Abstract
Copyright © 2021 American Association for Aerosol Research
EDITOR::
There is a need for robust methodology to simulate the aerosolisation, suspension and deposition of infectious viral particles in a controlled environment to assess the impact of airborne transport on viral infectivity. These data are essential to predict disease dynamics across a range of environments, and to better understand the factors influencing airborne transmission. Viral airborne stability remains a critical parameter for epidemiological models used in the identification of infection mitigation strategies. Quantifying stability is challenging and often associated with a large margin of error, particularly over short timescales.
Studying the airborne survival of viruses requires the generation and suspension of particles in a reproducible manner, avoiding impacts of aerosolisation on viral stability. Particle size distributions, number concentrations and water content can vary at source and these variations must be controlled or accounted for. The environmental conditions to which the airborne virus is exposed must be controlled and measured, particularly relative humidity (RH) and temperature. Finally, aerosol samples must be taken at appropriate times to determine the fraction of virus surviving, without degrading viral infectivity.
The CELEBS (controlled electrodynamic levitation and extraction of bioaerosols onto a substrate) technique has previously been used to study the airborne survival of bacteria (Fernandez et al. Citation2020; Fernandez et al. Citation2019) and has been designed to overcome the limitations (Haddrell and Thomas Citation2017) of preexisting techniques, such as the Goldberg drum (Goldberg et al. Citation1958). An adapted CELEBS for studying airborne viral pathogens within a high microbiological containment laboratory is presented and benchmarked with the mouse hepatitis virus (MHV), a surrogate coronavirus for more pathogenic viruses.
The instrument () uses piezoelectric dispensers to generate droplets of reproducible size and composition (Davies Citation2019; Li et al. Citation2015), charged by a charge induction electrode, and then trapped in an electrodynamic field formed by two ring electrodes. Air of controlled temperature and RH is passed over the trapped droplets. The airflow is laminar as it enters the levitation chamber (), ensuring that the droplets experience the conditions set within the gas flow. The temperature is controlled by conditioning the flowing air with a thermoelectric cooled/warmed heat sink; the RH is controlled by mixing humidified and dry airflows. The accessible temperature and RH ranges are shown in . The droplet size is controlled by changing the electrical pulse width sent to the dispenser (); using dispensers with different sized orifices further increases the accessible size range. Once droplets have been generated and suspended, they can be levitated for <5 s to hours or even days. The number of droplets can be quantified using a camera imaging from above the trap ().
Figure 1. Overview of CELEBS instrument and experimental procedure. (a) Top-down schematic of the CELEBS instrument. (b) Image from top-down camera within instrument showing 12 levitating droplets. (c) Schematic of the airflow as it enters the CELEBS chamber. (d) Maximum RH accessible as a function of the temperature between the rings. As the temperature deviates from room temperature (21 °C) the range of accessible RH’s decreases. (e) Contour graph of the radius of water droplets generated by a dispenser (30 µm orifice) as a function of the voltage and width of the electrical pulse sent to the dispenser. (f) Images from wells containing 17CL-1 cells, displaying CPE as a result of MHV infection (top) and an uninfected well (bottom), alongside an example schematic distribution of CPE positive wells in a plate inoculated with levitated virus. (g) Relationship between CPE positive wells and actual infectious units calculated using the Poisson distribution equation. (h) Infectious virus per droplet (bars show the mean of 3 levitations, error bars show the standard deviation) reported from 5 s levitations, with unprocessed virus culture, virus culture centrifuged at 6000 g for 2 min, virus culture passed through a 0.45 µm filter, and the expected virus per droplet value calculated from the concentration of the virus stock (measured using TCID50) and the expected droplet volume.
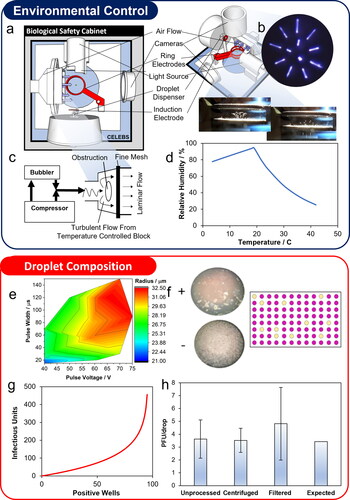
For all measurements reported here, the droplets have a higher water activity after generation than can be sustained by the sub-saturation RH (Walker et al. Citation2021). They rapidly lose moisture and size to form a composition that has an equal water activity to the gas phase RH. At RHs below 75%, the droplet composition is supersaturated with solutes concentrations above their bulk solubility limit, a key contrast between studies of survival in bulk solution and in the aerosol phase. DMEM is a commonly used solution to generate aerosol for airborne survival studies, with salt concentrations that are comparable to respiratory aerosol (Hildes and Ferguson Citation1955; Young and Schneyer Citation1981). The physical and compositional changes experienced by the droplets from generation to equilibration with the environmental conditions in the trap (mirroring the changes that occur when respiratory aerosols are exhaled) can be inferred from dynamics measurements with droplets of the same initial composition and size using the comparative kinetics electrodynamic balance (CK-EDB) (Marsh et al. Citation2017; Haddrell et al. Citation2019; Marsh et al. Citation2019; Marcolli and Krieger Citation2006; Steimer et al. Citation2015; Fernandez et al. Citation2020). CK-EDB data has not been included in this study, as the intent of this body of work is to demonstrate the improvement of methodology for the study of viral infectivity.
After the desired time airborne, the droplets are deposited into a cell culture medium, then used to infect a susceptible cell line (17CL-1 mouse fibroblasts for this study) seeded in a 96 well plate. This is similar to the standard 50% tissue culture infective dose (TCID50) endpoint assay used to measure infectious virus titer (Reed and Muench Citation1938). The key to selecting the cell line is a visible cytopathic effect (CPE) on the cells when infected by the virus (). The number of infectious viral particles deposited into the media is then calculated from the wells showing CPE using the Poisson distribution equation (). The large number of wells inoculated and the absence of sample dilution (typical of TCID50), makes this method suitable for quantifying virus concentrations in the range of 1–10 infectious units per ml. Thus, fewer droplets are used per measurement, resulting in lower variability, and generating higher resolution datasets. Quantifying the total virus extracted from the levitation, coupled with the number of droplets levitated, allows an estimate of the average virus per droplet for each levitation. When short levitations (<5 s) were carried out at high RH’s (>70%), the virus per droplet measured matched the value expected from the initial titer of the virus stock and the expected droplet volume, even when debris removal protocols were used (). Thus, measurements from short levitations at high RH can be used as a reference point to which subsequent measurements can be compared and normalized, a T0 (t = 0 s) point.
To demonstrate the capabilities of the instrument and method, a benchmark study was completed using MHV. Levitations for 30 s, 2 min, 5 min, and 10 min were carried out at 50% RH, 21 °C consistent with typical indoor conditions (). CELEBS measurements of airborne stability were more precise than other standard techniques such as the rotating drum (). The infectivity of MHV declined rapidly whilst airborne, with only 53% of virus remaining infectious after 2 min. However, the rate of decay decreased over time. To infer the impact of RH on this decay, the percentage infectivity remaining at 2 and 5 min was measured at 30%, 70%, and 90% RH at 21 °C (). At both timepoints an increase in infectivity with RH is observable. The relationship between temperature and remaining infectivity at 5 min is reported in . Decreasing the temperature while keeping the RH at 30% significantly reduced the loss of infectivity (p-value <0.015, 10 °C and 21 °C), with the virus showing no measurable drop in infectivity at 10 °C. However, increasing the temperature to 30 °C leads to a drop in infectivity comparable to 21 °C.
Figure 2. Demonstration of CELEBS study of viral airborne stability using MHV. Data are the mean of ≥3 measurements with error bars showing standard error. Percentage infectivity from individual measurements used to generate a–e are found in Table S1 (supplementary material). (a) 10-min decay curve of percentage infectivity with MHV levitated in DMEM droplets at 50% RH, 21 °C. (b) Investigation of the effect of RH on MHV airborne stability in DMEM droplets at 2 min and 5 min, 21 °C. (c) Investigation of the influence of temperature on MHV airborne stability in DMEM droplets at 5 min, 30% RH. (d) The relationship between absolute humidity and airborne stability of MHV at 5 min (data from (b) and (c)). (e) 1 h decay curve of percentage infectivity over time for MHV levitated in DMEM droplets at 30% RH, 21 °C. Inset into top right corner of figure is enlarged view of the first 5 min of the curve. (f) Investigation of the impact of airborne transport on the subsequent surface stability of MHV. Blue bars show the % infectivity of MHV in 50 µl DMEM droplets pipetted directly onto the surface and incubated at 21 °C, 30% RH for 1 h. Orange bars show the % infectivity of MHV in DMEM droplets deposited onto the surface and incubated for 1 h at 21 °C, 30% RH after being first levitated in CELEBS for 30 s at 21 °C, 30% RH.
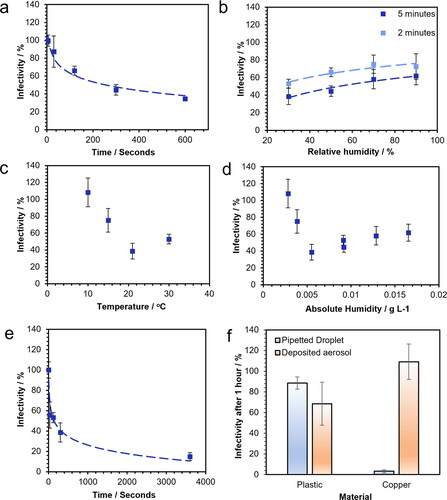
Previous studies have speculated that absolute humidity may be the best predictor of the loss of infectivity experienced by airborne viruses (Shaman and Kohn Citation2009; Marr et al. Citation2019). Although the absolute humidity describes the absolute mass concentration of water in air, it does not describe the availability of water to an airborne particle, which is instead impacted by RH and its equivalent, water activity, in the condensed phase. Furthermore, given that the saturation limit of water in air changes with temperature, it is possible to keep the absolute humidity the same whilst varying both temperature and RH; thus, a single absolute humidity value describes a wide range of conditions that can yield different desiccation kinetics at early time following droplet generation. The infectivity data collected in this study after 5 min is reported against absolute humidity in . Absolute humidity is clearly a poor variable for predicting loss of MHV infectivity in air; it important to be able to disentangle the effects of RH and temperature on airborne survival.
In addition to airborne stability, there is a need for approaches that robustly reproduce the stability of pathogens on surfaces under conditions typical of fomite formation from droplets of appropriate sizes (Goldman Citation2020). Typically, surface stability measurements are carried out by pipetting 10–100 µl of high titer viral suspension onto a surface (van Doremalen et al. Citation2020; Rabenau et al. Citation2005; Bean et al. Citation1982; Chin et al. Citation2020). Although accurately simulating contamination with large droplets, it does not recreate the deposition of smaller particles that have first undergone airborne processing typical of exhaled droplets. The physicochemical properties of smaller droplets are first influenced by the length of time they are airborne prior to deposition. For example, particles that have been airborne for longer will become dryer and more viscous, potentially resulting in less spreading and, therefore, less contact with the surface, reducing the impact of the surface on the stability of the virus. We compared the change in infectivity of MHV after 1 h on plastic and copper surfaces, both by pipetting directly a 50 µl droplet onto the surface (a volume used by van Doremalen et al. Citation2020) for SARS-CoV-2) and by first levitating droplets (initially 25 µm radius, <100 pL) for 30 s at 30% RH and then depositing onto the surface (). In the larger pipetted droplets, the average infectivity of the virus dropped to 89% after an hour on plastic and 3% on copper. However, whilst MHV was similarly stable in the smaller levitated droplets on plastic, it was found that the stability of the virus on copper was enhanced following aerosol processing (p < 0.005 when compared to the large droplet) with no measurable drop in infectivity detected within 1 hr. These surface measurements can be combined with airborne stability measurements, to provide an even clearer simulation of this potential transmission route.
Although MHV was relatively stable on the surface after an initial 30 s levitation (simulating airborne transport), the infectivity of the virus decreases to an average of 56% during levitation (), offsetting this apparent improvement in surface stability. The infectivity of MHV after 1 h in air at 30% RH was 14.8%, which whilst substantially lower than any measurement of infectivity of the deposited aerosol (even accounting for the drop in infectivity during the 30 s levitation), was higher than the infectivity on copper in the 50 µl pipetted droplet. These results demonstrate the limits to our understanding imposed by current research methods. CELEBS allows the more complete examination of conditions experienced by viruses during droplet transport and fomite formation, granting a more nuanced understanding of its role in the spread of disease.
The CELEBS technique represents a profound improvement in the ability to study the influence of environmental parameters on disease transmission. The technique allows the study of aerostability within monodisperse droplets of equilibrated sizes commensurate with droplet and airborne transmission, allowing the impact of droplet size on viral infectivity to be probed for the first time. The technique can be applied to very small amounts of virus, with most measurements in this study using less than 20 droplets each containing less than 5 individual infectious units. No additional concentration steps were needed for the virus culture to be useable in this instrument. The time-dependence of viral decay can be studied in far greater detail due to the ability to carry out levitations for times ranging from less than 5 s to hours. The high time resolution allows for the changes in infectivity that occur both during and after the rapid initial droplet evaporation to be measured and allow for measurements within timescales relevant to close contact droplet transmission as well as airborne transmission to be carried out. The instrument grants access to a broad range of environmental conditions and these conditions can be tightly controlled throughout the levitation, allowing for the independent assessment of the impact of temperature and RH on viral airborne infectivity. This has allowed for the clear disentanglement of the effects of relative and absolute humidity on viral airborne stability. The instrument allows for the direct comparison of surface stability in large, pipetted droplets to picolitre droplets that have undergone airborne equilibration, granting new insights into fomite transmission of viruses and demonstrating the complex interactions between the microenvironment within the droplet and the stability of the virus. In future studies, combining CELEBS measurements with CK-EDB measurements will offer unique insights into the interplay between aerosol microphysics and the survival of viruses in airborne droplets. Future application of this approach to the study of human pathogens, such as SARS-CoV-2, will provide the deeper level of understanding needed for the development of more accurate epidemiological models and the implementation of more effective infection control strategies.
Supplemental Material
Download PDF (639.4 KB)Additional information
Funding
References
- Bean, B.,. B. M. Moore, B. Sterner, L. R. Peterson, D. N. Gerding, and H. H. Balfour. 1982. Survival of influenza viruses on environmental surfaces. J. Infect. Dis. 146 (1):47–51. doi:10.1093/infdis/146.1.47.
- Chin, A. W. H., J. T. S. Chu, M. R. A. Perera, K. P. Y. Hui, H.-L. Yen, M. C. W. Chan, M. Peiris, and L. L. M. Poon. 2020. Stability of SARS-CoV-2 in different environmental conditions. Lancet. Microbe 1 (1):e10. doi:10.1016/S2666-5247(20)30003-3.
- Davies, J. F. 2019. Mass, charge, and radius of droplets in a linear quadrupole electrodynamic balance. Aerosol Sci. Technol. 53 (3):309–20. doi:10.1080/02786826.2018.1559921.
- Fernandez, M. O., R. J. Thomas, N. J. Garton, A. Hudson, A. Haddrell, and J. P. Reid. 2019. Assessing the airborne survival of bacteria in populations of aerosol droplets with a novel technology. J R Soc Interface . 16 (150):20180779 doi:10.1098/rsif.2018.0779.
- Fernandez, M. O., R. J. Thomas, H. Oswin, A. E. Haddrell, and J. P. Reid. 2020. Transformative approach to investigate the microphysical factors influencing airborne transmission of pathogens. Appl. Environ. Microbiol. 86 (23):e01543-20, 1–13. doi:10.1128/AEM.01543-20.
- Goldberg, L. J., H. M. S. Watkins, E. E. Boerke, and M. A. Chatigny. 1958. The use of a rotating drum for the study of aerosols over extended periods of time. Am. J. Hyg. 68 (1):85–93. doi:10.1093/oxfordjournals.aje.a119954.
- Goldman, E. 2020. Exaggerated risk of transmission of COVID-19 by fomites. Lancet. Infect. Dis. 20 (8):892–3. doi:10.1016/S1473-3099(20)30561-2.
- Haddrell, A., G. Rovelli, D. Lewis, T. Church, and J. Reid. 2019. Identifying time-dependent changes in the morphology of an individual aerosol particle from its light scattering pattern. Aerosol Sci. Technol. 53 (11):1334–51. doi:10.1080/02786826.2019.1661351.
- Haddrell, A. E., and R. J. Thomas. 2017. Aerobiology: Experimental considerations, observations, and future tools. Appl. Environ. Microbiol. 83 (17):1–15. doi:10.1128/AEM.00809-17.
- Hildes, J. A., and M. H. Ferguson. 1955. The concentration of electrolytes in normal human Saliva. Can. J. Biochem. Physiol. 33 (1):217–25. doi:10.1139/o55-031.
- Li, B., J. Fan, J. Li, J. Chu, and T. Pan. 2015. Piezoelectric-driven droplet impact printing with an interchangeable microfluidic cartridge. Biomicrofluidics 9 (5):054101 doi:10.1063/1.4928298.
- Marcolli, C., and U. K. Krieger. 2006. Phase changes during hygroscopic cycles of mixed organic/inorganic model systems of tropospheric aerosols. J. Phys. Chem. A. 110 (5):1881–93. doi:10.1021/jp0556759.
- Marr, L. C., J. W. Tang, J. Van Mullekom, and S. S. Lakdawala. 2019. Mechanistic insights into the effect of humidity on airborne influenza virus survival, transmission and incidence. J. R Soc. Interface 16 (150):20180298 doi:10.1098/rsif.2018.0298.
- Marsh, A., R. E. H. Miles, G. Rovelli, A. G. Cowling, L. Nandy, C. S. Dutcher, and J. P. Reid. 2017. Influence of organic compound functionality on aerosol hygroscopicity: dicarboxylic acids, alkyl-substituents, sugars and amino acids. Atmos. Chem. Phys. 17 (9):5583–99. doi:10.5194/acp-17-5583-2017.
- Marsh, A., G. Rovelli, R. E. H. Miles, and J. P. Reid. 2019. Complexity of measuring and representing the hygroscopicity of mixed component aerosol. J. Phys. Chem. A. 123 (8):1648–60. doi:10.1021/acs.jpca.8b11623.
- Rabenau, H. F., J. Cinatl, B. Morgenstern, G. Bauer, W. Preiser, and H. W. Doerr. 2005. Stability and inactivation of SARS coronavirus. Med. Microbiol. Immunol. 194 (1/2):1–6. doi:10.1007/s00430-004-0219-0.
- Reed, L. J., and H. Muench. 1938. A simple method of estimating 50 percent endpoints. American Journal of Epidemiology 27 (3):493–7. doi:10.1093/oxfordjournals.aje.a118408.
- Shaman, J., and M. Kohn. 2009. Absolute humidity modulates influenza survival, transmission, and seasonality. Proc. Natl. Acad. Sci. U S A. 106 (9):3243–8. doi:10.1073/pnas.0806852106.
- Steimer, S. S., U. K. Krieger, Y. F. Te, D. M. Lienhard, A. J. Huisman, B. P. Luo, M. Ammann, and T. Peter. 2015. Electrodynamic balance measurements of thermodynamic, kinetic, and optical aerosol properties inaccessible to bulk methods. Atmos. Meas. Tech. 8 (6):2397–408. doi:10.5194/amt-8-2397-2015.
- van Doremalen, N., T. Bushmaker, D. Morris, M. Holbrook, A. Gamble, B. Williamson, A. Tamin, et al. 2020. Aerosol and surface stability of HCoV-19 (SARS-CoV-2) compared to SARS-CoV-1. MedRxiv: The Preprint Server for Health Sciences. 382:1564–1567. doi:10.1056/NEJMc2004973.
- Walker, J. S., J. Archer, F. K. A. Gregson, S. E. S. Michel, B. R. Bzdek, and J. P. Reid. 2021. Accurate representations of the microphysical processes occurring during the transport of exhaled aerosols and droplets. ACS Cent. Sci. 7 (1):200–9. doi:10.1021/acscentsci.0c01522.
- Young, J. A., and C. A. Schneyer. 1981. Composition of Saliva in mammalia. Aust. J. Exp. Biol. Med. Sci. 59 (1):1–53. doi:10.1038/icb.1981.1.