Abstract
Airborne murine coronavirus was assessed for its sensitivity to the vapors of chemicals commonly used to disinfect indoor surfaces. As a model for the chemical sensitivity of airborne SARS-CoV-2, the infectious potential of airborne Mouse Hepatitis Virus (MHV) was tracked in the presence of the following pure chemical vapors, each of which was below its permissible exposure limit (PEL) as regulated by the US National Institute of Occupational Safety and Health (NIOSH): <50 ppmv for glycol; <1ppmv for HOCl; and <1ppmv for H2O2. Along with its growth media, infectious MHV was aerosolized in a particle size distribution between 0.5 μm and 3.2 μm into a sealed, dark, 9 m3 chamber maintained at 22°C and 60% RH, including levels of chemical vapors maintained below their respective PELs. As judged by the TCID50 of airborne MHV collected by condensation, this airborne virus was rapidly inactivated by HOCl vapor, incurring an average of 99% infectious potential loss after 16 ± 4 min exposure to ≤0.2 ppmv HOCl. Airborne MHV responded with a 99% loss of infectious potential in 38 ± 10 min of exposure to ≤0.9 ppmv H2O2; and, a 99% loss of infectious potential in 33 ± 15 min when exposed to a gas-phase dipropylene glycol blend ≤20 ppmv as TVOC. The juxtaposition of quantitative RT-PCR and TCID50 responses suggest that even low levels of gas-phase HOCl exposures can damage the genome of airborne coronavirus in relatively short time frames (c.a. <5 mins).
EDITOR:
Introduction
During the latter half of 2020, a significant airborne infectious disease transmission route for coronavirus was recognized by academic consortia and the World Health Organization (WHO) (Morawska and Cao Citation2020; Zhang et al. Citation2020; World Health Organization (WHO) Citation2022). Accordingly, a generalized industrial hygiene response to the SARS-CoV-2 pandemic includes enhanced ventilation and filtration, as well as systematic surface disinfectant applications to high-touch surfaces in the built environment (American Society of Heating and Refrigeration and Air Conditioning Engineers (ASHRAE) Citation2021; Centers for Disease Control (CDC) Citation2021). Recent studies have reported the infectious potential decay of SARS-CoV-2 and its surrogates on a wide variety of common architectural materials. While the characteristic half-life of SARS agents reported by some of these studies is greater than 5 h on steel and non-porous plastics (van Doremalen et al. Citation2020), little evidence has been isolated to substantiate coronavirus fomites as a dominant part of human infection routes indoors (Pitol and Julian Citation2021; L. M. Casanova et al. Citation2010). Out of an abundance of caution, commercial building facility management practices continue to execute (enhanced) surface cleaning practices using conventional chemicals. In this context, there are a wide variety of commercial surface disinfectants, the most popular of which include glycols, citric acid, hypochlorous acid, or hydrogen peroxide stabilized as their active ingredient(s) (Q. Lin et al. Citation2020b). Several reports quantitatively describe the sensitivity of surface-associated mammalian virus to these types of oxidants and alcohols. Among them, Park and coworkers (Park et al. Citation2007) evaluated the inactivation response of surface-associated human norovirus to both liquid and fog application of hypochlorous acid in ranges between 20 and 200 ppm. A similar chamber-based approach was used to assess the virucidal activity of aerosolized hypochlorous acid against influenza immobilized on surfaces along with different model soiling agents that present significant chlorine demand (Hakim et al. Citation2015); these authors challenged avian influenza strains on porous surfaces meant to represent agricultural impoundments delivering relatively high free chlorine concentrations in a range between 50 and 200 ppm.
Under typical indoor conditions, common cleaning chemicals (i.e., HOCl, H2O2 and glycols) have vapor pressures in a range that forces them to rapidly partition from the surfaces to which they are applied as a liquid, into a gas-phase. While these chemical gases have been reported to have relatively poor abilities toward inactivating bacteriophage that are typically used as disinfection surrogates (e.g., MS2 and Phi6), understanding the inactivation response of airborne coronaviruses to low-level vapors of these disinfectants remains limited with respect to mammalian viruses (Wood, Richter, and Sunderman Citation2019). In this context, Dubuis et al. (Citation2021) described the response of airborne influenza virus to ozone at levels used for remedial purposes (>1 ppmv) in the built environment; under different humidity conditions this team tracked the persistence of influenza A when aerosolized in different formulations of respiratory fluids.
Previous studies have reported the response of surfaceborne and airborne viruses to relatively high concentrations of oxidant or alcohol in ranges well above 1 ppm. In response, we report how the infectious potential of an accepted model for the environmental persistence of SARS-CoV-2—the β-corona Mouse Hepatitis Virus (MHV)—responds to low-levels of common chemical vapors, in ranges and time frames that may likely be encountered during routine cleaning activities in the built environment. In all cases, the airborne MHV infectivity potential decay accelerated when exposed to gas-phase disinfectants, even when the disinfectant concentrations were well below the permissible exposure limits (PEL) established by regulatory agencies in the United States.
With the exception of surfactants, many common disinfectants that are periodically applied to high-touch surfaces in the built environment can rapidly partition into a gas-phase, leaving little residue after repeated applications. The classic application of diols (e.g., dipropylene glycol vapors) retains US EPA approval for the inactivation of airborne microbes, but a systematic diol dosing regimen, appropriate for emerging (viral) pathogens, has not been rigorously compiled for occupied spaces. Applying liquid disinfectants to high-touch surfaces in occupied spaces is widely accepted as a maintenance practice; yet, introducing gas-phase hypochlorous acid or hydrogen peroxide for airborne pathogen inactivation in occupied spaces does not have formal regulatory approval in the United States. Indeed, the gas-phase partitioning of these agents from the high-touch surfaces they are meant to clean can have indirect disinfection effects on airborne microbes, even at low levels (i.e., below their respective PELs). In this context, we report that trace levels of these gas-phase disinfectants encountered under common indoor environmental conditions significantly affects the infectious potential of a model airborne β-coronavirus (MHV) in time frames relevant to indoor ventilation rates.
Coronaviruses have been shown to lose their infectious potential in proportion to their time in direct contact with a disinfectant at a given concentration. This decay in virus infectivity potential has been cited for both contact with liquid disinfectant on surfaces and with gas-phase disinfectant in the air (Hulkower et al. Citation2011; L. M. Casanova et al. Citation2010; Schinköthe et al. Citation2021). In this context, gas-phase disinfectants can be more potent than their liquid aerosol-phase counterparts because inter-droplet interactions are not required. In the absence of chemical disinfectants, variables such as temperature, light flux, and relative humidity have all been isolated to characterize the persistence of airborne phage and mammalian viruses (Schuit et al. Citation2020; K. Lin et al. Citation2020a). Previous work characterizing airborne MHV response to these indoor environmental variables, as well as established disinfectant PELs, have been leveraged to characterize the effective dose-response of airborne MHV to different gas-phase disinfectants.
Here we used high-efficiency aerosol condensation capture to support observations of viral median tissue culture infectious dose (TCID50) and genomic assays (qRT-PCR) used to assess airborne MHV persistence. These measurements were combined to track airborne murine coronavirus as it aged in controlled indoor atmospheres, while exposed to low-levels of conventional gas-phase disinfectants. This study demonstrated that low-level gas-phase concentrations of different commercial disinfectant formulations, including dipropylene glycol, hydrogen peroxide and hypochlorous acid, can rapidly inactivate airborne murine coronavirus under conditions and characteristic times relevant to common indoor air exchange rates. In all cases observed here, the airborne MHV decay of both its infectivity potential and genetic materials was accelerated when exposed to each gas-phase disinfectant at levels well below their respective PELs.
Materials and methods
DBT cell propagation, MHV culture and quantitation
Mouse astrocytoma derived cells (DBT) (Environmental Biotechnology Group at the University of Michigan, MI, USA) were passaged in Dulbecco’s Modified Eagle’s Medium (DMEM, ATCC, Manassas, VA, USA) with 10% Fetal Bovine Serum (FBS, Sigma-Aldrich St. Louis, MO, USA) and 1% antibiotic/antimycotic (AA, Sigma-Aldrich, St. Louis, MO, USA) and maintained under sterile conditions at 37 °C in an incubator atmosphere at 85 kPa including 5% CO2. Nearly confluent DBT cells in DMEM with 2% FBS and 1% AA were inoculated with the β-coronavirus Mouse Hepatitis Virus ((MHV) ATCC VR-764) and harvested after 48 h by centrifuging cell debris (3 min @ 3000 g) and collecting the virus-containing supernatant. The DBT host cells used were passaged less than 25 generations in all cases. Aliquots from this MHV preparation were immediately transferred on ice for culturing (TCID50), genomic quantitation (RT-qPCR) and aerosolization into a full-scale environmentally controlled chamber as previously described (Nieto-Caballero et al. Citation2022). The infectious potential of MHV was assessed using a classic tissue culture syncytial assay adapted for aerosol analysis according to the methods developed by Caballero and coworkers reporting TCID50/m3 (lower detection limit, 5 x 102/m3). The quantities of MHV-associated genome were assessed using a widely accepted reverse transcriptase polymerase chain reaction protocol adapted for aerosol analysis according to the detailed method reported by (Nieto-Caballero et al. Citation2022). This viral RNA was quantified with reverse transcription-quantitative PCR (RT-qPCR), following its collection and immediate preservation in DNA/RNA Shield (Zymo Research Inc., Irvine, CA, USA). Upon their collection, viral RNA was immediately extracted from air chamber samples with the Quick-RNA (Viral Kit Zymo Research Inc., Irvine, CA, USA) and the first-strand cDNA synthesis was immediately performed on 10 µl of RNA extract from samples in parallel with negative extraction controls using a RevertAid RT kit (Thermo Fisher Scientific, Waltham, MA, USA) following the manufacturer’s protocol with 2 µl random hexamer primers and an initial incubation step for GC-rich templates. Controls containing no reverse transcriptase (RT-) were used to assess for amplification of extraneous DNA. Quantification of cDNA products via qPCR was performed as described by (Nieto-Caballero et al. Citation2022). Genomic quantitation of airborne virions are reported as an average target gene copy # MHV genes/m3 (lower detection limit, 10/m3). A RT-qPCR calibration standard and associated controls are provided in the supplementary materials (S1).
Aerosol generation and capture
A polydisperse MHV-containing aerosol from 8 mL of MHV virus culture (detailed above) was nebulized into a sealed 9 m3 (2.1 m x 2.1 m x 2.1 m), well-mixed, clean (HEPA-filtered and VOC-scrubbed) chamber with humidity and temperature-control (). Prior to each experiment, HEPA filtration was used to reduce the airborne particle concentrations in the chamber to <100 particles/L, while an activated carbon scrubber reduced the total volatile organic carbon (TVOC) concentration to <200 ppbv. The chamber was charged with MHV containing aerosol using a 6-jet Collison Nebulizer (CH Technologies, Westwood, NJ, USA) operating with a 5 mL precious fluids reservoir adaptor, under a pressure of 138 kPa of dry, HEPA-filtered, compressed breathing air until the chamber aerosol concentration of approximately 6 x 105 particles/L was achieved (0.5 μm < D50 < 3.5 μm). For each experiment conducted in this study, 10 min elapsed between the introduction of the first MHV aerosol and the initial aerosol sample to ensure a steady-state aerosol distribution under these operating conditions accounting for deposition (). Ninety-five percent of the MHV virus aerosolized was contained in the particle size fraction in the range of 0.5–3.5 μm as determined by RT-qPCR of MHV genes recovered by a multiple orifice uniform deposit impactor (MOUDI) as previously described (Nieto-Caballero et al. Citation2022). The sealed chamber air was scrubbed by HEPA filtration and activated carbon before and after each experiment, as verified by real-time optical particle counting (InstaScope, DetectionTek, Boulder, CO, USA) and TVOC measurements (DirectSense II, Graywolf Sensing, Calamity Bay, CA, USA). All experiments were conducted in the dark, with the temperature and humidity maintained at approximately 60% RH and 22°C. Airborne MHV was recovered using time-resolved condensation growth tube capture (CGTC) sampling (BioSpot-VIVAS, Aerosol Devices Inc., Fort Collins, CO, USA). Each aerosol sample was collected with CGTC over 5-minute intervals at a sample flow rate of 8 L/min, and collected into sterile sample wells filled with 2 mL DMEM with 2% FBS and 10% AA maintained at 12°C.
Figure 1. Schematic of the 9 m3 environmental chamber used in these studies. This chamber contained four low power (4w) floor fans to ensure a well-mixed environment, a relative humidity and temperature monitor, a humidifier, Collison six-jet nebulizers to aerosolize purified murine coronavirus cultures, an optical particle counter and an aerosol condensation growth tube collector.
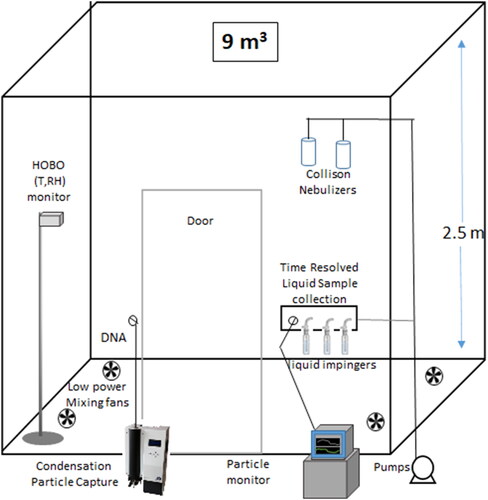
Figure 2. Particle size distribution of virus containing aerosol retained in the test chamber between 10 and 100 min of aerosol age in the presence of disinfectant vapors. (Inset) Aerosol concentration of optical diameters in range between 0.5 μm and 5 μm.
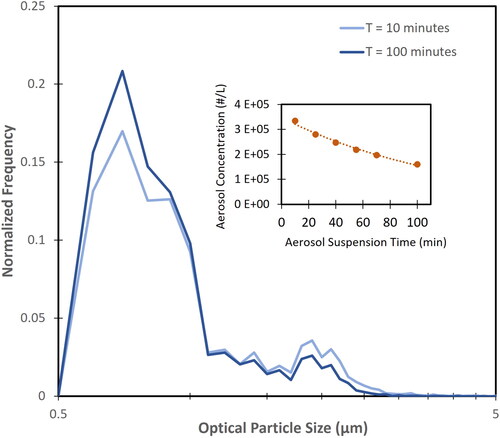
For each experiment, a baseline MHV aerosol sample was collected 10 min after the nebulization initiated, and prior to any disinfectant exposure. The MHV aerosol was suspended in the chamber for approximately 20 min prior to exposure to any gas-phase disinfectant. For the control studies (MHV aerosolized in the absence of any chemical vapors), samples were collected at the following intervals: 10, 20, 30, 50, 75, and 100 min. All control and disinfectant exposure experiments were conducted in triplicate.
Aerosol was collected by condensation along with accompanying disinfectant vapor, which is vented outside the device. While the condensation growth capture device used here is not designed to retain gaseous phase materials, some disinfectant vapor may partition into the collection reservoirs during the sampling process. To ensure that disinfectants were not present in the collection reservoir(s) during these studies, we measured free chlorine and peroxide in the collection wells with and without (sterile distilled water) the collection media present. Under no condition could we observe these oxidants present in collection media above their detection limit (c.a. 0.1 ppm) under this collection protocol. When collection reservoirs containing DMEM was purposely dosed with HOCl above detection limit, it was rapidly quenched (<1 min) by the DMEM components, suggesting that once airborne virus was recovered by condensation in these samplers, any potential oxidative disinfectant collected with it was rendered inactive.
Experimental design
A series of independent chamber experiments, for each of the following disinfectants was executed to observe airborne murine coronavirus response to trace levels of these oxidants in their vapor phase. In each disinfection scenario, triplicate chamber experiments were conducted with a minimum of three analytical replicates for each sample recovered in the experimental time series. Results are reported here as average ± standard deviation for airborne TCID50 and airborne MHV gene copy numbers. The gas-phase disinfectant concentrations are reported here as the maximum concentration encountered by the airborne virus. Each disinfectant was allowed to decay over the course of each experiment after it was introduced into the chamber 20 min after the MHV aerosol was nebulized. The 20 min between the MHV aerosol introduction and disinfectant exposure ensured that the aerosol was well mixed, and that a baseline MHV aerosol sample was collected before any disinfectant exposure commenced.
Gas-phase dipropylene glycol blend
A commercial disinfectant aerosol formulation containing a stabilized dipropylene glycol blend (“Citrace” [EPA Reg. No. 67619-29], Clorox, Oakland, CA, USA) was introduced into the chamber over 8 s using a pressurized spray that resulted in a chamber concentration of <20 ppmv (TVOC) that decayed over the course of the exposure experiments. The disinfect aerosol was introduced into the chamber air approximately 5 min after the baseline MHV aerosol sample was collected. It is worth noting that even though the maximum TVOC concentration reported here of <20 ppmv includes the active ingredients in the dipropylene glycol blend of 5.31% dipropylene glycol and 66.34% ethanol, the total TVOC concentration is far less than the dipropylene glycol PEL50 ppmv. Under this disinfectant exposure scenario, airborne MHV was sampled after exposure to the gas-phase glycol blend for approximately 6, 15, 23, and 35 min relative to the introduction of the disinfectant to the chamber. Immediately following their collection, the respective aerosol samples were immediately prepared for culturing (TCID50) and quantitative genomics (reverse transcriptase, qRT-PCR).
Gas-phase hypochlorous acid (HOCl)
A commercial aerosol formulation containing stabilized sodium hypochlorite (“Anywhere” [EPA Reg. No. 67619-42] Clorox, Oakland, CA, USA) was used to charge the chamber with gas-phase hypochlorous acid (HOCl). The disinfect was introduced into the chamber to a maximum HOCl concentration of 0.2 ppmv, which peaked approximately 7 min after the baseline MHV aerosol sample was collected (20 min after the MHV was introduced into the chamber), and allowed to naturally decay over the course of the exposure experiments. Approximately 150 ml of hypochlorous acid solution was introduced to the chamber by pipette and HOCl was allowed to partition into the gas phase as previously described (Biesiada et al. Citation2022). Airborne HOCl samples were collected using midget fritted impingers (Ace Glassware, Eight-Four, PA, USA) filled with 10 mL of 25 mM borate buffer operating at a flow rate of 1 L/min, and were taken concurrently alongside MHV samples in order to assess the HOCl concentrations that MHV was exposed to over the course of inactivation experiments. Airborne HOCl sample concentrations were then assessed by a fluorescent probe method calibrated for vapor phase HOCl described elsewhere (Biesiada et al. Citation2022). Under this disinfectant exposure scenario, airborne MHV was sampled after exposure to the gas-phase HOCl for approximately 2, 8, 31, and 46 min relative to the introduction of the disinfectant to the chamber. Immediately following their collection, the respective aerosol samples were immediately prepared for culturing (TCID50) and quantitative genomics (reverse transcriptase, qRT-PCR).
Gas-phase hydrogen peroxide (H2O2)
Consistent with the exposure experiments described above, approximately 15 min after the MHV aerosol was introduced into the chamber, and 8 min after the baseline MHV aerosol sample was collected, a 30% (w/w) hydrogen peroxide solution (H2O2, Interstate Chemical Company, Hermitage, PA, USA) was nebulized to a final gas-phase concentration of <0.9 ppmv H2O2. The H2O2 was nebulized using a 6-jet Collison nebulizer for 20 s with 138 kPa inlet pressure using dry, HEPA filtered, compressed breathing air and allowed to naturally decay in the chamber over the course of the experiment. Gas-phase hydrogen peroxide concentrations were measured every 60 s in real-time using a portable spectrochemical meter with a lower detection limit of 0.015 H2O2 ppmv (SC-8000, Riken Keiki, Tokyo, Japan). Under this disinfectant exposure scenario, airborne MHV was sampled after exposure to the gas-phase H2O2 for approximately 1, 8, 17, 25, and 33 min relative to the introduction of the disinfectant to the chamber. Immediately following their collection, the respective aerosol samples were immediately prepared for culturing (TCID50) and quantitative genomics (reverse transcriptase, qRT-PCR).
Results
Gas-phase dipropylene glycol blend effects
In response to an indoor atmosphere at 60% RH and 22°C containing a gas-phase glycol blend, significant decreases in the infectious potential of airborne MHV were observed after 10 min of exposure to no more than 20 ppmv glycol vapor (reported here as TVOC of glycol including its propellant and odorants), well below the glycol PEL of 50 ppmv. After less than 10 min of airborne exposure to the gas-phase glycol blend (<20 ppmv TVOC), the MHV TCID50 approached the detection limit of the assay which corresponded to a two-log reduction (99% inactivation) in 33 ± 15 min, while an otherwise identical control condition (no disinfectant present) experienced an averaged two-log reduction in its airborne infectious potential in 212 ± 120 min ( (control); B (dipropylene glycol blend)). As judged by TCID50, under the same glycol vapor exposure conditions, the averaged half-life of airborne MHV virus is approximately 5 ± 2 min; under otherwise identical environmental conditions in the absence of these vapors, the airborne MHV virus half-life was 32 ± 18 min. The airborne MHV gene copies recovered by CGTC after the associated exposure to gas-phase glycol blend demonstrated a decay to half of that recovered under control conditions. As judged by RT-qPCR, the averaged half-life of airborne MHV virus (target) genes exposed to gas-phase glycol blend (<20 ppmv TVOC) under these conditions is approximately 18 ± 2 min; under otherwise identical control condition in the absence of glycol vapors the half-life was 45 ± 4 min (). The decay rates for both MHV TCID50 and gene copies during exposure to gas-phase glycol blend are 5 and 2 times higher, respectively, than those calculated for control conditions ().
Figure 3. The average half-life and time for the two-log (99%) reduction of airborne MHV infectivity potential (TCID50) and gene copy numbers (RT-qPCR). (a) Control conditions represent the MHV response in the absence of any chemical treatment; (b) exposure conditions with gas-phase dipropylene glycol (<20 ppmv TVOC); (c) exposure conditions with HOCl vapor (<0.2 ppmv); (d) exposure conditions with H2O2 vapor (<0.9 ppmv). Bar heights represents the average and error bar presents the pooled standard deviation (n = 3 experiments each with analytical triplicates).
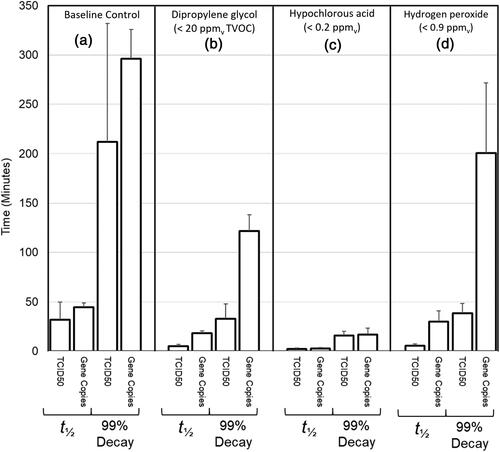
Figure 4. Airborne murine coronavirus (MHV) concentrations, as judged by TCID50 (o) and gene copy numbers qRT-PCR (▲), in an environmental chamber maintained at 60% RH and 22°C. (a) Control conditions represent the MHV response in the absence of any gas-phase chemical treatment; (b) exposure conditions with gas-phase dipropylene glycol (<20 ppmv TVOC); (c) exposure conditions with HOCl vapor (<0.2 ppmv); (d) exposure conditions with H2O2 vapor (<0.9 ppmv). Vertical lines (ǁ) represent termination of gas-phase disinfectant loading into the chamber. Declining horizontal lines are the regression slopes of independent exposure experiments used to calculate the average decay of triplicate experiments.
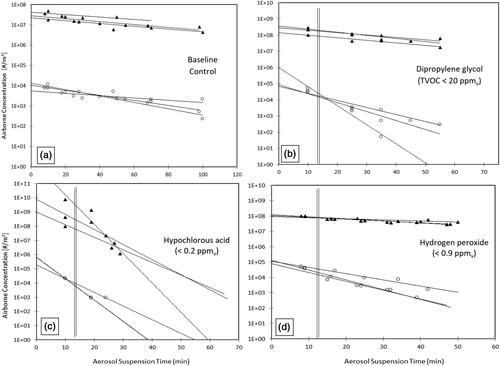
Gas-phase hypochlorous acid effects
In response to an indoor atmosphere at 60% RH and 22°C containing gas-phase hypochlorous acid (HOCl) at no more than 0.2 ppmv, below its PEL of 1 ppmv, significant decreases in the infectious potential of airborne MHV were observed after 2 min of exposure. After exposure to gas-phase HOCl, the airborne MHV TCID50 approached an averaged two-log reduction (99% inactivation) in 16 ± 4 min, while an otherwise identical control condition (no HOCl vapor) experienced an averaged two-log reduction in its airborne infectious potential in 212 ± 120 min ( (control); C (HOCl)). While the gas-phase HOCl levels varied during these exposure trials, at no time did the hypochlorous acid vapor concentration exceed 0.2 ppmv (ca. 40% the PEL).
As judged by TCID50, the averaged half-life of MHV aerosol exposed to low-levels of gas-phase HOCl under these conditions is approximately 2 ± 1 min; under otherwise identical control conditions, the half-life was 32 ± 18 min. The associated decay of airborne MHV gene copies recovered by CGTC was significantly different in the presence and absence of gas-phase HOCl. As judged by RT-qPCR, the averaged half-life of airborne MHV virus (target) genes exposed to low-levels of gas-phase HOCl under these conditions is on average 3 ± 1 min; under otherwise identical conditions in the absence of HOCl the half-life was 45 ± 4 min ( (control); C (HOCl)). The decay rates for both MHV TCID50 and gene copies during exposure to gas-phase HOCl was 12 and 19 times higher, respectively, than those determined for control conditions (, and ).
Table 1. The average half-life and 99% decay for each exposure condition. Respective decay times are reported as average ± SD (min).
Table 2. Averaged decay rate constant ± SD (min−1) or each exposure condition tabulated below graph.
Gas-phase hydrogen peroxide vapor effects
In response to an indoor atmosphere at 60% RH and 22°C containing gas-phase hydrogen peroxide levels at no more than <0.9 ppmv H2O2, below its PEL (1 ppmv), significant decreases in the infectious potential of airborne MHV were observed after less than 10 min of exposure. The MHV TCID50 results from replicate experiments conducted under these conditions indicate that the infectivity half-life of MHV aerosol occurs at 6 ± 1 min and an averaged two-log reduction (99% inactivation) is achieved in 38 ± 10 min. In otherwise identical control conditions (no H2O2), MHV aerosol experience an averaged the infectivity half-life of 32 ± 18 min and a two-log reduction in infectivity after 212 ± 120 min ( (control); D (H2O2)). The associated decay of airborne MHV gene copies recovered by CGTC, as judged by RT-qPCR, had an averaged half-life of 39 ± 14 min when exposed to low-levels of gas-phase H2O2, and a two-log decay of 258 ± 91 min, slightly less than those encountered under control conditions in the absence of H2O2 ( (control); D (H2O2)). The decay rates for both MHV TCID50 and gene copies during exposure to gas-phase H2O2 was 5 and 1.2 times higher, respectively, than those determined for control conditions ().
Discussion
Diols and oxidants are often used to clean and/or disinfect the furnishings and high-touch surfaces found in the built environment before, during and after their occupation (i.e., in schools, restaurants, gymnasiums, etc.). Residuals from common cleaning chemicals can persist in the built environment long after their initial application (Farmer et al. Citation2019). These cleaners include solutions of glycols, stabilized chlorine species, as well as stabilized peroxides. As distinct from fumigation activities, where relatively high concentrations of chemicals are applied for rapid indoor disinfection, the unavoidable partitioning of these chemicals from the surfaces they are routinely meant to clean often results in trace levels of their associated vapors in occupied spaces. Such “low-levels” are defined here as those vapor-phase concentrations below prescribed occupational health thresholds — specifically referred to as Permissible Exposure Limit (PEL) (Occupational Safety and Health Administration (OSHA) Citation2022) and/or Threshold Limit Values (TLV) (American Council of Government Industrial Hygienists (ACGIH) Citation2022).
While PEL and TLV thresholds are reported as concentrations, the associated exposure is an integrated product expressed through a time-weighted average (TWA), framed in hours, often corresponding to the length of conventional working days (8–10 h). When apportioning human activity as an indoor bioaerosol source, the juxtaposition of the infectivity half-life of an airborne virus, like a coronavirus (t1/2 ∼30 min), to the characteristic times typically associated with indoor air exchange rates is significant, where typical ventilation rates range 1 hr−1 < ACH < 4 hr−1 (e.g., 1 room full volume of air exchanged between 15 and 60 min).
Oxidative disinfectants and alcohols are often delivered to indoor surfaces (and air) using a broad array of different nebulizers that deliver dilute solutions of these chemicals. In practice, consumer nebulizers impart the energy required to aerosolize solutions of oxidants/alcohols using hand-actuated pumps, piezoelectric devices or simple electrified compressors that propel solution through critical orifices, some of which are purposely designed to impart fundamental charge to the microdroplets aerosolized. The resulting vapor phase concentrations associated with aerosolization of disinfectant solutions are unique to the local air quality conditions (i.e., temperature and RH) as well as the oxidant concentration, surface activity, ionic strength and particle size distribution of the aerosol delivered. In this study, we purposely isolated vapor phase concentrations as the exposure process variable, using a simple evaporative approach to ensure no disinfectant-containing aerosol was present.
As a model, we tracked the persistence of airborne murine coronavirus, an accepted surrogate for the pathogenic human coronavirus (SARS-CoV-2 (Körner et al. Citation2020)), under common indoor conditions (60% RH, 22 °C). In this context, results suggest that even low-levels of gas-phase constituents from cleaning, well below their PELs, can have a potent effect on airborne coronavirus infectivity and persistence in time frames relevant to cycles of human exposure indoors. Of the effects observed, gas-phase hypochlorous acid had the most marked impact on the physiology and infectious potential of airborne coronavirus, lowering its averaged half-life (TCID50) from more than 30 min (32 ± 18 min) to less than 3 min (2 ± 1 min), even with the protective effect of being micro-aerosolized in its own growth media .
As judged by aerosol TCID50 decay, both gas-phase glycol blend and hydrogen peroxide had significant effects on the airborne coronavirus half-life below their respective PELs; however, unlike hypochlorous acid, both peroxide and glycol vapors had lesser impacts on the ability to recover the airborne genomic material of this virus. Hypochlorous acid vapors appeared to damage the genetic material of the virus-containing aerosol as its specific RNA target could not be recovered and amplified (RT-qPCR) as effectively as the corresponding controls (). These results suggest low-levels of HOCl may at least impart internal physiologic damage associated with chlorine penetration of intact airborne virions. However, a specific inactivation mechanism could not be isolated here, acknowledging that a significant amount of subgenomic RNA may exist in these aerosol preparations (Alexandersen, Chamings, and Bhatta Citation2020) and that other physiologic biopolymers were not assayed for potential damage (e.g., capsid and receptor proteins or lipid envelope).
The airborne MHV virus TCID50 decay observed here, manifest in classic dose-response patterns associated with single-agent disinfection challenges of coronavirus previously observed on surfaces and in potable water (L. Casanova et al. Citation2009; L. M. Casanova et al. Citation2010; Viana Martins, Xavier, and Cobrado Citation2022). As judged by the average time required to achieve 99% inactivation, on average it took more than 200 min (212 ± 120 min) to realize two-log TCID50 reduction at 60% RH in the absence of any chemical vapors. Under otherwise identical environmental conditions, when chemical vapors were introduced to the chamber and controlled below their respective PELs, the time for two-log inactivation dropped significantly. In order of dose response, HOCl (<0.2 ppmv) was by far the most potent, significantly dropping the average time to two-log inactivation below 20 min (16 ± 4 min); glycol levels at or below 20 ppmv TVOC dropped the average time for two-log inactivation to just over 30 min (33 ± 15 min); and, hydrogen peroxide levels at or below 0.9 ppmv, dropped the average time for two-log inactivation to under 40 min (38 ± 10 min). The acute inactivation effects of gas-phase HOCl, clearly separated from diol and peroxide exposures, which were in significantly higher exposure windows than HOCl. These results suggest HOCl vapor is extremely effective at inactivating airborne MHV well below its PEL. Indeed, these “low” HOCl vapor levels likely extend to other common indoor environments where chlorine is routinely used for disinfection of surfaces and liquids, but partitions into an associating vapor (i.e., swimming pools, therapy pools, hot tubs, gymnasiums, etc.).
In summary, a mammalian coronavirus that is widely accepted as a model for the disinfection response of pathogenic human SARS-CoV-2 agents showed significant sensitivity to low-level vapors of common cleaning agents while suspended in micro-aerosols of its propagation media. Under these conditions, the characteristic times defining inactivation kinetics of this response (t1/2 half-life) were significantly less than characteristic ventilation times (i.e., air exchange rates) for high-occupancy indoor settings. Acknowledging that the coronavirus used here was co-aerosolized in its growth media (DMEM), we purposely used this experimental approach with a conservative strategy toward of promoting airborne virus persistence in the presence airborne disinfectants at low-levels. Other respiratory body fluids, artificial or actual, have been shown to confer protective effects to airborne mammalian viruses (Dubuis et al. Citation2021) in the presence of high levels of oxidative disinfectants (>1.5 ppmv O3). Although such respiratory fluids were not tested here, these results demonstrate the potential sensitivity of airborne coronavirus in co-aerosol with substantial protective potential (DMEM). Here we used regulatory PELs to frame indoor exposure conditions, the sources for which are realistic application scenarios for diols and oxidants. Thus, an indirect aerosol disinfection benefit may be realized following routine cleaning in some common indoor settings.
Supplemental Material
Download MS Word (42.9 KB)Acknowledgments
The authors would like to thank Dr. Julie Korak of the University of Colorado Boulder’s Environmental Engineering Program for her technical assistance with the Horiba fluorometer.
Disclosure statement
The authors acknowledge that the chemicals provided for this research were provided by the Clorox company, the Interstate Chemical Company and Zymo Research Chemicals.
References
- Alexandersen, S., A. Chamings, and T. R. Bhatta. 2020. SARS-CoV-2 Genomic and Subgenomic RNAs in diagnostic samples are not an indicator of active replication. Nat. Commun. 11:6059. doi:10.1038/s41467-020-19883-7.
- American Council of Government Industrial Hygienists (ACGIH). 2022. Annotated table of threshold limit values. https://www.acgih.org/TLV/.
- American Society of Heating, Refrigeration and Air Conditioning Engineers (ASHRAE). 2021. Epidemic task force core recommendations for reducing airborne infectious disease. https://www.ashrae.org/file%20library/technical%20resources/covid-19/core-recommendations-for-reducing-airborne-infectious-aerosol-exposure.pdf
- Biesiada, E., K. McCabe, O. Gomez, B. Volbers, and M. Hernandez. 2022. Adapting fluorescing imidazoline conjugates for low level quantitation of free airborne chlorine. J. Aerosol Sci. Forthcoming.
- Casanova, L. M., S. Jeon, W. A. Rutala, D. J. Weber, and M. Sobsey. 2010. Effects of air temperature and relative humidity on coronavirus survival on surfaces. Appl. Environ. Microbiol. 76 (9):2712–7. doi:10.1128/AEM.02291-09.
- Casanova, L., W. A. Rutala, D. J. Weber, and M. Sobsey. 2009. Survival of surrogate coronaviruses in water. Water Res. 43 (7):1893–8. doi:10.1016/j.watres.2009.02.002.
- Centers for Disease Control (CDC). 2021. CoVID 19 cleaning, disinfection and ventilation. https://www.cdc.gov/coronavirus/2019-ncov/community/disinfecting-building-facility.html.
- Dubuis, M.-E., É. Racine, J. M. Vyskocil, N. Turgeon, C. Tremblay, E. Mukawera, G. Boivin, N. Grandvaux, and C. Duchaine. 2021. Ozone inactivation of airborne influenza and lack of resistance of respiratory syncytial virus to aerosolization and sampling processes. PLoS One. 16 (7):e0253022. doi:10.1371/journal.pone.0253022.
- Farmer, D. K., M. E. Vance, J. P. D. Abbatt, A. Abeleira, M. R. Alves, C. Arata, E. Boedicker, S. Bourne, F. Cardoso-Saldaña, R. Corsi, et al. 2019. Overview of HOMEChem: House observations of microbial and environmental chemistry. Environ. Sci. Process. Impacts. 21 (8):1280–300. doi:10.1039/c9em00228f.
- Hakim, H., C. Thammakarn, A. Suguro, Y. Ishida, A. Kawamura, M. Tamura, K. Satoh, M. Tsujimura, T. Hasegawa, and K. Takehara. 2015. Evaluation of sprayed hypochlorous acid solutions for their virucidal activity against avian influenza virus through in vitro experiments. J. Veterinar. Med. Sci. 77 (2):211–5. doi:10.1292/jvms.14-0413.
- Hulkower, R. L., L. Casanova, W. A. Rutala, D. J. Weber, and M. Sobsey. 2011. Inactivation of surrogate coronaviruses on hard surfaces by health care germicides. Am. J. Infect. Control. 39 (5):401–7. doi:10.1016/j.ajic.2010.08.011.
- Körner, R. W., M. Majjouti, M. A. Alcazar, and E. Mahabir. 2020. Of mice and men: The coronavirus MHV and mouse models as a translational approach to understand SARS-CoV-2. Viruses. 12 (8):880. doi:10.3390/v12080880.
- Lin, K., R. Chase, C. R. Schulte, and L. C. Marr. 2020a. Survival of MS2 and Φ6 viruses in droplets as a function of relative humidity, pH, and salt, protein, and surfactant concentrations. PLoS ONE. 15 (12):e0243505–18. doi:10.1371/journal.pone.0243505.
- Lin, Q., J. Y. C. Lim, K. Xue, P. Yin, M. Yew, C. Owh, P. L. Chee, and J. X. Xian. 2020b. Sanitizing agents for virus inactivation and disinfection. View. 1 (2):e16. doi:10.1002/viw2.16.
- Morawska, L, and J. Cao. 2020. Airborne transmission of SARS-CoV-2: The world should face the reality. Environ. Int. 139:105730. doi:10.1016/j.envint.2020.105730.
- Nieto-Caballero, M., R. Davis, E. Huynh, E. Fuques, S. Ushijima, O. Gomez, M. Tolbert, and M. Hernandez. 2022. Carbohydrate vitrification in microaerosolized saliva associates with the humidity-dependent infectious potential of Airborne Murine Coronavirus. Proceedings of the National Academy of Sciences, NEXUS.
- Occupational Safety and Health Administration (OSHA). 2022. Annotated tables of permissible exposure limits (PEL). https://www.osha.gov/annotated-pels.
- Park, W. P., J. D. Boston, J. A. Kase, M. N. Sampson, and M. D. Sobsey. 2007. Evaluation of liquid- and fog-based application of sterilox hypochlorous acid solution for surface inactivation of human norovirus. Appl. Environ. Microbiol. 73 (14):4463–8. doi:10.1128/AEM.02839-06.
- Pitol, A. K, and T. R. Julian. 2021. Community transmission of SARS-CoV-2 by surfaces: Risks and risk reduction strategies. Environ. Sci. Technol. Lett. 8 (3):263–9. doi:10.1021/acs.estlett.0c00966.
- Schinköthe, J., H. A. Scheinemann, S. Diederich, H. Freese, M. Eschbaumer, and J. P. Teifke. 2021. Airborne disinfection by dry fogging efficiently inactivates limitations of commercial spore carriers. Environ. Microbiol. 87 (3):1–14.
- Schuit, M., S. Ratnesar-Shumate, J. Yolitz, G. Williams, W. Weaver, B. i. A. Green, D. Miller, M. Krause, K. Beck, S. Wood, et al. 2020. Airborne SARS-CoV-2 is rapidly inactivated by simulated sunlight. J. Infect. Dis. 222 (4):564–71. doi:10.1093/infdis/jiaa334.
- van Doremalen, N., T. Bushmaker, D. H. Morris, M. G. Holbrook, A. Gamble, B. N. Williamson, A. Tamin, J. L. Harcourt, N. J. Thornburg, S. I. Gerber, et al. 2020. aerosol and surface stability of SARS-CoV-2 as compared with SARS-CoV-1. N Engl. J. Med. 382 (16):1564–7. doi:10.1056/NEJMc2004973.
- Viana Martins, C. P., C. S. F. Xavier, and L. Cobrado. 2022. Disinfection methods against SARS-CoV-2: A systematic review. J. Hosp. Infect. 119:84–117. doi:10.1016/j.jhin.2021.07.014.
- Wood, J., W. Richter, and M. Sunderman. 2019. Evaluating low concentration hydrogen peroxide vapor for inactivation of ebola virus surrogates Phi6 and MS2 bacteriophage. US EPA Presentation Document. https://www.epa.gov/sites/default/files/2020-02/documents/wood_epa_revised.pdf.
- World Health Organization (WHO). 2022. Infection Prevention and Control in the Context of Coronavirus Disease (COVID-19): A Living Guideline. World Health Organization, WHO Health Emergencies - Infection Prevention and Control, Avenue Appia 20, 1211 Geneva 27, Switzerland. Document # WHO/2019-nCoV/ipc/guideline/2022.1
- Zhang, R., Y. Li, A. L. Zhang, Y. Wang, and M. J. Molina. 2020. Identifying airborne transmission as the dominant route for the spread of Covid-19. Proc. Natl. Acad. Sci. U S A. 117 (26):14857–63. doi:10.1073/pnas.2009637117.