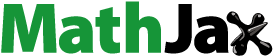
Abstract
For accurate laser-induced incandescence (LII) measurements of soot properties it is of great importance to understand the nature of the physical processes involved during rapid laser heating. In this work, we investigate how well-characterized differently matured fresh soot from a soot generator responds to rapid laser heating. For this purpose, a double-pulse LII setup is used with 10 μs time separation between the pulses using various combinations of two common LII wavelengths (532 and 1064 nm). Detection is performed at two wavelength bands for fluorescence analysis, and additionally elastic light scattering is used for mass loss analysis during heating. We investigate how the LII signal changes with pre-heating laser energy, specifically by fluence curve analysis to estimate the influence of thermal annealing, sublimation and laser-induced fluorescence interference. It is shown that extensive absorption enhancement occurs for all types of soot as the soot is thermally annealed, which is manifested through decreasing dispersion coefficient ξ and an increasing absorption coefficient E(m,λ). When comparing young and mature soot, a much larger impact of sublimation can be observed in the fluence curves of the mature soot. Also, we observe an enhanced contribution of laser-induced fluorescence for the young soot when performing LII measurements using 532 nm, which is suggested to originate from vaporized carbon fragments with an aromatic structure. This work further shows the potential of utilizing double-pulse arrangements for increasing the detectability of poorly absorbing soot, but also it highlights the impact of laser heating on soot, which may be important to avoid interferences when performing soot diagnostics.
EDITOR:
1. Introduction
Throughout the formation and maturation process of soot in a combustion environment, its optical and physicochemical properties change drastically (López-Yglesias, Schrader, and Michelsen Citation2014; Apicella et al. Citation2015; Johansson et al. Citation2017; Michelsen Citation2017). Hence, when soot is rapidly cooled and emitted from the combustion process, its properties will depend on residence time, temperature, and the chemical environment where it was formed. The optical and physicochemical properties of the fresh soot may range from small poorly absorbing soot particles with a high wavelength dependence (Simonsson et al. Citation2015; Török et al. Citation2018; Michelsen et al. Citation2020) and an disordered nanostructure with low C/H ratio of about 1.4–2.5 (Michelsen et al. Citation2020), to large dendritic shaped aggregates including highly ordered graphitic structures and a C/H ratio of 8–20 (see (Michelsen et al. Citation2020) and references therein) and of near black-body optical properties. The understanding of this wide spread of properties from different combustion processes and its relation to the maturity of soot is crucial, as various types of soot may impact climate (Bond et al. Citation2013, IPCC Citation2013) and human health (Janssen et al. Citation2012) differently. Also, difficulties may arise as diagnostic techniques for soot detection could respond differently to soot of different maturity.
Laser-induced incandescence (LII) is a common optical technique for soot diagnostics which can provide quantitative and/or qualitative measurements of different soot properties (Michelsen et al. Citation2015). The physical principle of the technique is based on heating of soot with high energy laser radiation until it reaches temperatures of 3000–4000 K, and the subsequent detection of the thermal radiation of the soot in the visible and near infrared spectrum. This enhanced thermal radiation is the LII signal. By investigating how the laser-soot interaction behaves as a function of laser energy, wavelength and time, one may obtain information about soot volume fractions, primary particle sizes, and absorption wavelength dependence. But as soot particles are heated to LII temperatures using a short laser pulse, the soot properties will to some extent be altered. The main processes which are related to the altering of soot and of the soot aerosol are (1) sublimation and vaporization, as carbon fragments and other species may be emitted from the soot surface (Olofsson et al. Citation2015), (2) thermal annealing, which may induce changes in the soot nano-structure and enhance the structural order with larger graphitic layers with less defects (Vander Wal, Ticich, and Stephens Citation1998; Vander Wal and Choi Citation1999; Apicella et al. Citation2019), and (3) new particle formation as the vaporized fragments can nucleate and form small particles with amorphous character (Michelsen et al. Citation2007, Migliorini et al. Citation2020). In order to perform optimized LII measurements on soot of different maturity, it is crucial to understand how these processes depend on the maturity of the soot.
Extensive work was done by Vander Wal and coworkers (Vander Wal, Ticich, and Stephens Citation1998; Vander Wal and Choi Citation1999) in the late 90´s to understand the influence of rapid laser heating on soot. By performing double-pulse LII experiments followed by transmission electron microscopy (TEM) on sampled soot, they observed the impact of rapid laser heating on soot as TEM micrographs revealed extensive graphitization of the soot as a result of thermal annealing. The enhanced graphitic properties of the soot also induced an enhancement of the LII signal detected after laser heating. The changes were observed at energies above 0.1 J/cm2 at 1064 nm, but it was also noted that significant mass loss occurred at energies above 0.45 J/cm2. In Cenker and Roberts (Citation2017) similar double-pulse experiments were performed on less mature soot in a Santoro burner flame and observations showed that pre-heating with higher fluences than 0.1 J/cm2 resulted in more rapid LII decay rates indicating significant sublimation, while fluences above 0.15 J/cm2 showed an enhanced LII signal due to the influence of thermal annealing. Possible differences in optical and physicochemical properties of the investigated soot may explain differences in sublimation and annealing thresholds. Recently Apicella et al. (Citation2019) investigated how rapid laser heating alters soot generated using different fuels (ethylene and methane) and specifically soot of different maturity. It was shown that the nanostructure of the soot was extensively altered, but differently for young and mature soot, as the young soot showed an onion-like structure with voids, while the mature soot showed a collapsed multifaceted rosette structure. Further investigations were performed by Migliorini et al. (Citation2020), who used broad-band extinction measurements of laser-heated soot in combination with Raman spectroscopy and scanning mobility particle sizing (SMPS). Soot from either ethylene or methane combustion were investigated and dispersion coefficients of ξ = 1.02 and 2.22 were estimated, respectively. The structural changes due to laser heating of the two types of soot showed differences, of which the ethylene soot specifically showed indications of decreasing maturity from extinction measurements (increasing dispersion coefficient) and from Raman spectroscopy (decreasing I(D)/I(G)). The size distribution measurements showed a smaller size mode appearing with increasing laser heating. Similar work was done by Michelsen et al. (Citation2007), who observed newly formed particles which appeared to have less structural order. Hence, it might be suggested that a possible explanation for the increasing dispersion coefficient in Migliorini et al. (Citation2020) is due to newly formed particles which may have optical properties similar to young soot both due to its bulk properties (Michelsen et al. Citation2007) and small size (Wan, Shi, and Wang Citation2020).
The objective of this work is to further explore how rapid laser heating influences characteristic properties of well characterized soot particles of different maturity by investigating the changes in laser-soot interaction after laser pre-heating of the soot. We specifically employ a 2-pulse (2P) LII setup as done in Cenker and Roberts (Citation2017) and Vander Wal, Ticich, and Stephens (Citation1998) to obtain information about the pre-heating influence on the soot, by comparing the LII signal from the soot with (2P) and without (1P) pre-heating. The 3 types of soot in this work, denoted OP1, OP6 and OP7, have previously been extensively investigated (Török et al. Citation2018; Le et al. Citation2019; Malmborg et al. Citation2019; Török, Mannazhi, and Bengtsson Citation2021) using optical as well as other aerosol measurement techniques to investigate the optical and physicochemical properties. Previous work has shown that OP1 soot is of mature black carbon (BC) character, with a dispersion coefficient of ∼1.2 (λ = 405 − 1064 nm (Török et al. Citation2018) and λ = 532 & 1064 (Török, Mannazhi, and Bengtsson Citation2021)), with E(m,1064 nm) = 0.33 (Török, Mannazhi, and Bengtsson Citation2021), and with an organic- to total carbon ratio (OC/TC) of 9% (Török et al. Citation2018). Both OP6 and OP7 soot is of young, partly matured BrC-like character as they absorb less efficiently and have been estimated to have a dispersion coefficient of 2.5 and 3.5 (λ = 405 − 1064 nm (Török et al. Citation2018)), and an OC/TC of 59% and 87%, respectively (Török et al. Citation2018). Further it was shown that heating the soot in a thermodenuder and an oven prior to diagnostics resulted in a lower dispersion coefficient for both OP6 and OP7 soot (2.3 and 2.5), however still significantly higher than that of OP1, suggesting that the refractory soot itself is of less mature character (Török et al. Citation2018).
An important part of this work is the use of LII fluence curve analysis, which was performed for the 3 types of mini-CAST soot of different maturity as the soot was pre-heated with a laser pulse of various amount of energy prior to the LII measurement. Special focus was directed on the OP1 and OP6 soot where the temporal LII signal is long enough to perform full analysis. Observations showed that the pre-heating influences the absorption properties of the soot as both less energy is needed to reach a certain LII signal, and as higher peak LII signals are reached for a certain pulse energy. Our results are in good agreement with previous work, suggesting that rapid laser heating will alter the soot by thermal annealing and mass loss by vaporization/sublimation. The effects of these processes was found to vary largely with the soot maturity. Additionally, for the less mature soot, an enhanced laser-induced fluorescence (LIF) signal contribution was observed during LII measurements using excitation at 532 nm and we hypothesize that this is related to the vaporized species, which may fluoresce when excited with 532 nm radiation.
2. Experimental setup
An overview of the LII-setup is shown in . The soot source for producing the soot aerosol is a mini-CAST soot generator model 5201 C (Jing Citation2009). The mini-CAST operates on a propane-air co-flow diffusion flame. By adjusting the fuel/air ratio and by mixing nitrogen into the fuel flow, the conditions of soot formation in the flame changes and different types of soot can be emitted when quenched by a steady nitrogen flow at a fixed height. The three types of polydisperse soot examined in this work have previously been investigated, showing large differences in maturity (Török et al. Citation2018; Le et al. Citation2019; Malmborg et al. Citation2019). The OP1 soot is mature, while OP6 soot and OP7 soot is of decreasing maturity and will be referred to as young soot. The soot concentration in the probe volume spanned about 2 orders of magnitude between the mature and young soot (∼200 ppb and a few ppb, respectively).
Figure 1. An overview of the double pulse (2P) LII setup. The angle between the two Nd:YAG laser beams is exaggerated for clarity. The laser beams cross in the probe volume just at the outlet of the mini-CAST soot generator. The detection setup allows for detection of the LII signal at three wavelength bands with center wavelengths of 684 nm, 575 nm, and 532 nm. The optics are denoted A – aperture, L – lens, DM – dichroic mirror, M – mirror and F – optical filter.
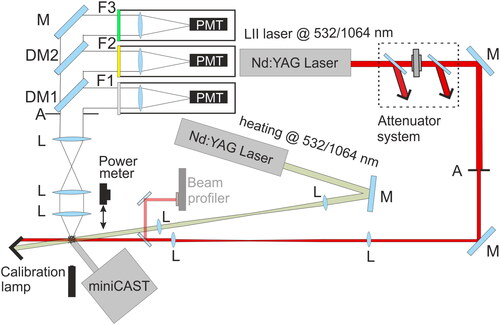
The pulsed laser system consisted of two Nd:YAG lasers (Quantel Brilliant B) operated at 1064 and 532 nm, respectively, which are common wavelengths used for LII measurements. A digital pulse generator was used to organize the timing of the two pulses, of which the first one performs the pre-heating and the second one is used for the LII measurement. The delay between the pulses was set to 10 μs to enable the pre-heated soot to reach ambient temperature before LII measurements using the second pulse. It should be noted that similar delays were used by both Vander Wal and Choi (Citation1999) and Cenker and Roberts (Citation2017), nevertheless, this choice of delay time was validated experimentally. For the pre-heating laser, the 4 mm center of the beam was cut out, reshaped and relay imaged in the probe volume at a size of 5 mm in diameter. The measurement laser beam was passed through an attenuation system of two thin film polarizers at the Brewster angle with a half-wave plate in-between. Similar to the pre-heating beam, the center 4 mm was cut out, reshaped and relay imaged into the probe volume, at a size of approximately 2 mm in diameter, resulting in a probe volume of ∼70 mm2. The laser beams which both had a top-hat spatial profile were overlapped using a small angle in between, and as the LII laser beam was positioned clearly inside the pre-heating beam, a homogeneous heating of the soot in the probe volume was assured.
For signal detection, three photomultiplier tubes (PMTs) were used within their range for linear response (Mansmann, Dreier, and Schulz Citation2017). Two were used for LII detection at 575 nm and 684 nm, both of type Hamamatsu H10721-20. The third PMT measured the elastic scattering from the soot particles during LII detection when the laser wavelength of 532 nm was used. This PMT was also from Hamamatsu but of type H6780-04. A 4-channel digital oscilloscope (LeCroy Waverunner 104MXi) with a sampling-rate of 1 GHz was used for signal collection. A beam profiler camera (Wincam D beam profiler) was used for beam profile monitoring during alignment and a Labsphere IES 1000 was positioned in front of the detection system for pyrometry calibration.
3. Methodology
The energy balance of a soot particle during heating by a laser pulse can be described by EquationEquation (1)(1)
(1) . The temperature evolution of the soot is coupled to the change of the internal energy
which depends on the heat exchange rates (denoted as
) of the different physical processes involved during and after the laser pulse.
(1)
(1)
The absorption rate ( is dictated by the input energy and the complex refractive index m of the soot material. The sublimation expressed through the rate
does not come into play until temperatures of around 3400 K, above which it becomes increasingly important with increase in soot temperature (Liu et al. Citation2006; Olofsson et al. Citation2015). Conduction (expressed through
)
is the dominating heat loss mechanism in most situations and is governed by the temperature difference between the soot and the surroundings. The radiative emission rate (
) depends on the complex refractive index of the soot but contributes much less to the overall energy balance in ambient condition (Michelsen et al. Citation2015). Finally, the rate
deals with other processes such as thermionic emission and annealing, which are often considered to influence the change in internal energy marginally. Annealing is, however, known to induce changes in the soot material and may influence its optical and physicochemical properties (Vander Wal, Ticich, and Stephens Citation1998; Vander Wal and Choi Citation1999; Michelsen et al. Citation2015; Apicella et al. Citation2019; Migliorini et al. Citation2020).
As described in (Sipkens and Daun Citation2017), the peak LII signal as a function of input energy, can be an effective tool for investigating the laser-soot interaction when performing LII measurements. In , an example of time-resolved experimental LII signals from laser heated soot is shown as the black lines. The peak LII intensity can be observed as a function of the input energy, namely the fluence curve which is shown as the red curve connecting the peaks. In the low fluence regime where is the dominant process, the temperature increase approximately follows a linear trend (De Iuliis et al. Citation2006; Sipkens and Daun Citation2017), which will result in a rapid LII signal increase due to its strong temperature dependence LII ∝ T5. As soot reaches temperatures above the sublimation threshold, which approximately coincides with the inflexion point of the fluence curve, sublimation will successively be the dominating loss mechanism and hinder the linear temperature increase. As can be seen in , the curve will level off as the increase in absorbed energy primarily goes into the sublimation process.
Figure 2. In (a) an example of experimental time-resolved LII signals as a function of laser fluence is shown. The red curve which connects the peak LII signal as a function of laser fluence, is termed fluence curve. In (b) a simulation of the influence of primary particle size and absorption properties on the fluence curve is illustrated. A top-hat laser profile, with an LII laser wavelength of 1064 nm and detection at 575 nm was used for the modeling case. Values of E(m) and dpp is specified in the legend.
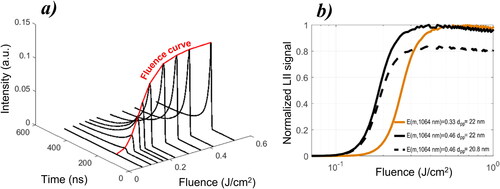
As soot is heated to high temperatures with a short laser pulse, the properties of the soot particles may, as previously discussed, change. As shown in Apicella et al. (Citation2019), Cenker and Roberts (Citation2017), Migliorini et al. (Citation2020), Vander Wal and Choi (Citation1999), Vander Wal, Ticich, and Stephens (Citation1998), restructuring of the soot material due to thermal annealing will influence the optical properties of the material, along with mass loss by sublimation. In , numerical simulations using the LII model is used to illustrate differences in fluence curve shape that may occur as a result of rapid laser heating. The increase in absorption efficiency is described by a change of the absorption function E(m) from 0.33 to 0.46, while the mass loss is described by a decrease of the primary particle size dpp from 22 nm to 20.8 nm. As can be seen, a change of E(m) results in a shift of the fluence curve toward lower fluences, while the decrease in dpp will result in a lower LII signal.
4. Results and discussion
The double-pulse LII measurements were done in three sets with the wavelength combinations of λpre-heat − λLII = 532–532, 1064–532, and 532–1064 nm, where λpre-heat is the wavelength of the first laser pulse responsible for the pre-heating and λLII is the wavelength of the second laser pulse, inducing the incandescence. The measurement cases with pre-heating will be denoted 2P (2-pulse) and the cases without pre-heating will be denoted 1P (1-pulse). Throughout the results and discussion, focus will be directed toward the comparison of mature OP1 soot and young OP6 soot, as OP7 soot gave signals with rather low signal-to-noise ratios in some measurement series.
4.1. Influence of pre-heating on fluence curves
All the fluence curves for the three wavelength combinations as well as for the OP1, OP6, and OP7 soot, with and without pre-heating are presented in , where the curves are normalized to the signal from non-heated soot with the detection wavelength of 575 nm. As indicated in the figure, the pre-heating appears to influence the fluence curves overall by I) inducing a change in the peak LII intensity, II) changing the amount of energy needed to heat the soot to peak LII, and III) for some pre-heated cases with LII using 532 nm excitation, extensive signal enhancement can be observed at low fluences. This signal enhancement is a laser-induced fluorescence interference, which will be further discussed in Section 3.5.
Figure 3. The fluence curves are shown for all double pulse (2P) measurements of OP1, OP6, and OP7 soot using detection at 575 nm. In (a)–(c), the pre-heating is done using 532 nm laser radiation and LII measurements are done using 1064 nm laser radiation (532–1064), ensuring interference-free LII. For (d)–(f) the pre-heat and LII combination is 532–532, and for (g)–(i) it is 1064–532. For these measurements LIF interference is expected for OP6 and OP7 soot, which consists of a significant amount of organic carbon. Some fluence curve changes induced by the pre-heating are highlighted with arrows, and are further discussed in the text.
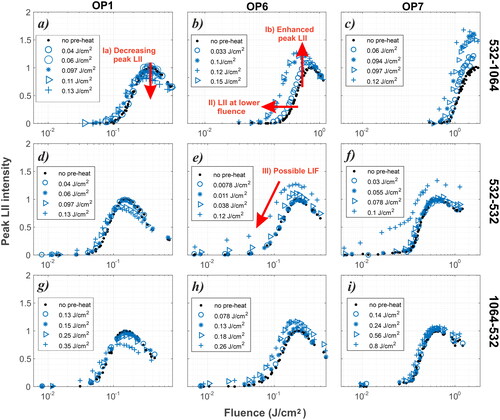
show the influence of a λpre-heat = 532 nm on the LII signal induced using λLII = 1064 nm. By increasing the pre-heat pulse fluence, it can be seen that at ∼0.06 J/cm2, the first shift of the fluence curve toward lower fluences can be observed for OP1 soot, still with the same LII peak intensity. This shift indicates increased absorption efficiency (as discussed in Section 3 in relation to ) and is assumed to be related to thermal annealing of the soot. This annealing becomes more efficient with increased pre-heat pulse energy, thereby shifting the fluence curve more with increasing pre-heat laser fluence.
Another effect which can be observed in for a pre-heat laser fluence of ∼0.097 J/cm2 and above, is a decrease of the peak LII signal. The lower peak LII signal can be related to mass loss of the particle through sublimation/vaporization as a result of the pre-heating. Hence, for this mature OP1 soot, the absorption efficiency due to annealing increases at much lower temperatures than the sublimation threshold. These observations of a decreasing LII peak with increasing pre-heating has also been observed by Cenker and Roberts (Citation2017) and Vander Wal, Ticich, and Stephens (Citation1998).
The analysis becomes less straightforward for OP6 and OP7 soot, which consist of both less mature soot and higher fraction of organic carbon which may partly evaporate at elevated temperatures (Török et al. Citation2018). For both OP6 and OP7 soot, the shift of the fluence curve becomes even more evident as shown in . Contrary to the OP1 case, the OP6 and OP7 soot show a strong peak signal enhancement for increasing pre-heat laser fluences which shows that the enhancement of LII signal due to annealing is the dominant process in comparison with the influence of sublimation. These observations will be further discussed in relation to , where extended analysis of the fluence curves in is presented.
Figure 4. The influence of pre-heating is shown on the peak LII signal and on the relative absorption efficiency in relation to the pre-heating energy. In (a) the relative peak intensity is shown for all studied soot when performing LII measurements using 1064 nm. The trend observed at the delayed LII signal (at 50 ns after peak LII) is also shown as gray lines for comparison. In (b) the relative curve position is shown for the same cases as in (a). In (c) and (d) the relative curve position for OP1 and OP6, respectively, can be observed for all experimental cases. Linear fitting is done for the pre-heated soot using 532 nm, to investigate the pre-heating influence on the dispersion coefficient. The dispersion coefficient as a function of the pre-heating energy is shown as the blue line, following the right blue y-axis. All pre-heating energies using 1064 nm are multiplied by the factor (1/2)ξ for the unheated mini-CAST soot.
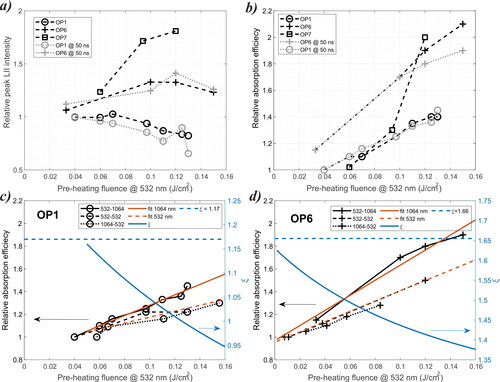
When comparing all of the fluence curves of OP1 soot, in , where the heating and LII wavelengths are different combinations of 532 and 1064 nm, it can be seen that the changes induced by the pre-heating will give roughly the same resulting fluence curve trends. This wavelength independent behavior can be related to its mature character, exposing no significant laser-induced fluorescence (LIF) contribution when performing LII with λLII = 532 nm ().
In contrast to the mature OP1 type of soot, the OP6 and OP7 soot is as discussed previously, less mature and consists of a relatively larger mass fraction of organic carbon which is partly refractory (Török et al. Citation2018). As can be observed in where the prompt LII fluence curves are shown for OP6 and OP7 soot, respectively when performing LII with λLII = 532 nm, some differences can be observed in relation to . The appearance of the curves looks similar but it can be observed that for OP6 soot, a distinct increase in LII signal can be seen at low fluences (as pointed out by the red arrow in ). Fluorescence is most likely a substantial part of the detected signal, and deeper analysis of this LIF contribution will be performed in Section 4.4.
In the change in relative peak intensity and the change in absorption efficiency are shown for various preheating energies using λLII = 1064 nm, based on the data presented in . The change in relative peak intensity is the ratio between the LII peak intensities, and the change in absorption efficiency is given from the relative shift of the fluence curve, specifically 1/shift as a shift to lower fluences can be converted to a higher absorption efficiency. The black lines represent prompt LII detection, while gray lines display the delayed LII detection at 50 ns after peak LII to assure detection of pure LII and avoidance of potential fluorescence interference. In , the change in peak LII signal intensity is shown. For OP1 soot the peak LII signal is rather constant until a pre-heat fluence of ∼0.07 J/cm2. For increasing fluences above 0.07 J/cm2, the peak LII signal decreases to peak LII signals below the original level of the unheated soot, suggesting extensive mass loss by sublimation, as discussed in relation to . For the OP6 soot, the peak LII intensity increases extensively by up to almost 40% above the unheated signal at 0.12 J/cm2 pre-heating, suggesting that annealing and potentially a higher peak LII temperature are the dominant mechanisms. The same applies for OP7 soot, however to a much larger extent as the peak LII is 80% higher than the non-pre-heated soot at 0.12 J/cm2 pre-heating. Hence, it appears that the thermal annealing process induces larger changes in the peak LII signal for the younger soot, prior to sublimation.
In , the influence of pre-heating on the fluence curve position as a result of changed absorption properties is shown, observed as the shift to lower fluences (as indicated by the horizontal arrow in ). The lower energy needed to reach a certain LII signal when the soot is pre-heated is related to the enhanced absorption efficiency as discussed earlier in relation to , hence shows the change in absorption efficiency. It can be seen that for the mature OP1 soot, the absorption efficiency increases for fluences higher than ∼0.05 J/cm2. For OP6 soot the fluence curve is shifted at lower fluences, while OP7 soot needs approximately the same amount of energy to induce any fluence curve shift as for OP1 soot. The reason for the non-consistent trend between the energy needed to induce a fluence curve shift in relation to the soot maturity may be due to a combination of 1) the absorption efficiency related to the soot maturity, 2) the extent of thermal annealing and its influence on the absorption efficiency and, 3) the potential influence of evaporated organic carbon and other volatile species of which an extensive amount is evaporated at relatively low temperatures (800 K (Török et al. Citation2018)).
The mature and the young soot show quite different behaviors from the results in . Soot absorption properties of OP1 soot starts to change at ∼0.05 J/cm2 (at 532 nm), while sublimation becomes significant at about ∼0.07 J/cm2. For OP6 soot, the soot absorption properties change already at 0.04 J/cm2, and there are indications of sublimation at around 0.1 J/cm2. For OP7 soot, the absorption property changes at ∼0.06 J/cm2, and for this case potential sublimation is masked by the increased annealing.
4.2. Influence on soot maturity
The dispersion coefficient ξ describes the wavelength dependence of the absorption cross section (and emissivity) of soot, see EquationEquation (2)(2)
(2) ,
(2)
(2)
where E(m,λ) is the absorption function which describes how efficiently soot absorbs electromagnetic radiation of a certain wavelength λ. Often the dispersion coefficient is determined in the visible and near infrared region using two or multiple wavelengths. In Török, Mannazhi and Bengtsson (Citation2021), two-wavelength LII was used to measure ξ, which was estimated to be 1.17, 1.7 and 2.3 for OP1, OP6, and OP7 soot, respectively, showing a strong relationship between dispersion coefficient and maturity. In , the change of the absorption efficiency for OP1 soot is shown as a function of pre-heating fluence for all experimental conditions, with focus on the cases λpre-heat-λLII = 532–532 and 532–1064 nm, where specifically the pre-heating conditions are the same. The interesting observation is that the slopes of the curves are different when comparing LII using 532 and 1064 nm to probe the preheated soot with varying fluence. By fitting a linear function to the experimental trends, shown as orange curves, the influence on the dispersion coefficient could be obtained from the ratio of the curves. The resulting trend for the dispersion coefficient ξ as a function of pre-heating is shown as the blue curve, following the right y-axis. The same procedure was done for OP6 soot, as can be seen in . According to the change of ξ with pre-heating, a decrease from 1.17 to just about 1 was observed for OP1 soot and a decrease from 1.66 to just about 1.4 was observed for OP6 soot, when pre-heating with 0.13 and 0.12 J/cm2, respectively. This shows how soot heating using a short laser pulse can change the optical properties of soot irreversibly, which has also been observed in Cenker and Roberts (Citation2017) and Vander Wal, Ticich and Stephens (1998).
The influence of thermal annealing by pre-heating the soot may also be quantified by observing how the fluence curve position changes with pre-heating fluence. As a LII signal is observed at successively lower fluences the higher pre-heating laser fluence is used, it implies an increase in the estimated E(m,λ). For OP1 and OP6 soot, the E(m,1064 nm) would change from 0.33 and 0.16 (Török, Mannazhi, and Bengtsson Citation2021) to approximately (not taking sublimation and change of thermal properties into account) 0.46 and 0.23, respectively when pre-heated using 532 nm radiation of 0.13 and 0.12 J/cm2.
4.3. Laser elastic scattering for mass loss estimation
The elastic light scattering (ELS) during laser-soot interaction was recorded for all LII measurements done using 532 nm as done in Witze et al. (Citation2001). As soot is heated to high temperatures, sublimation will occur and mass loss will influence the appearance of the scattering signal. At higher fluences, soot may reach sublimation temperatures before peak laser intensity, and hence both peak LII and ELS will appear earlier in time. Hence, peak ELS intensity will then depend on both the sublimation process and the extent of mass loss and cannot be directly used for estimation of the mass loss due to the pre-heating laser pulse. As ELS is obtained from soot without preheating (1P) as well as with pre-heating(2P), the difference in signal may be utilized to obtain information about the influence of sublimation from the pre-heating pulse. By fitting the scattering signal obtained at a low fluence where sublimation is negligible, to the leading edge of all ELS signals (20%–50% of max ELS signal), which is a common method for estimations of coating mass using continuous-wave LII (CW-LII) (Gao et al. Citation2007), the ELS signal as a function of laser fluence can be predicted as if no sublimation would occur. The difference compared to the measurements in Gao et al. (Citation2007) is that the soot is exposed to a gaussian beam of nanosecond length, and not of microseconds.
In , a sequence of time-resolved scattering signals are shown (in orange) with a fitted signal (in blue), which should represent the scattering signal independent of sublimation. In , the fitted peak ELS signal is shown as a function of fluence for an OP6 case and as black markers (1P triangles and 2P circles). The signal trends hence show the peak ELS signal as it would appear if no sublimation would occur. As the scattering signal scales according to the approximate relative mass m after sublimation in percentage of the original mass can be estimated as the square-root of the ELS-ratio. In , the ELS trends for OP1 and OP6 soot is shown as a function of the pre-heating fluence. For OP1 soot, mass loss becomes significant at ∼0.18 J/cm2 (which corresponds to 0.08 J/cm2 at 532 nm) and is in relatively good agreement with the observed trends in the fluence curve analysis in Section 4.1. It can be seen that when pre-heated with 0.35 J/cm2 at 1064 nm (∼0.16 J/cm2 at 532 nm assuming ξ = .17), the mass after extensive pre-heating reached approximately 80% of the original mass.
Figure 5. In (a) a sequence of ELS signals (red) are shown along with the leading edge fit (blue) for estimating the ELS signal without the influence of sublimation. In (b) the evolution of the fitted signals is shown for 1P and 2P setups for an OP6 case using a pre-heating fluence of 0.20 J/cm2 as a function of LII fluence. The peak ELS signal is included in the figure for comparison (dots). Red stars show the estimated mass loss in relation to the fitted ELS ratio at the given LII fluence. In (c) the resulting estimates of the relative mass is shown for OP1 and OP6 soot as a function of fluence. For comparison, the result of Cenker et al. (Cenker and Roberts Citation2017) is included and the resulting mass loss from LII model simulations for OP1 soot.
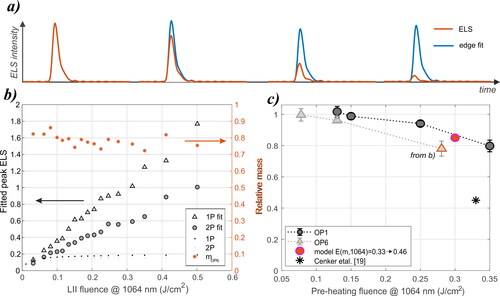
For OP6 soot, the scattering signal appears to decrease at even lower fluences than OP1 and lead to more efficient mass loss. In Cenker and Roberts (Citation2017) a loss down to 45% of its original mass was observed when pre-heated with 0.33 J/cm2 for less mature soot in a Santoro burner flame, and this data has also been included in . It is plausible that part of this signal loss is related to the evaporation of volatile hydrocarbons at temperatures below 1000 K. In addition to uncertainties in thermal annealing and optical properties, it should further be noted that elastic light scattering (ELS) is a complex process for fractal aggregates like soot and that the present analysis is based on a simple mass loss model. An extensive investigation of the scattering properties of OP1, OP6, and OP7 soot has been presented in Karlsson et al. (Citation2022).
Finally, by utilizing the LII model and by assuming that the E(m,1064) changes from 0.33 to 0.46 due to pre-heating, we may estimate the possible mass loss, by assuming that the evaporated species will not contribute to the LII signal. As the pre-heating of 0.13 J/cm2 at 532 nm would correspond to 0.3 J/cm2 at 1064 nm (by assuming ξ = 1.17), LII modeling (shown in ) shows that the mass would be approximately 85% of the original mass. The result is included in (pink circle), and as can be seen, the result agrees well with the results based on the ELS measurements of OP1 soot.
Figure 6. The fluence curves of 532–532 and 532–1064, with (2P) and without (1P) pre-heating are shown, where the fluence curve axis for the 532 nm case has been normalized to the 1064 nm case using the estimated i so that both excitation curves overlap. Also, all curves are normalized to the time-resolved LII signal at 50 ns after peak LII (and re-scaled to 1 for peak LII at 1064 nm) in order to obtain the difference in the fluence curve using 1064 nm (only LII; black and gray curve) and the fluence curve using 532 nm (LII + LIF; red curves).
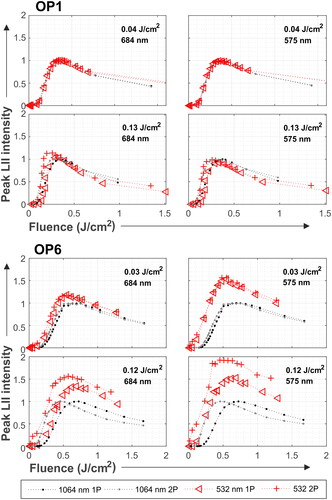
4.4. LIF interference
The LIF contribution to the LII signal can be overcome by considering the time-resolved LII signal at a time when the LIF contribution is considered negligible (as done in the ξ analysis) (Therssen et al. Citation2007; Cléon et al. Citation2011; Musikhin et al. Citation2019). Here however, the contribution of LIF will be estimated, with and without pre-heating prior to the LII measurement. The contribution of LIF at the time of peak LII is estimated by overlapping the time-resolved LII signal at 50 ns delay after peak LII, as done in Török, Mannazhi, and Bengtsson (Citation2021). In , these curves are shown, with (2P) and without (1P) pre-heating using the laser wavelength combinations of 532–532 and 532–1064 with low and high fluence pre-heating of OP1 and OP6 soot. As the 532–1064 combination shows the sole LII signal the curves (in black and gray) are normalized. The 532–532 shows the LII + LIF signal (in red) and hence, the difference between the curves (black/gray and red) can be considered as the LIF contribution. For OP1, it can be seen that regardless of the amount of pre-heating, the fluence curves obtained with 532 and 1064 nm do overlap well for both the 2P and 1P case, indicating that there is no significant LIF contribution, either with or without pre-heating.
For OP6 soot it can be seen that there is a considerable contribution from LIF, but the contribution does not change when pre-heating with 0.03 J/cm2. Using 0.12 J/cm2 pre-heating however, there is a change induced, showing an enhanced LIF contribution to the signal. The origin of this fluorescence signal is uncertain but may be related to volatiles released by the pre-heating pulse, which are then probed by the LII laser pulse. Another interesting observation is that the fluorescence is much stronger at 575 nm in comparison with 684 nm. This could indicate that the LIF signal originates from polycyclic aromatic hydrocarbons, which in sooting flames have a spectrum in the visible region after excitation at 532 nm. Also, this type of species has been detected in OP6 and OP7 soot after laser ablation in an SP-AMS (Malmborg et al. Citation2019).
The observations here can be discussed in relation to the changes in the fluence curves in , where an enhanced signal contribution is observed at low fluences, due to an enhanced LIF signal contribution as a result of substantial pre-heating of the young soot. It should however be noted that the difference between the curves does not represent the maximum LIF signal contribution in general, as the peaks of the LII and LIF signals do not necessarily overlap for all fluences.
The enhanced LIF signal contribution may appear unexpected, as thermal annealing will induce structural changes of the heated soot, which thereby obtain properties of more mature soot. But, as observed by Michelsen et al. (Citation2007) and Migliorini et al. (Citation2020), evaporated species and fragments from laser-heated soot particles may nucleate and form new particles in the size range of ∼10 nm. In Michelsen et al. (Citation2007) a new mode of particles was formed when soot was laser-heated at fluences higher than 0.12 J/cm2 at 532 nm. From transmission electron micrographs, it was observed that the small particles were at least partly of very low structural order. Also, in Migliorini et al. (Citation2020), laser-heated soot from an ethylene and a methane flame was investigated, showing characteristics of less mature soot with increasing laser heating. As the estimation of ξ was performed from extinction measurements, small particles and fluorescing species may have a non-negligible influence on ξ. Hence, we suggest that one possible explanation of the enhanced LIF contribution in our results may be related to the evaporation of carbon fragments which may fluoresce when exposed to laser radiation of 532 nm. In this work, it is however not investigated whether these species nucleate into small particles of low structural order or remain in gas-phase.
For OP1 soot, there was no indication of any significant LIF signal, which is not surprising as the organic part of this soot is very low (Török et al. Citation2018). Additionally, it was shown in Malmborg et al. (Citation2019) that laser heating of OP1 soot preferably led to small carbon fragments such as C, C2 and C3, which have no absorption and fluorescence characteristics in the visible spectral region.
4.5. Applicability
The present work can give guidance in the choice of optimal excitation wavelength using one pulse LII in a sooting environment with large variation in soot maturity. As young soot has a strong wavelength dependence and absorb more efficiently at shorter wavelengths, it may be preferred to choose a wavelength as short as possible but without inducing any fluorescence. It is true though that the influence of mature soot on the integrated LII signal in a sooting probe volume will be dominating. This is the case both due to its efficient absorption properties and its emissivity which both are directly proportional to E(m,λ), and as mature soot mass is often dominant in many combustion processes. Nevertheless, it is important to consider the influence of the choice of LII laser wavelength for efficient detection. Hence a laser wavelength around 700 nm for LII measurements would avoid creating significant fluorescence and simultaneously minimize the difference in absorption efficiency between different types of soot.
Double-pulse LII measurements has in the present investigation been used for a fundamental investigation of soot characteristics. However, based on the present results it could potentially be used as a soot diagnostic. As such the double-pulse method could be used to enhance the detectability of young poorly absorbing soot in sooty environments where not much mature soot is present, as the first pulse anneals the soot and increases its absorption efficiency thereby increasing the sensitivity of the detection using the second pulse. Depending on the type of LII study, it may however be important to consider the influence of pre-heating not just on the enhanced absorption, but also on the induced changes to the LIF signal and the thermal properties of the soot. We further speculate on the potential of detecting very young soot by double pulse LII, in order to get closer to the point of where soot is formed and one may differentiate between refractory soot and precursors such as PAHs.
5. Summary and conclusions
The response of mature and young soot to rapid (nanosecond) laser heating was investigated using a double-pulse LII setup. By pre-heating the different types of soot using the first laser pulse, LII measurements were done using either 532 or 1064 nm radiation. The use of two different wavelengths allowed for the estimation of the wavelength dependence of the processes occurring due to pre-heating. The novel findings presented in this work are listed below.
Pre-heating the soot altered the mature and young soot in different ways. While the mature soot exhibited an absorption enhancement, suggesting thermal annealing, mass loss was also prominent, influencing the peak LII intensity. For the young soot, modest pre-heating resulted in extensive enhancement of both the absorption efficiency of the soot and the peak LII intensity.
For OP1 and OP6 soot, the influence of pre-heating on the dispersion coefficient was estimated. A decrease was observed for both OP1 and OP6 as their values decreased from 1.17 to 1.03 and 1.67 to 1.42, respectively when heated with 0.13 and 0.12 J/cm2 at 532 nm. This change in absorption dependence is related to a more mature character of the soot after annealing.
From elastic laser scattering measurements, leading edge fitting was performed to estimate the mass loss due to pre-heating. The method agreed well with mass loss estimations from the LII model.
From analysis of the fluence curves based on the time-resolved signal at 50 ns after peak LII, the contribution of LIF at 532 nm could be estimated for OP1 and OP6 soot. Pre-heating the soot, induced an enhancement of the LIF contribution for the OP6 soot. We suggest, in alignment with other works, that vaporized carbon species from the soot particles are fluorescing when exposed to laser radiation of 532 nm. It is however not investigated whether this signal originates from gaseous species or newly nucleated particles.
Further, we can suggest the use of the double pulse technique as a method for increasing the detectability of young soot which absorbs poorly at the most common LII wavelength of 1064 nm. Also, the present study can give guidance in order to choose the optimum laser wavelength for LII for efficient soot detection related to the maturity of the soot.
Additional information
Funding
References
- Apicella, B., P. Pré, M. Alfè, A. Ciajolo, V. Gargiulo, C. Russo, A. Tregrossi, D. Deldique, and J. N. Rouzaud. 2015. Soot nanostructure evolution in premixed flames by High Resolution Electron Transmission Microscopy (HRTEM). Proc. Combust. Inst. 35 (2):1895–902. doi:10.1016/j.proci.2014.06.121.
- Apicella, B., P. Pré, J. N. Rouzaud, J. Abrahamson, R. L. V. Wal, A. Ciajolo, A. Tregrossi, and C. Russo. 2019. Laser-induced structural modifications of differently aged soot investigated by HRTEM. Combust. Flame 204:13–22. doi:10.1016/j.combustflame.2019.02.026.
- Bond, T. C., S. J. Doherty, D. W. Fahey, P. M. Forster, T. Berntsen, B. J. DeAngelo, M. G. Flanner, S. Ghan, B. Kärcher, D. Koch, et al. 2013. Bounding the role of black carbon in the climate system: A scientific assessment. J. Geophys. Res. Atmos. 118 (11):5380–552. doi:10.1002/jgrd.50171.
- Cenker, E., and W. L. Roberts. 2017. Quantitative effects of rapid heating on soot-particle sizing through analysis of two-pulse LII. Appl. Phys. B 123 (3):74. doi:10.1007/s00340-017-6653-7.
- Cléon, G., T. Amodeo, A. Faccinetto, and P. Desgroux. 2011. Laser induced incandescence determination of the ratio of the soot absorption functions at 532 nm and 1064 nm in the nucleation zone of a low pressure premixed sooting flame. Appl. Phys. B 104 (2):297–305. doi:10.1007/s00340-011-4372-z.
- De Iuliis, S., F. Migliorini, F. Cignoli, and G. Zizak. 2006. Peak soot temperature in laser-induced incandescence measurements. Appl. Phys. B 83 (3):397–402. doi:10.1007/s00340-006-2210-5.
- Gao, R. S., J. P. Schwarz, K. K. Kelly, D. W. Fahey, L. A. Watts, T. L. Thompson, J. R. Spackman, J. G. Slowik, E. S. Cross, J.-H. Han, et al. 2007. A novel method for estimating light-scattering properties of soot aerosols using a modified single-particle soot photometer. Aerosol Sci. Technol. 41 (2):125–35. doi:10.1080/02786820601118398.
- IPCC. 2013. Climate change 2013: The physical science basis. Contribution of Working Group I to the Fifth Assessment Report of the Intergovernmental Panel on Climate Change. Cambridge, United Kingdom and New York, NY, USA, Cambridge University Press.
- Janssen, N. A. H., M. E. Gerlofs-Nijland, T. Lanki, R. O. Salonen, F. Cassee, G. Hoek, P. Fischer, B. Brunekreef, and M. Krzyzanowski. 2012. Health effects of black carbon. Copenhagen, Denmark: WHO.
- Jing. 2009. "Mini-CAST soot generator." Accessed October, 2019. http://www.sootgenerator.com/.
- Johansson, K. O., F. E. Gabaly, P. E. Schrader, M. F. Campbell, and H. A. Michelsen. 2017. Evolution of maturity levels of the particle surface and bulk during soot growth and oxidation in a flame. Aerosol Sci. Technol. 51 (12):1333–44. doi:10.1080/02786826.2017.1355047.
- Karlsson, A., S. Török, A. Roth, and P.-E. Bengtsson. 2022. Numerical scattering simulations for estimating soot aggregate morphology from nephelometer scattering measurements. J. Aerosol Sci. 159:105828. doi:10.1016/j.jaerosci.2021.105828.
- Le, K. C., T. Pino, V. T. Pham, J. Henriksson, S. Tӧrӧk, and P.-E. Bengtsson. 2019. Raman spectroscopy of mini-CAST soot with various fractions of organic compounds: Structural characterization during heating treatment from 25 °C to 1000 °C. Combust. Flame 209:291–302. doi:10.1016/j.combustflame.2019.07.037.
- Liu, F., B. J. Stagg, D. R. Snelling, and G. J. Smallwood. 2006. Effects of primary soot particle size distribution on the temperature of soot particles heated by a nanosecond pulsed laser in an atmospheric laminar diffusion flame. Int. J. Heat Mass Transf. 49 (3–4):777–88. doi:10.1016/j.ijheatmasstransfer.2005.07.041.
- López-Yglesias, X., P. E. Schrader, and H. A. Michelsen. 2014. Soot maturity and absorption cross sections. J. Aerosol Sci. 75:43–64. doi:10.1016/j.jaerosci.2014.04.011.
- Malmborg, V. B., A. C. Eriksson, S. Török, Y. Zhang, K. Kling, J. Martinsson, E. C. Fortner, L. Gren, S. Kook, T. B. Onasch, et al. 2019. Relating aerosol mass spectra to composition and nanostructure of soot particles. Carbon 142:535–46. doi:10.1016/j.carbon.2018.10.072.
- Mansmann, R., T. Dreier, and C. Schulz. 2017. Performance of photomultipliers in the context of laser-induced incandescence. Appl. Opt. 56 (28):7849–60. doi:10.1364/AO.56.007849.
- Michelsen, H. A. 2017. Probing soot formation, chemical and physical evolution, and oxidation: A review of in situ diagnostic techniques and needs. Proc. Combust. Inst. 36 (1):717–35. doi:10.1016/j.proci.2016.08.027.
- Michelsen, H. A., M. B. Colket, P.-E. Bengtsson, A. D’Anna, P. Desgroux, B. S. Haynes, J. H. Miller, G. J. Nathan, H. Pitsch, and H. Wang. 2020. A Review of terminology used to describe soot formation and evolution under combustion and pyrolytic conditions. ACS Nano. 14 (10):12470–90. doi:10.1021/acsnano.0c06226.
- Michelsen, H. A., C. Schulz, G. J. Smallwood, and S. Will. 2015. Laser-induced incandescence: Particulate diagnostics for combustion, atmospheric, and industrial applications. Prog. Energy Combust. Sci. 51:2–48. doi:10.1016/j.pecs.2015.07.001.
- Michelsen, H. A., A. V. Tivanski, M. K. Gilles, L. H. van Poppel, M. A. Dansson, and P. R. Buseck. 2007. Particle formation from pulsed laser irradiation of soot aggregates studied with a scanning mobility particle sizer, a transmission electron microscope, and a scanning transmission X-ray microscope. Appl. Opt. 46 (6):959–77. doi:10.1364/ao.46.000959.
- Migliorini, F., S. De Iuliis, R. Dondè, M. Commodo, P. Minutolo, and A. D'Anna. 2020. Nanosecond laser irradiation of soot particles: Insights on structure and optical properties. Exp. Therm. Fluid Sci. 114:110064. doi:10.1016/j.expthermflusci.2020.110064.
- Musikhin, S. M. R., G. J. Smallwood, T. Dreier, K. J. Daun, and C. Schulz. 2019. Spectrally and temporally resolved LII interference emission in a laminar diffusion flame. Proceedings of Combustion Institute—Canadian Section.
- Olofsson, N.-E., J. Simonsson, S. Török, H. Bladh, and P.-E. Bengtsson. 2015. Evolution of properties for aging soot in premixed flat flames studied by laser-induced incandescence and elastic light scattering. Appl. Phys. B 119 (4):669–83. doi:10.1007/s00340-015-6067-3.
- Simonsson, J., N.-E. Olofsson, S. Török, P.-E. Bengtsson, and H. Bladh. 2015. Wavelength dependence of extinction in sooting flat premixed flames in the visible and near-infrared regimes. Appl. Phys. B 119 (4):657–67. doi:10.1007/s00340-015-6079-z.
- Sipkens, T., and K. Daun. 2017. Defining regimes and analytical expressions for fluence curves in pulsed laser heating of aerosolized nanoparticles. Opt. Express. 25 (5):5684–96. doi:10.1364/OE.25.005684.
- Therssen, E., Y. Bouvier, C. Schoemaecker-Moreau, X. Mercier, P. Desgroux, M. Ziskind, and C. Focsa. 2007. Determination of the ratio of soot refractive index function E(m) at the two wavelengths 532 and 1064 nm by laser induced incandescence. Appl. Phys. B 89 (2-3):417–27. doi:10.1007/s00340-007-2759-7.
- Török, S., V. B. Malmborg, J. Simonsson, A. Eriksson, J. Martinsson, M. Mannazhi, J. Pagels, and P.-E. Bengtsson. 2018. Investigation of the absorption Ångström exponent and its relation to physicochemical properties for mini-CAST soot. Aerosol Sci. Technol. 52 (7):757–67. doi:10.1080/02786826.2018.1457767.
- Török, S., M. Mannazhi, and P.-E. Bengtsson. 2021. Laser-induced incandescence (2λ and 2C) for estimating absorption efficiency of differently matured soot. Appl. Phys. B 127 (7):96. doi:10.1007/s00340-021-07638-1.
- Vander Wal, R. L., and M. Y. Choi. 1999. Pulsed laser heating of soot: Morphological changes. Carbon 37 (2):231–9. doi:10.1016/S0008-6223(98)00169-9.
- Vander Wal, R. L., T. M. Ticich, and A. B. Stephens. 1998. Optical and microscopy investigations of soot structure alterations by laser-induced incandescence. Appl. Phys. B 67 (1):115–23. doi:10.1007/s003400050483.
- Wan, K., X. Shi, and H. Wang. 2020. Quantum confinement and size resolved modeling of electronic and optical properties of small soot particles. Proceedings of the Combustion Institute. doi:10.1016/j.proci.2020.07.145.
- Witze, P. O., S. Hochgreb, D. Kayes, H. A. Michelsen, and C. R. Shaddix. 2001. Time-resolved laser-induced incandescence and laser elastic-scattering measurements in a propane diffusion flame. Appl. Opt. 40 (15):2443–52. doi:10.1364/ao.40.002443.