
where
is the simplified hygroscopicity. The thermodynamic interpretation is more explicit in the simplified equation; In EquationEquation (7)
(7)
(7) , the hygroscopicity is the ratio of the molecular volume of water and the solute, adjusted by the water affinity-the interaction parameter, and is a combination of the entropy and enthalpy terms. This shows that the hygroscopicity depends not only on the molar volume now but also the molecular interaction. Notice that when
equals to zero, the ideal solution formed and the hygroscopicity is purely entropic controlled by the polymer model. For the non-polymer chemical, this entropy model may not be applicable. With the
as a degree of freedom, the deviation from an inaccurate model may be included into
and cause
to be highly negative.
If the saturation increases for a given nano-polymer, there exists a critical polymer volume fraction, at the point of droplet activation.
is determined from the derivative of saturation to volume fraction equal to zero:
(7)
(7)
Thus, the simplified, Flory-Huggins-Köhler hygroscopicity κFHK-s can be predicted from experimental parameters as follows:
(8)
(8)
If the nanoparticle solute has F → 0 then the single-parameter κ-hygroscopicity will be proportional to Thus, hygroscopicity decreases when the dry diameter increases. When the interaction parameter is 0.5, κ-hygroscopicity = F. In other words, for a polymer where F → 0 the solute does not dissolve; κ = 0 and the particle is non-hygroscopic (Sullivan et al. Citation2009). For inhaled hydrophilic biodegradable nano-particle systems, water is assumed a good solvent and thus EquationEquation (8)
(8)
(8) will be compared to experimental data to test the new model.
4. Results and discussion
shows the CCN activation data of (NH4)2SO4 and four hydrophilic biodegradable nanoparticles exposed to ∼1% supersaturation. At 50% CCN/CN efficiency, the critical activation diameter, Dc is defined. At 1% s (NH4)2SO4 activates at 25 nm. (NH4)2SO4 is inorganic, readily disassociates and is more hygroscopic than mannitol (Dc = 46 nm), lactose (Dc = 50 nm), gelatin (Dc = 74 nm), and PEG (Dc = 54 nm). A smaller Dc for a constant s indicates more hygroscopic material and thus the organic nanoparticles have larger Dc than (NH4)2SO4. In RK theory, Dc is expected to increase as the molecular weight increases (Petters et al. Citation2009). However, for PEG (100,000 g mol−1) the activation dry diameter is similar to lactose (360 g mol−1) and RK theory prediction for hygroscopicity fails.
Figure 1. The droplet activation for ammonium sulfate (red triangles, 132 g mol-1), mannitol (blue diamonds, 182 g mol−1), lactose (pink circles, 342 g mol−1), gelatin (green squares, ∼75,000 g mol−), and PEG (grey rhombus, ∼100,000 g mol−1) at ∼1% supersaturation. A sigmoidal fit (solid line) determines critical dry diameter, Dc, at CCN/CN = 0.5.
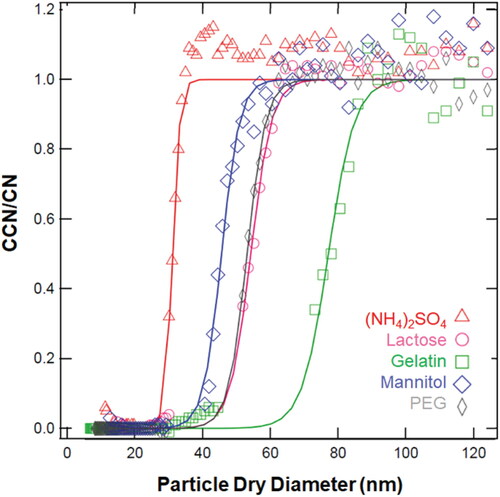
The experiments are repeated over a range of s and the s-Dc data is shown in . s-Dc is used to determine organic nano-particle κ-values derived from RK theory and FHK theory and the water-polymer interaction parameters, χ. Lower molecular weight compounds mannitol and lactose (< 500 g mol−1) are shown in and higher molecular weight polymers (gelatin and PEG) are shown in . The hydrophilic organic compounds deviate from RK theory (Petters et al. Citation2009) (). However, the RK prediction deviates more for larger molar volume molecules ( and ). FHK, assumes the nano-particle behaves as a polymer and when χ = 0, the FHK model is an improvement over RK theory for gelatin and PEG. FHK with χ = 0 is similar to RK for lactose and mannitol and underpredicts the hygroscopicity of non-polymers. The FHK model is improved by decreasing χ. A smaller implies that a smaller supersaturation with the same dry diameter is required for droplet formation and therefore greater water up-take will occur for the same amount of solute. The best fit χ for mannitol and lactose (–12 and −8.3, respectively) agree well with the non-exponential response of the s-Dc data (.).
Figure 2. The relationship between supersaturation and nanoparticle dry diameter activation and droplet growth. (s-Dc) data for ammonium sulfate (red triangles) and RK theory (solid lines) are shown for reference. Dashed lines apply FHK theory with and best fit single water-activity interaction parameter,
(a) Shows data and theoretical fits for gel-like low molecular weight non-polymers, mannitol and lactose. (b) Shows data and theoretical fits for polymers PEG and gelatin. Raoult-Köhler (RK) theory predicts a smaller activation dry diameter with a smaller molecular weight. The RK line for PEG is beyond the range of the figure. RK theory fails (not shown) for PEG with a molecular weight of 100,000 g mol-1. Note supersaturation
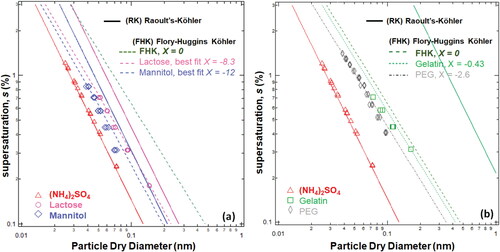
Figure 3. Nano-particle hygroscopicity as a function of molar volume. Data points are the critical activation diameters, Dc at s = 1% for lactose (pink circles), mannitol (blue triangles), PEG (grey rhombus) and Gelatin (green squares). The solid line is the RK prediction while the dashed lines are the FHK prediction for the measured Dc and best fit interaction parameter, χ. In FHK, hygroscopicity is a function of both the dry diameter and the interaction parameter (EquationEquation (6)(6)
(6) ). The dry diameter can be measured and the interaction parameter is determined from .
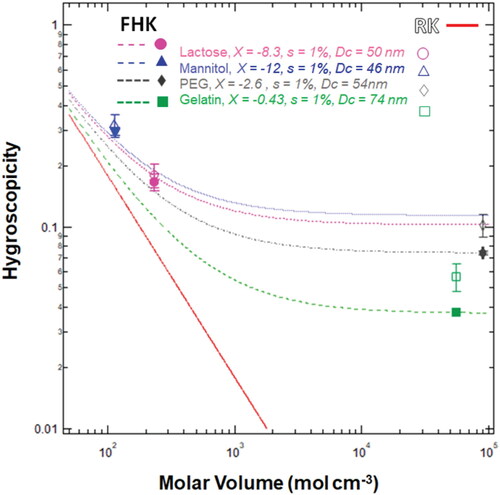
Previously measured polymer interaction parameters for PEG and gelatin range from 0.42 to 0.46 (Dormidontova Citation2002; Borchard, Bremer, and Keese Citation1980). These values are obtained from density differences of the melting temperature induced by changing the concentration of polymer in water. χ values less than 0.5 favor mixtures with water. Negative χ values suggest that interactions are highly favorable and water vapor readily adheres to the aerosol surface. In our study, we obtain the polymer water interaction parameters in a supersaturated environment. The values are negative (–2.6 for PEG and −0.42 for gelatin) and indicate that the propensity for water to adsorb on the nanoparticle surfaces in supersaturated conditions is more significant than previously considered. It should be noted that the derived interaction parameter does not include droplet curvature effects at the air-water interface; the surface tension of the droplet is assumed to be a dilute solution with the same as pure water. Additional surface tension measurements were conducted for PEG, mannitol, and lactose (see ). PEG is found to be weakly surface active, and gelatin was unable to be dissolved for surface tension droplet measurement. The negative χ for both PEG and gelatin confirms that the curvature effect for nano-scale droplet formation may be non-negligible. Thus the polymer water uptake behavior at the nano-scale may be different from the bulk-scale. Previous studies have observed these differences in scales for polymer surface property (Hahm Citation2014), mechanical property (Crosby and Lee Citation2007) and glass transition temperature studies (Napolitano and Wübbenhorst Citation2011). It is possible that χ, that accounts for surface tension affinity of water at the droplet air interface, would be smaller for nano particles than that of values derived from bulk phase study. Hence, the negative χ is likely derived from confounding effects of neglecting the surface tension contribution (EquationEquations (4)–(8)) or the nature of the nano sizes. More studies must be conducted to understand the polymer water interaction derived from CCN activation.
compares the calculated hygroscopicity (κRK or κFHK) as a function of the solute molar volume. Each solutes Dc is used to estimate the κRK and κFHK at supersaturation = 1%. The experimentally determined κRK and κFHK values for non-polymer compounds are similar (< 0.05 deviation) and within experimental uncertainty of mannitol and lactose data points. κRK and κFHK can be calculated from theoretical known values and compared to the calculated values from experimental data. κRK derived from experimental data do not fall on the theoretical κRK line (). RK theory assumes the ideal dilute droplet solution has a water activity equal to mole fraction of water and thus an increase in solute molar volume results in an exponential decrease in hygroscopicity. The dashed lines represent the Flory-Huggins Köhler theory under a constant dry diameter and best fit interaction parameter. For small molar volumes (< 100 mol cm−3), the FHK and RK converge with hygroscopicity in range of 0.3 to 0.4. However, as the molar volume increases, κFHK plateaus, and deviates from theoretical RK behavior. Hence, κFHK depends on both the dry diameter and the interaction parameter when the molar volume is large.
It is noted that the interaction parameter is an experimentally determined parameter and additional parameters are likely the reason why the predicted FHK hygroscopicity fits much better than the RK hygroscopicity. This is also why the Wolf model, with three additional fitting parameters for the interaction parameter is avoided. Instead, the interaction parameter is considered a constant in this work. To reduce the fitting parameters and make physical meaning between the parameters more explicit, we consider the interaction parameter as a constant independent to the volume fraction and compare the simplified model (κFHK-s) to κFHK.
For solutes such as lactose, gelatin and PEG, the FHK hygroscopicity decreases with the dry diameter (). The hygroscopicity for mannitol is almost a constant and does not decrease with the dry diameter and deviates from the prediction. Lactose, gelatin and PEG are larger compounds; the molecular weight and molar volume of mannitol is less than 200 g mol−1 and 200 × 10−6 mol m−3, respectively. Although the hygroscopicity is related to the dry diameter, for and non-polymer chemicals, the predicted hygroscopicity was underestimated, which means the entropy model for polymer is not applicable anymore. FHK hygroscopicity should not be used to calculate the dry diameter for non-polymer, especially by EquationEquation (8)(8)
(8) .
Figure 4. (a) Relationship between hygroscopicity and dry diameter. The hygroscopicity decreases with the increasing dry diameter in both theory and experiment except for mannitol. (b) Comparison of κ-hygroscopicity FHK to simplified FHK theory. The dashed lines are the simplified EquationEquation (7)(7)
(7) while the solid lines are the theoretical FHK prediction using EquationEquation (6)
(6)
(6) .
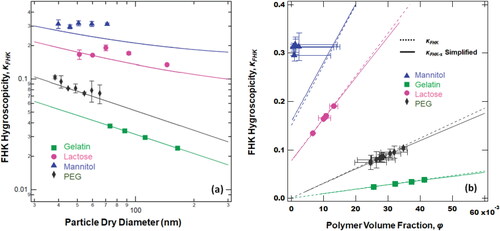
shows that the simplified FHK model (κFHK-s and EquationEquation (6)(6)
(6) ) works well for both experiment data and in theory. EquationEquation (6)
(6)
(6) predicts nanoparticle hygroscopicity if the volume fraction is known. For a small polymer (<0.1 and as is the case for nanoparticle water droplet formation), the simplified κFHK-s agrees well with FHK prediction. Furthermore, the simplified model works just as well as FHK for gelatin, lactose and PEG. Both models do not agree with mannitol experimental data. Mannitol has a constant hygroscopicity and constant volume fraction that is not related to the dry diameter.
5. Summary
Hygroscopicity is an important factor and estimates of particle hygroscopicity must also be determined at nano-scales. In this work, we showed the relationship between the hygroscopicity and the water-polymer interaction parameter for four common nano-particle compounds employed in aerosol therapeutics. The final wet particle size of inhaled and exhaled particles can be predicted by estimated and actual hygroscopicity values. Thus, it is critical to measure and also define accurate models to describe the water up-take of hydrophilic, biodegradable polymers frequently used as drug delivery materials to define aerosol therapy efficiency.
The supersaturated CCN measurement technique can measure they hygroscopicity of mannitol, lactose, gelatin and PEG particles. For all four compounds, traditional Raoult-Köhler theory poorly predicts the behavior of the organic compounds. Flory-Huggins Köhler theory with a constant interaction parameter can improve predictions of droplet formation. Indeed, the addition of another parameter is necessary, and the interaction parameter dominates the behavior of the hygroscopicity. The FHK model is less applicable for non-polymeric organics but can be applied to non-polymers with high molecular weights and molar volumes.
We studied the robustness of the FHK water uptake model for biodegradable nano particles and derived a simplified equation to be used if the RH and dry particle diameter is known. The application of the FHK model to hygroscopicity shows that for polymer material, entropy, the tendency of molecules mixing, the enthalpy and the interaction of the molecules must be considered. Under the good solvent assumption, the volume fraction is small and the hygroscopicity can be simply described as a function of dry diameter and interaction parameter. Our results show that for mannitol, a compound with molecular weight under 200 g mol−1, the hygroscopicity is constant and does not depend on the dry diameter. An independence of hygroscopicity with dry diameter suggest that entropic mixing is not important. Hence, the final size of the nano particles inhaled can be predicted through hygroscopicity if the dry diameter of the particles were known.
Author contributions
CM and AAA designed experiments and analytical methods. CM conducted the experiments, provided calculations and data analysis. Both authors contributed to the writing and preparation of the manuscript. KAM collected and analyzed surface tension data.
Supplemental Material
Download MS Word (69 KB)Disclosure statement
The authors declare that they have no conflict of interest.
Data availability statement
Data will be available from the authors upon request.
Additional information
Funding
References
- Agnihotri, S. A., N. N. Mallikarjuna, and T. M. Aminabhavi. 2004. Recent advances on chitosan-based micro- and nanoparticles in drug delivery. J. Control. Release. 100 :5–28. doi: 10.1016/j.jconrel.2004.08.010.
- Anbarasan, A., J. Nataraj, N. Shanmukhan, and A. Radhakrishnan. 2018. Effect of hygroscopicity on pharmaceutical ingredients, methods to determine and overcome: An overview. J. Chem. Pharm. Res 10 (3):61–7.
- Azarmi, S., W. H. Roa, and R. Löbenberg. 2008. Targeted delivery of nanoparticles for the treatment of lung diseases. Adv. Drug Deliv. Rev. 60 :863–75. doi: 10.1016/j.addr.2007.11.006.
- Borchard, W., W. Bremer, and A. Keese. 1980. The state diagram of the water-gelatin system. Colloid Polym. Sci. 258 (5):516–26. doi: 10.1007/BF01404159.
- Broday, D. M., and P. G. Georgopoulos. 2001. Growth and deposition of hygroscopic particulate matter in the human lungs. Aerosol Sci. Technol. 34 (1):144–59. doi: 10.1080/02786820118725.
- Crosby, A. J., and J. Lee. 2007. Polymer nanocomposites: The “nano” effect on mechanical properties. Polym. Rev. 47 (2):217–29. doi: 10.1080/15583720701271278.
- Dinar, E., T. Anttila, and Y. Rudich. 2008. CCN activity and hygroscopic growth of organic aerosols following reactive uptake of ammonia. Environ. Sci. Technol. 42 (3):793–9. doi: 10.1021/es071874p.
- Dormidontova, E. E. 2002. Role of competitive PEO-water and water-water hydrogen bonding in aqueous solution PEO behavior. Macromolecules 35 (3):987–1001. doi: 10.1021/ma010804e.
- Flory, P. J. 1942. The thermodynamics of high polymer solutions. V. Phase equilibria in the ternary system: Polymer 1-Polymer 2-solvent. Stat. Mech. Cross-Linked Polym. Networks II. Swelling J. Chem. Phys. 10 (279). doi: 10.1063/1.1723621.
- Fofie, E., V. Castelluccio, and A. Asa-Awuku. 2018. Exploring CCN droplet suppression with a higher sensitivity optical particle counter. Aerosol Sci. Technol. 52 (1):78–86. doi: 10.1080/02786826.2017.1379592.
- Gill, S., R. Löbenberg, T. Ku, S. Azarmi, W. Roa, and E. J. Prenner. 2007. Nanoparticles: Characteristics, mechanisms of action, and toxicity in pulmonary drug delivery - A review. J. Biomed. Nanotechnol. 3 :107–119. doi: 10.1166/jbn.2007.015.
- Hahm, J. I. 2014. Fundamentals of nanoscale polymer-protein interactions and potential contributions to solid-state nanobioarrays. Langmuir 30 (33):9891–904. doi: 10.1021/la404481t.
- Hindle, M., and P. W. Longest. 2010. Evaluation of Enhanced Condensational Growth (ECG) for controlled respiratory drug delivery in a mouth-throat and upper tracheobronchial model. Pharm. Res. 27 (9):1800–11. doi: 10.1007/s11095-010-0165-z.
- Karimi, M., W. Albrecht, M. Heuchel, M. H. Kish, J. Frahn, T. Weigel, D. Hofmann, H. Modarress, and A. Lendlein. 2005. Determination of water/polymer interaction parameter for membrane-forming systems by sorption measurement and a fitting technique. J. Memb. Sci. 265 (1–2):1–12. doi: 10.1016/j.memsci.2005.04.030.
- Kalberer, M., D. Paulsen, M. Sax, M. Steinbacher, J. Dommen, A. S. H. Prevot, R. Fisseha, E. Weingartner, V. Frankevich, R. Zenobi, et al. 2004. Identification of polymers as major components of atmospheric organic aerosols. Science 303 (5664):1659–62. doi: 10.1126/science.1092185.
- Kim, J. W., J. Xi, and X. A. Si. 2013. Dynamic growth and deposition of hygroscopic aerosols in the nasal airway of a 5-year-old child. Int. J. Numer. Method. Biomed. Eng. 29 (1):17–39. doi: 10.1002/cnm.2490.
- Köhler, H. 1936. The nucleus in and the growth of hygroscopic droplets. Trans. Faraday Soc. 32:1152–61. doi: 10.1039/TF9363201152.
- Kumar, P., A. Nenes, and I. N. Sokolik. 2009. Importance of adsorption for CCN activity and hygroscopic properties of mineral dust aerosol. Geophys. Res. Lett. 36 (24):L24804. doi: 10.1029/2009GL040827.
- Lathem, T. L., and A. Nenes. 2011. Water vapor depletion in the DMT continuous-flow CCN chamber: Effects on supersaturation and droplet growth. Aerosol Sci. Technol. 45 (5):604–15. doi: 10.1080/02786826.2010.551146.
- Longest, P. W., and M. Hindle. 2012. Condensational growth of combination drug-excipient submicrometer particles for targeted high efficiency pulmonary delivery: Comparison of CFD predictions with experimental results. Pharm. Res. 29 (3):707–21. doi: 10.1007/s11095-011-0596-1.
- Longest, P. W., G. Tian, and M. Hindle. 2011. Improving the lung delivery of nasally administered aerosols during noninvasive ventilation-An application of Enhanced Condensational Growth (ECG). J. Aerosol Med. Pulm. Drug Deliv. 24 (2):103–18. doi: 10.1089/jamp.2010.0849.
- Malcolmson, R. J., and J. K. Embleton. 1998. Dry powder formulations for pulmonary delivery. Pharm. Sci. Technol. Today. 1 :394–98. doi: 10.1016/S1461-5347(98)00099-6.
- Man, N. C., S. M. Kreidenweis, and C. K. Chan. 2008. Measurements of the hygroscopic and deliquescence properties of organic compounds of different solubilities in water and their relationship with cloud condensation nuclei activities. Environ. Sci. Technol. 42 (10):3602–8. doi: 10.1021/es7023252.
- Moore, R. H., A. Nenes, and J. Medina. 2010. Scanning mobility CCN analysis—A method for fast measurements of size-resolved CCN distributions and activation kinetics. Aerosol Sci. Technol. 44 (10):861–71. doi: 10.1080/02786826.2010.498715.
- Muralidharan, P., M. Malapit, E. Mallory, D. Hayes, and H. M. Mansour. 2015. Inhalable nanoparticulate powders for respiratory delivery. Nanomed. Nanotechnol. Biol. Med. 11 :1189–99. doi: 10.1016/j.nano.2015.01.007.
- Napolitano, S., and M. Wübbenhorst. 2011. The lifetime of the deviations from bulk behaviour in polymers confined at the nanoscale. Nat. Commun. 2 (1):1–7. doi: 10.1038/ncomms1259.
- Petters, M. D., and S. M. Kreidenweis. 2007. A single parameter representation of hygroscopic growth and cloud condensation nucleus activity. Atmos. Chem. Phys. 7 (8):1961–71. doi: 10.5194/acp-7-1961-2007.
- Petters, M. D., S. M. Kreidenweis, A. J. Prenni, R. C. Sullivan, C. M. Carrico, K. A. Koehler, and P. J. Ziemann. 2009. Role of molecular size in cloud droplet activation. Geophys. Res. Lett. 36 (22):L22801. doi: 10.1029/2009GL040131.
- Petters, M. D., S. M. Kreidenweis, J. R. Snider, K. A. Koehler, Q. Wang, A. J. Prenni, and P. J. Demott. 2006. Cloud droplet activation of polymerized organic aerosol. Tellus B Chem. Phys. Meteorol. 58 (3):196–205. doi: 10.1111/j.1600-0889.2006.00181.x.
- Roberts, G. C., and A. Nenes. 2005. A continuous-flow streamwise thermal-gradient CCN chamber for atmospheric measurements. Aerosol Sci. Technol. 39 (3):206–21. doi: 10.1080/027868290913988.
- Shelly, M. P., G. M. Lloyd, and G. R. Park. 1988. A review of the mechanisms and methods of humidification of inspired gases. Intensive Care Med. 14 :1–9. doi: 10.1007/BF00254114.
- Sullivan, R. C., M. K. J. Moore, M. D. Petters, S. M. Kreidenweis, G. C. Roberts, and K. A. Prather. 2009. Atmospheric chemistry and physics effect of chemical mixing state on the hygroscopicity and cloud nucleation properties of calcium mineral dust particles. Atmos. Chem. Phys. 9 (10):3303–16. doi: 10.5194/acp-9-3303-2009.
- Tang, M., C. K. Chan, Y. J. Li, H. Su, Q. Ma, Z. Wu, G. Zhang, Z. Wang, M. Ge, M. Hu, et al. 2019. A review of experimental techniques for aerosol hygroscopicity studies. Atmos. Chem. Phys. 19 (19):12631–86. doi: 10.5194/acp-19-12631-2019.
- Visalakshi, N. A., T. T. Mariappan, H. Bhutani, and S. Singh. 2005. Behavior of moisture gain and equilibrium moisture contents (EMC) of various drug substances and correlation with compendial information on hygroscopicity and loss on drying. Pharm. Dev. Technol. 10 (4):489–97. doi: 10.1080/10837450500299883.
- Vu, D., S. Gao, T. Berte, M. Kacarab, Q. Yao, K. Vafai, and A. Asa-Awuku. 2019. External and internal cloud condensation nuclei (CCN) mixtures: Controlled laboratory studies of varying mixing states. Atmos. Meas. Tech. 12 (8):4277–89. doi: 10.5194/amt-12-4277-2019.
- Vu, T. V., J. M. Delgado-Saborit, and R. M. Harrison. 2015. A review of hygroscopic growth factors of submicron aerosols from different sources and its implication for calculation of lung deposition efficiency of ambient aerosols. Air Qual. Atmos. Health 8 (5):429–40. doi: 10.1007/s11869-015-0365-0.
- Weinmüller, C., C. Langel, F. Fornasiero, C. J. Radke, and J. M. Prausnitz. 2006. Sorption kinetics and equilibrium uptake for water vapor in soft-contact-lens hydrogels. J. Biomed. Mater. Res. 77A (2):230–41. doi: 10.1002/jbm.a.30598.
- Wolf, B. A. 2003. Chain connectivity and conformational variability of polymers: Clues to an adequate thermodynamic description of their solutions, 2. Macromol. Chem. Phys. 204 (11):1381–90. doi: 10.1002/macp.200350002.
- Zhang, Q., J. L. Jimenez, M. R. Canagaratna, J. D. Allan, H. Coe, I. Ulbrich, M. R. Alfarra, A. Takami, A. M. Middlebrook, Y. L. Sun, et al. 2007. Ubiquity and dominance of oxygenated species in organic aerosols in anthropogenically‐influenced Northern Hemisphere midlatitudes. Geophys. Res. Lett. 34 (13):L13801. doi: 10.1029/[email protected]/(ISSN)1944-8007.GRL40.
Appendix
Köhler theory shows that
(A.1)
(A.1)
where is saturation,
is the water activity of the solution and D is the diameter of the droplet. A is a coefficient related to pure water droplet properties which is equal to
(A.2)
(A.2)
where is the molecular weight of water,
is the gas constant,
is the temperature and
is the density of water.
is the surface tension of the droplet which is assumed to be the same as pure water.
If the solute is a polymer, then the water activity can be described through Flory-Huggins Theory
(A.3)
(A.3)
where is the volume fraction of the polymer.
is the Flory-Huggins interaction parameter which is assume to be independent of volume fraction since that water is a good solvent for the polymer solute.
is the ratio of the molecular volume of water and the solute.
(A.4)
(A.4)
where is the molecular volume of water and
is the molecular volume of the solute. Notice that F is the reciprocal of f in Petters et al. (Citation2006) study. The volume fraction is
(A.5)
(A.5)
where is the dry particle size of the polymer.
Combine EquationEquations (A.1)(A.1)
(A.1) and (A.3) we have the Flory- Huggins-Köhler theory
(A.6)
(A.6)
(A.6)
(A.6)
The definition of the hygroscopicity from Petters et al. is
(A.7)
(A.7)
Combine with Flory-Huggins theory (EquationEquation (A.3)(A.3)
(A.3) ), we have
(A.8)
(A.8)
From EquationEquation (A.8)(A.8)
(A.8) ,
now is a parameter that depends on the volume fraction of the polymer. The maximum
happens when
and becomes
(A.9)
(A.9)
To calculate the critical diameter and the critical volume fraction
where
we have
(A.10)
(A.10)
(A.11)
(A.11)
For a soluble polymer with high molecular volume, since and
is approaches zero, EquationEquation (A.11)
(A.11)
(A.11) can be further simplified, and
become
(A.12)
(A.12)
By Taylors’ expansion to the second order of EquationEquation (A.8)(A.8)
(A.8) , the hygroscopicity as a function of volume fraction can be written
(A.13)
(A.13)
Substitute EquationEquation (A.12)(A.12)
(A.12) into EquationEquation (A.13)
(A.13)
(A.13)
(A.14)
(A.14)