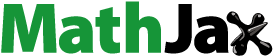
Abstract
We propose a simple method to control the size of nanoparticle generated by spark discharge. Nanoparticle characteristics are of importance for numerous applications. Property control can lead to desired applications. The spark discharge method can generate nanoparticles of high purity with ease. A pin-to-plate type spark discharge-nanoparticle generator (SDG) solely created approximately 1 metal particles/cm3 of geometric mean diameter (GMD) of 20.4 nm. Instead of pure gas, ethanol vapor and droplets from an atomizer were introduced into the SDG. The GMD was increased to 40.0 nm similar to the size of particles generated from the atomizer. The differences in discharge voltage and frequency were too small to change nanoparticle characteristics. To investigate this phenomenon, a bubbler replaced the atomizer and various solvents were used such as ethanol, methanol, and water. The GMD was reduced to 14.6 nm when the methanol bubbler was used. Organic solvent decreased GMD with decreased number concentration, while water did not contribute to the change in particle size. This suggests that vapor from the bubbler suppresses homogeneous nucleation while droplets from the atomizer induce heterogeneous nucleation. This research can be applied to nanoparticle control techniques for production processes.
Copyright © 2022 American Association for Aerosol Research
EDITOR:
1. Introduction
Nanoparticles can be applied to various fields. Due to their unique characteristics, they can be used for plasmonic phenomenon (Matricardi et al. Citation2018), therapeutic application (Wilhelm et al. Citation2016), and solar cells (Bai et al. Citation2012). Nanoparticles from the spark discharge method Citation(Tabrizi et al. 2009) can be prepared without suspension liquid or any impurities. Metal nanoparticles can be easily oxidized, however, they can be suppressed via several methods including generation in the reduction atmosphere (Hallberg et al. Citation2018). Research regarding the spark discharge includes the optimization of energy transfer to the interelectrode gaps during the synthesis of nanoparticles (Mylnikov, Efimov, and Ivanov Citation2019), the generation of mixed metallic nanoparticles (Tabrizi et al. Citation2010), the assembly of nanoparticles for gas sensing (Bae et al. Citation2017), and the generation of catalytic materials (Messing et al. Citation2010).
Nanoparticle size control is quite important for the various applications mentioned above. When the process leads in controlled way, nanoparticles are useful for desired applications such as mechanical (Ashraf et al. Citation2018; Oberdisse Citation2006), optical (Ma et al. Citation2016), and biological behaviors (Zook et al. Citation2011).
The combinations of liquid droplets and spark discharge have been investigated because a spark ignition system in internal combustion engines also involves instantaneous liquid fuel sprays through atomization and continuous sparks by electrical current supply in a confined space as a spark discharger (Danis, Namer, and Cernansky Citation1988). For example, silicone oil and ethanol droplets larger than 20 µm were used for the interaction between streamer-induced discharges and droplets (Tardiveau and Marode Citation2003). The influence of water-droplet sizes on the electrical discharge was investigated (Deng et al. Citation2010).
Here, we report the effect of ethanol atomization on the generation of nanoparticles such as silver made from spark discharge. Our spark discharge system could generate silver nanoparticles of geometric mean diameter (GMD) of 20.5 nm without an atomizer. By changing dilution flowrates and discharge parameters with ethanol atomizer, silver nanoparticles showed changes in the particle size and the number concentration. To understand this phenomenon, a bubbler was adopted to apply solvent vapor atmosphere to spark discharge chamber. Sampled particles were analyzed through a transmission electron microscopy (TEM) and the emission spectra were compared with each condition.
2. Materials and methods
An experimental setup to study the effect of droplet aerosol on characteristics of spark, discharge and nanoparticle generator (SDG) is shown in . This setup is composed of an atomizer, a custom-made SDG, a scanning mobility particle sizer (SMPS) for measuring nanoparticle size distributions and an oscilloscope for observing electrical spark discharge characteristics.
Figure 1. The experimental schematic (a) shows aerosol generation setup and (b) shows the electrical circuit around the SDG.
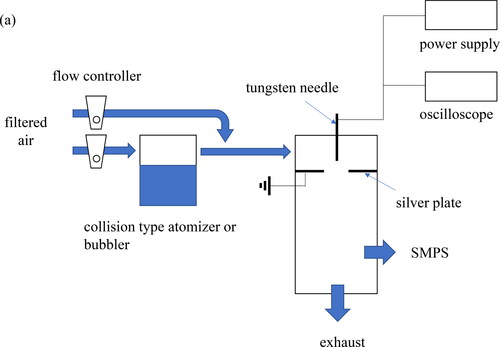
2.1. Aerosol generation
A single-jet atomizer (AG-01, HCTm Co., Ltd, Korea) and the SDG were connected in series. Compressed air through a diffusion dryer and a HEPA filter was used as the carrier gas and the dilution gas for aerosol generation. We used ethanol (99.5%, 459844, Sigma-Aldrich), methanol (99.8%, 179337, Sigma-Aldrich) and triple distilled water (HPLC grade, Duksan Chemical, Korea). Carrier gas entered the atomizer and ethanol aerosol was generated. Dilution gas was connected in parallel to the atomizer. The total flow was maintained as 5.0 L/min with atomizer flow and dilution flow. The size distribution of ethanol aerosol was controlled by changing atomizer gas flow rate and dilution flow rate through flow regulators connected on each flow paths. A bubbler (Chojalab, Korea) was installed to investigate the effect of vapor during atomization. The temperature of the bubbler was maintained between 20 ∼ 25 °C.
The SDG used in this experiment is similar to the device used in the previous experiment (Han et al. Citation2012). The 2 mm diameter electrode connected to the external circuit for the generation of spark discharge was pin-shaped and made of tungsten (tip angle 5.15°, 98% purity). The grounded plate was made of silver (1.0 mm thickness, 99.9% purity) with a 2 mm diameter hole. The hole center of the silver plate was aligned with the axis of the pin electrode. A positive high voltage power supply (Korea SMPS, C.160, Korea) provided 4.0 kV to the pin electrode of this SDG and the current was about 1.00 mA over the experimental cases. A fixed capacitance of 2 nF was connected to the power supply in parallel, and the resistance of 5 MΩ was set between the tungsten electrode and the power supply. For controlling inlet carrier gas condition, dilution air and the ethanol aerosol were mixed and entered the SDG.
2.2. Measurement
Electrical oscillation characteristics of spark discharge electrode were obtained using an oscilloscope (DSO-X 3014A, Agilent Technologies, USA) and a high voltage probe (P6015A, 100 MΩ, 3.0 pF, Tektronix) connected in parallel with the electrode. Oscilloscope signals were averaged over 10 measurements.
Size distributions of generated aerosol were measured at the inlet and outlet of SDG. An electrostatic classifier (DMA, TSI 3082) and a condensation particle counter (CPC, TSI 3775) were used to obtain the size distribution of aerosol at each sampling point. The size distributions were averaged over 3 measurements.
To confirm the effect of the ethanol atomizer on nanoparticles generated in SDG, we collected the nanoparticles on the TEM grid within the SDG chamber. To characterize particle generation, emission spectra were measured at the SDG using a spectrometer (FER-1024BRX, Princeton Instruments). The integration time was 3 s to obtain sufficiently averaged signal intensity over the spark discharge frequency which was approximately 260 Hz.
3. Results and discussion
To study the effect of ethanol atomizer inlet flow on nanoparticles generated in SDG, we measured the aerosol size distributions at the SDG inlet as changing the dilution ratios of the atomizer. The particle size distributions were obtained at the outlet of the SDG while the electrical potential of 4.0 kV was applied to the pin electrode of the SDG. As shown in , the number concentration of the particles measured at the outlet of the SDG changed as atomizer flow rate increased with maintaining the total inlet flow rate of 5.0 L/min at the SDG. Increasing the air flow rate at the atomizer, one mode started to appear around 40 nm while the other mode around 20 nm decreased.
Figure 2. Size distribution of (a) the particles from SDG followed by the ethanol atomizer with different flow rates and (b) particles measured without SDG.
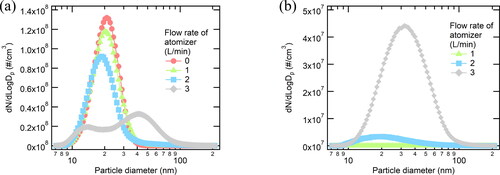
To understand the appearance of the larger particles and the disappearance of the smaller particles, we measured the size distribution of ethanol particles from the atomizer without spark discharge as shown in . It is probable that 30-nm particles induced only by the atomizer could reduce the number concentration of 20-nm particles during spark-discharge generation.
It is probable that impurities can remain after the ethanol atomization. According to Stabile et al. (Citation2013), the mode diameter is influenced by the volume fraction of solution impurities as follows:
(1)
(1)
where Dpp is the primary particle mode, Dd is the mode of droplets, and V is the volume fraction of impurities. We understand that the smaller particles around 30 nm in can be impurities generated from ethanol. The main component of the residue is thought to be ions in the solution as described in Park, McMurry, and Park (Citation2012), and Ichihara et al. (Citation2020).
We measured the size distribution of silver nanoparticle generated with the bubbler as shown in . As increasing the flow rate at the bubbler, GMDs and the number concentrations of SDG particles were decreased except for the case where water was used. The bubbling of ethanol and methanol definitely contributed to the generation of smaller particles while the use of water had nothing to do with the change in particle size.
Figure 3. Size distribution of nanoparticles generated by SDG with different flowrates at the liquid bubbler; (a) ethanol, (b) methanol, and (c) water.
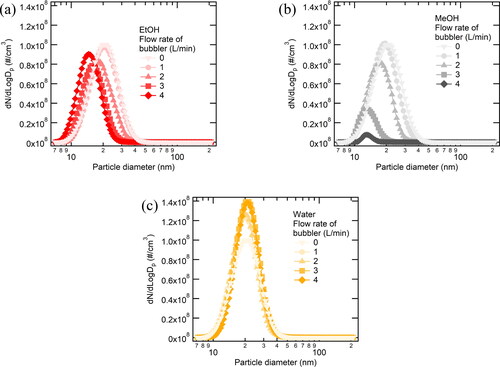
shows the changes in GMD depending on flow rates of the atomizer and the bubbler. As we introduced the ethanol particles with the atomizer, particle grew to 30.0 nm (47.3% of its original size). Meanwhile, supplying ethanol or methanol vapor to the SDG certainly decreased particle sizes down to 13.8 nm (31.9% of its original sizes).
Vapor pressures of methanol, ethanol and water at 25 °C are 0.167, 0.0790 and 0.0313 atm, respectively. These values show close relationship between SDG silver particles. During spark discharge process, spontaneous evaporation of metal leads to homogeneous nucleation. Vapor pressure during particle growth in each organic vapor can reduce particle growth as in the following EquationEquation (2)(2)
(2) (Wu and Biswas, Citation1998),
(2)
(2)
where dp is the particle diameter, D is the diffusion coefficient,
is the molecular volume, Nav is Avogadro’s number, C is the vapor concentration, and Cs is the vapor concentration at particle surface. The condensation rate is closely related to the particle size. Thus, it is valid that the reduced particle size is caused by the suppression of homogeneous nucleation through the introduction of organic vapors.
Supplying volatile liquid vapor into the spark discharge zone, the particle size would be reduced. The particle size generated by our SDG is inversely related to the vapor pressure of solvent. The particle size generated with the atomizer was nominally few tens of nanometers. The atomizer-generated particles which are larger than the SDG particles with air can contribute to heterogeneous nucleation. Therefore, the particle size can be increased with increasing flow rate of atomizer.
To observe the vapor or particle effect on the crystallinity or shape of the spark discharge-generated particles, TEM images of silver particles generated for various conditions were obtained as shown in . Fringe patterns were observed from each particle images. The most frequent d-spacings were approximately 0.21 nm which corresponded to (2 0 0) plane of silver (Agnihotri, Mukherji, and Mukherji Citation2014) over the experimental conditions. This means that the crystalline states of generated silver particles were definitely identical with each other.
Figure 5. TEM images of particles generated (a) without an atomizer or a bubbler, (b) at 3 L/min of ethanol atomizer, (c) at 3 L/min of ethanol bubbler, (d) at 3 L/min of methanol bubbler, (e) at 3 L/min of water bubbler.
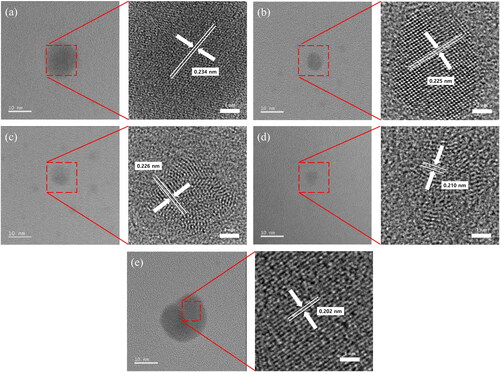
We performed SEM-EDS observations (see the online supplementary information) on generated particles to identify tungsten component. The atomic percentages of tungsten to silver were approximately 15%. This suggests that the tungsten anode was evaporated during spark discharge.
The electrical discharge characteristic is known as highly associated with the generation of nanoparticles by the electrode evaporation in SDG. The energy accumulated in the external capacitor becomes a current flowing between the electrodes during spark discharge and evaporates the electrode in SDG. Therefore, the amount of nanoparticles generated in SDG is related to the discharge voltage (Vd) and the frequency (f) (Janda et al. Citation2016; Tabrizi et al. Citation2009) as follows:
(3)
(3)
where C is the capacitance.
shows the electrical characteristics of spark discharge under each experimental condition. There was no distinctive difference in the morphology of particles between experimental conditions. This means that the energy used in SDG ought to be similar to each other.
Figure 6. Discharge voltage (Vd) and frequency measured at SDG electrode using different carrier gas flowrates. (a) at EtOH atomizer, (b) at EtOH bubbler, (c) at MeOH bubbler, (d) at water bubbler. Error bars indicate standard deviations over 10 repeated measurements.
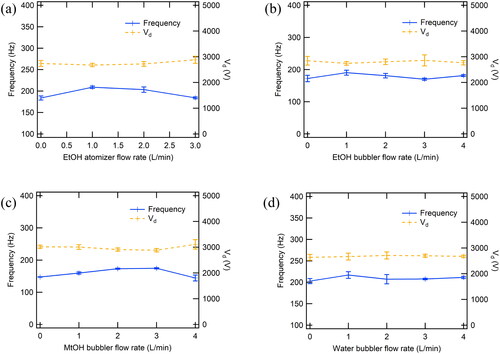
In order to examine the discharge characteristics in terms of spark electrical energy, emission intensities from SDG were measured using the spectrometer during spark discharge. The role of volatile liquid at the bubbler can be characterized by emission spectra. shows the emission spectra for each experimental condition where the dilution air flowrate was 2 L/min. The difference between peaks showed no tendency over experimental conditions. Instead, the peak wavelength was nearly identical with each other. The peaks at 337, 421, 478 and 523 nm can be identified as silver (Ahmat, Ahmed, and Nadeem Citation2014). Hydrogen and oxygen can be identified as the peaks at 656 and 777 nm, respectively (Tewari et al. Citation2014). Emission mechanism is closely related to the metal electrode evaporation. Evaporation occurs in the range of microseconds which is relatively shorter than particle growth time. Therefore, the emission spectrum shown in explains that the supply of volatile liquid vapor might not affect the plasma characteristics associated with the metal vapor generation.
4. Conclusions
We have investigated the effect of the flow of droplets generated in the atomizer into the SDG on the nanoparticle size. The addition of ethanol droplets increased particle size as well as number concentration. Ethanol droplets increased the size of silver nanoparticles generated at the SDG with heterogeneous nucleation. Ethanol vapor alone reduced silver particle concentration with reducing vapor pressure of silver. The change in nanoparticle size distribution proves that the atomizer or bubbler can distort particle nucleation process without changing electrical discharge characteristics. With this knowledge, we can obtain better controllability over nanoparticle formation process in the spark discharge by simply adding an atomizer or a bubbler for various nanoparticle applications.
Supplemental Material
Download MS Word (4.1 MB)Supplemental material
Supplementary material: analysis on the spark discharge electrode as well as generated particles is described.
Disclosure statement
The authors declare that they have no known competing financial interests or personal relationships that could have appeared to influence the work reported in this article.
Additional information
Funding
References
- Agnihotri, S., S. Mukherji, and S. Mukherji. 2014. Size-controlled silver nanoparticles synthesized over the range 5–100 nm using the same protocol and their antibacterial efficacy. RSC Adv. 4 (8):3974–83. doi:10.1039/C3RA44507K.
- Ahmat, L., I. Ahmed, and A. Nadeem. 2014. Infrared laser induced plasma diagnostics of silver target. Phys. Plasmas 21 (9):093501. doi:10.1063/1.4894221.
- Ashraf, M. A., W. Peng, Y. Zare, and K. Y. Rhee. 2018. Effects of size and aggregation/agglomeration of nanoparticles on the interfacial/interphase properties and tensile strength of polymer nanocomposites. Nanoscale Res. Lett. 13 (1):214.
- Bae, Y., P. V. Pikhitsa, H. Cho, and M. Choi. 2017. Multifurcation assembly of charged aerosols and its application to 3D structured gas sensors. Adv. Mater. 29 (2):1604159. doi:10.1002/adma.201604159.
- Bai, Y., H. Yu, Z. Li, R. Amal, G. Q. Lu, and L. Wang. 2012. In situ growth of a ZnO nanowire network within a TiO2 nanoparticle film for enhanced dye-sensitized solar cell performance. Adv. Mater. 24 (43):5850–6. doi:10.1002/adma.201201992.
- Danis, A. M., I. Namer, and N. P. Cernansky. 1988. Droplet size and equivalence ratio effects on spark ignition of monodisperse N-heptane and methanol sprays. Combust. Flame 74 (3):285–94. doi:10.1016/0010-2180(88)90074-0.
- Deng, H., Z. He, J. Ma, Y. Xu, J. Liu, and R. Guo. 2010. Initiation and propagation of discharge in liquid droplets: Effect of droplet sizes. IEEE Trans. Plasma Sci. 38 (12):3282–8. doi:10.1109/TPS.2010.2051687.
- Hallberg, R. T., L. Ludvigsson, C. Preger, B. O. Meuller, K. A. Dick, and M. E. Messing. 2018. Hydrogen-assisted spark discharge generated metal nanoparticles to prevent oxide formation. Aerosol Sci. Technol. 52 (3):347–58. doi:10.1080/02786826.2017.1411580.
- Han, K., W. Kim, J. Yu, J. Lee, H. Lee, C. Gyu Woo, and M. Choi. 2012. A study of pin-to-plate type spark discharge generator for producing unagglomerated nanoaerosols. J. Aerosol Sci. 52:80–8. doi:10.1016/j.jaerosci.2012.05.002.
- Ichihara, F., K. Lee, M. Sakamoto, H. Higashi, and T. Seto. 2020. Aerosolization of colloidal nanoparticles by a residual-free atomizer. Aerosol Sci. Technol. 54 (10):1223–30. doi:10.1080/02786826.2020.1770197.
- Janda, M., V. Martišovitš, K. Hensel, and Z. Machala. 2016. Generation of antimicrobial NOx by atmospheric air transient spark discharge. Plasma Chem. Plasma Process. 36 (3):767–81. doi:10.1007/s11090-016-9694-5.
- Ma, Q., H. Zhang, W. Liu, J. Ge, J. Wu, S. Wang, and P. Wang. 2016. Surface-enhanced Raman scattering substrate based on cysteamine-modified gold nanoparticle aggregation for highly sensitive pentachlorophenol detection. RSC Adv. 6 (88):85285–92. doi:10.1039/C6RA15774B.
- Matricardi, C., C. Hanske, J. L. Garcia-Pomar, J. Langer, A. Mihi, and L. M. Liz-Marzán. 2018. Gold nanoparticle plasmonic superlattices as surface-enhanced Raman spectroscopy substrates. ACS Nano 12 (8):8531–9.
- Messing, M. E., R. Westerström, B. O. Meuller, S. Blomberg, J. Gustafson, J. N. Andersen, E. Lundgren, R. van Rijn, O. Balmes, H. Bluhm, et al. 2010. Generation of Pd model catalyst nanoparticles by spark discharge. J. Phys. Chem. C 114 (20):9257–63. doi:10.1021/jp101390a.
- Mylnikov, D., A. Efimov, and V. Ivanov. 2019. Measuring and optimization of energy transfer to the interelectrode gaps during the synthesis of nanoparticles in a spark discharge. Aerosol Sci. Technol. 53 (12):1393–403. doi:10.1080/02786826.2019.1665165.
- Oberdisse, J. 2006. Aggregation of colloidal nanoparticles in polymer matrices. Soft Matter 2 (1):29–36. doi:10.1039/b511959f.
- Park, J. Y., P. H. McMurry, and K. Park. 2012. Production of residue-free nanoparticles by atomization of aqueous solutions. Aerosol Sci. Technol. 46 (3):354–60. doi:10.1080/02786826.2011.631614.
- Stabile, L., C. V. Trassierra, G. Dell’Agli, and G. Buonanno. 2013. Ultrafine particle generation through atomization technique: The influence of the solution. Aerosol Air Qual. Res. 13 (6):1667–77. doi:10.4209/aaqr.2013.03.0085.
- Tabrizi, N. S., M. Ullmann, V. A. Vons, U. Lafont, and A. Schmidt-Ott. 2009. Generation of nanoparticles by spark discharge. J. Nanopart. Res. 11 (2):315–32. doi:10.1007/s11051-008-9407-y.
- Tabrizi, N. S., Q. Xu, N. M. van der Pers, and A. Schmidt-Ott. 2010. Generation of mixed metallic nanoparticles from immiscible metals by spark discharge. J. Nanopart. Res. 12 (1):247–59. doi:10.1007/s11051-009-9603-4.
- Tardiveau, P., and E. Marode. 2003. Point-to-plane discharge dynamics in the presence of dielectric droplets. J. Phys. D: Appl. Phys. 36 (10):1204–11. doi:10.1088/0022-3727/36/10/309.
- Tewari, S. V., R. J. Kshirsagar, A. Roy, R. Sarathi, A. Sharma, and K. C. Mittal. 2014. Optical emission spectroscopy study on flashover along insulator surface due to particle contamination. Laser Part. Beams 32 (4):681–9. doi:10.1017/S0263034614000718.
- Wilhelm, S., A. J. Tavares, Q. Dai, S. Ohta, J. Audet, H. F. Dvorak, and W. C. W. Chan. 2016. Analysis of nanoparticle delivery to tumours. Nat. Rev. Mater. 1 (5):16014. doi:10.1038/natrevmats.2016.14.
- Wu, C.-Y., and P. Biswas. 1998. Particle growth by condensation in a system with limited vapor. Aerosol Sci. Technol. 28 (1):1–20. doi:10.1080/02786829808965508.
- Zook, J. M., R. I. MacCuspie, L. E. Locascio, M. D. Halter, and J. T. Elliott. 2011. Stable nanoparticle aggregates/agglomerates of different sizes and the effect of their size on hemolytic cytotoxicity. Nanotoxicology 5 (4):517–30.