Abstract
We present an advanced optical method to measure the phase function of circular intensity differential scattering (CIDS), i.e., the normalized Mueller matrix element -S14/S11, from individual single flowing through aerosol particles. Here, a 32-anode photomultiplier tube and its associated electronics, combined with an elliptical reflector, were used to record the scattering phase functions, when a particle were illuminated by a left-handed and a right-handed circular polarization laser beam around the focus of the reflector successively. The new design does not need lock-in amplifier, polarization modulator, and rotating goniometer as the traditional setup. It can reach a particle detection ability with a maximum rate of 50,000 particle/sec. CIDS phase functions from tryptophan particles, polystyrene latex microspheres, aggregates of Escherichia coli, Bacillus subtilis spores, Yersinia rohdei, and bacteriophage MS2 were measured, the results showed that this method has the ability to rapidly discriminate between single bioaerosol and non-bioaerosol particles.
EDITOR:
1. Introduction
Elastic light scattering has emerged as a powerful tool to characterize our surroundings including molecules, aerosols, cloud particles (e.g., dust or ice), and winds in atmosphere (Boreisho, Konyaev, and Orlov Citation2022; Mishchenko, Hovenier, and Travis Citation1999; Pan, Aptowicz, et al. Citation2022). The scattering patterns distributed with angles from a single particle or a group of particles can be used to retrieve the particle’s morphology, concentration, composition, and to detect and characterize bioaerosols (Pan, Aptowicz, et al. Citation2022; Kaye et al. Citation2000, Citation2005; Pan et al. Citation2003; Bickel and Stafford Citation1981). It has been known that the polarization properties are more sensitive to the molecular structures in a particle or the changes in a particle morphology (Boreisho, Konyaev, and Orlov Citation2022; Pan, Aptowicz, et al. Citation2022; Bickel and Stafford Citation1981; Piedra et al. Citation2019; Mishchenko et al. Citation2011; Gassó and Knobelspiesse Citation2022; Tanre et al. Citation2011; Le Gratiet, Marongiu, and Diaspro Citation2020; Stachelek et al. Citation2022; Cox and Barnett Citation2023; Sivaprakasam, Czege, and Eversole Citation2013; Pan, Kalume, et al. Citation2022; Diaspro et al. Citation1991; Hunt and Huffman Citation1973), and the calculated scattering patterns that contain polarization information demonstrated higher classification ability for different kinds of particles than the patterns with intensity distributions only (Piedra et al. Citation2019). The studies related to polarization scattering have been carried out, for example, in stand-off systems (Mishchenko et al. Citation2011; Gassó and Knobelspiesse Citation2022; Tanre et al. Citation2011), in polarized microscopic imaging from particles on a substrate (Le Gratiet, Marongiu, and Diaspro Citation2020; Stachelek et al. Citation2022; Cox and Barnett Citation2023), in particle flowing through systems (Sivaprakasam, Czege, and Eversole Citation2013; Pan, Kalume, et al. Citation2022), and in particle suspension (Diaspro et al. Citation1991; Hunt and Huffman Citation1973).
For an elastic light scattering process, two Stokes vectors with 4 elements are generally used to describe the spatial distributions of intensity and polarization of the illuminating and scattered light, and a Mueller matrix with 4 × 4 elements to characterize the scatterer’s properties. The Mueller matrix can be retrieved from the measurements of the scattered light, or directly evaluated by theoretical calculation from a well-defined scatterer/particle (s) (Sivaprakasam, Czege, and Eversole Citation2013; Pan, Kalume, et al. Citation2022; Diaspro et al. Citation1991; Hunt and Huffman Citation1973; Bustamante, Maestre, and Tinoco Citation1980; Ashraf, Ranjan, and Diaspro Citation2021). Within the Mueller matrix elements, S11 represents the scattering intensity, and other elements such as S12, S34, and S14 contain important linear and circular polarization information. While linear polarization has been widely used in remote-sensing, circular polarization is still in early development for particle characterization (Gassó and Knobelspiesse Citation2022; Tanre et al. Citation2011; Sivaprakasam, Czege, and Eversole Citation2013; Pan, Kalume, et al. Citation2022). Early studies found that S34 has high sensitivity in detecting the trivial changes in a cell, such as a mutation or even if the cell has lost its viability (Bickel and Stafford Citation1981; Bickel et al. Citation1976), and S14 can be used to distinguish particles that contain RNA or DNA, due to the helical structure of those molecules, or particles having chiral morphological structures from these without chirality (Sivaprakasam, Czege, and Eversole Citation2013; Pan, Kalume, et al. Citation2022; Diaspro et al. Citation1991; Hunt and Huffman Citation1973; Bustamante, Maestre, and Tinoco Citation1980; Ashraf, Ranjan, and Diaspro Citation2021; Bickel et al. Citation1976; Surkov et al. Citation2023). In general, the circular polarization property of a scatterer is measured by the circular intensity differential scattering (CIDS), which is the normalized element -S14/S11, or CIDS = (Ileft - Iright)/(Ileft + Iright), where Ileft and Iright are the measured intensities of the scattered light at a certain angle while the particle is illuminated by a left-handed and a right-handed circular polarization (LCP & RCP) light, respectively. For a symmetric particle or a large collection of randomly orientated, irregularly shaped particles, this CIDS will be zero in all scattering angles. However, for particles having helix or chiral structures, CIDS signal will be nonzero, which can be either greater or less than zero in certain scattering angles (Ashraf, Ranjan, and Diaspro Citation2021; Bickel et al. Citation1976; Bustamante, Maestre, and Tinoco Citation1980; Diaspro et al. Citation1991; Hunt and Huffman Citation1973; Pan, Kalume, et al. Citation2022; Patterson et al. Citation1986; Sivaprakasam, Czege, and Eversole Citation2013; Surkov et al. Citation2023).
Detection of helical structures from DNA or RNA molecules in biological samples started in the 1970s–1980s. There were only a few experiments reported that used a lock-in amplifier, a polarization modulator, and a rotation goniometer to record the CIDS phase function from a collection of particles, e.g., polystyrene latex (PSL) microspheres or cells in a suspension (Diasproet al. Citation1991; Hunt and Huffman Citation1973; Dorman and Maestre Citation1973; Gross et al. Citation1991; Maestre et al. Citation1982; Wells et al. Citation1986; Shapiro et al. Citation1994). Even though the CIDS measurement has been applied into microscopic imaging for extracting DNA chiral structures inside a cell nuclei, such as molecular conformation within complex biosystems, or monitoring fine structural changes of cells without using specific fluorescent labels (Cox and Barnett Citation2023; Le Gratiet, Marongiu, and Diaspro Citation2020; Stachelek et al. Citation2022; Marongiu et al. Citation2020), there has been little improvement in the method that would enable to detect and characterize bioaerosol particles, especially no report of rapid CIDS (or S14) measurement from single flowing through particles. The recently reported method (Pan, Kalume, et al. Citation2022) showed that the scattering phase functions can be measured from individual single flowing through aerosol particles via the illuminations of LCP or RCP light, respectively, then the CIDS phase function was obtained by subtracting the two scattering phase functions from different individual particles. This experimental arrangement works only when the particles population is homogeneous while the two scattering phase functions are measured, but CIDS could not be measured from a single individual particle, due to the limited speed of the switcher or shutter for turning ON/OFF the LCP or RCP lights, as well as the low frame rate of the ICCD detector. Here, we report an advanced method that can measure proper CIDS phase functions from individual single flowing through aerosol particles via LCP and RCP illuminations on the same particle, as it passes through the interrogation volume of the system. The key improvements are achieved by (1) using a 32-anode photomultiplier tube (32-PMT) replacing the ICCD and its associated electronics (Pan et al. Citation2001) to record the scattering phase functions, the data recording frame rate of the 32-PMT can reach 150,000/sec instead of 50/sec from the previous ICCD [16]; (2) aligning the LCP and RCP laser beams to be around the focus of the elliptical reflector and allowing the flowing through particle to interact with them successively in space without using the ON/OFF switcher or shutter. The new design upheld the advancements of the previous method that does not need a lock-in amplifier, a polarization modulator, and a goniometer for data recording, but can reach a particle detection rate as 50,000 particle/sec.
2. Experimental arrangement
shows the experimental arrangement for measuring CIDS phase function from individual single flowing through aerosol particles. and shows its side and top view respectively. The 532 nm LCP and RCP laser beams for particle illumination, with equal intensities, were generated using the same method as described in the previous article (Pan, Kalume, et al. Citation2022). However, the two beams were aligned into two parallel beams by the Glan-laser polarizer, but not combined into one beam again to overlap each other in the previous setup. The parallel LCP and RCP beams were arranged to be 40 µm above and 40 µm below the focal point of an elliptical reflector, which is a custom-design reflector (inset (c)) for collecting scattering light. The detail of the particle-laser interaction area around the focal point is enlarged as shown in inset (d). Here, the LCP beam, the RCP beam, the trigger beam, and the optical axis through the focal point of the reflector were within a vertical plane. The 80 µm gap between the LCP and RCP beams were aligned to allow the particle to travel 8 µs (particle moved ∼10 m/sec) to match the fastest data recording speed for two events using the corresponding electronics of the 32-PMT, but still in the acceptable pattern deform zone of the reflector for the two interaction spots of the particle with the two beams, more details of the time arrangement were further elaborated later.
Figure 1. (a) Side and (b) top view of the experimental setup for measuring CIDS phase functions from single individual flowing through aerosol particles; (c) the custom-design elliptical reflector for collecting scattering light; (d) enlargement of the particle-laser interaction area around the focal point of the reflector.
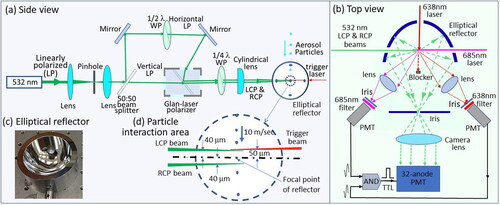
The test particles were generated by an ink jet aerosol generator (IJAG) and focused into a laminar flow with a moving speed of ∼10 m/sec and roughly 100 µm in diameter by a sheath nozzle. A cross-beam trigger scheme (Pan et al. Citation1999), which used two orthogonally arranged diode laser beams (638 nm and 685 nm) set 50 µm above the reflector’s focal point and two photomultiplier tubes (PMT) with a narrow band filter at 638 nm and 685 nm respectively, was used to monitor the presence of flowing through particles, then a logical TTL AND gate pulse was generated from the two PMTs to trigger the electronics of the 32-PMT for recording the two scattering phase functions. A single aerosol particle, which passes through the cross spot of the two diode lasers (50 µm × 50 µm), then was illuminated by the 532 nm LCP and RCP laser beam successively. The reflector projected both the forward and backward hemisphere angle-dependent scattering light simultaneously (θ = 0°–180°; ϕ = 180°] onto the 32-PMT (Hamamatsu Photonics H7260-20) for data recording. The particle-laser interaction volume [50 µm × 50 µm (area)×80 µm (height)] was within the limited pattern distortion range that was less than 50 µm from the focal point of the reflector [34]. Both the scattering phase functions via LCP and RCP illuminations were recorded with a 1 µs integration window. Here, the half wave plate, the quarter wave plate, and the Glan-laser polarizer were the key parts for producing the RCP and LCP beams in place of an polarization modulator. In order to get the scattering phase function from the recorded scattering pattern, the intensity-pixel (x, y) was converted into an intensity-angle (θ, φ) distribution in both forward and backward hemispheres by ray tracing into the spheric coordinate system as described previously (Pan, Kalume, et al. Citation2022; Fernandes et al. Citation2006; Walters et al. Citation2019). For every particle passing through the cross spot of the two diode lasers, the system was triggered to record two intensity-pixel distributions, which were corresponding to two scattering phase functions, when the particle was crossing the LCP and RCP beams successively with 8 µs interval.
NIST-traceable PSL microspheres were used to test and calibrate the system. The concentration of PSL in the IJAG cartridge was diluted enough to make sure there was only one microsphere or none contained in every droplet produced by the IJAG. For other test particles, the suspensions were prepared in a proper concentration to be dried into a solid particle at a size of ∼ 3 µm in diameter for CIDS measurements.
3. Experimental results and discussion
Six types of samples, tryptophan (Tryp), PSL microspheres, aggregates of Escherichia coli (E. coli), Bacillus subtilis spores (BS), Yersinia rohdei (Yr), and bacteriophage MS2, were aerosolized to test the system, and hundreds of CIDS phase functions from each sample were recorded. shows one typical scattering phase functions from single PSL, Tryp, BS, and MS2 particles using the illuminations of 532 nm LCP (dashed blue line) and RCP (solid red line) laser beam. Inset are their corresponding CIDS phase functions from normalized subtraction of the two scattering phase functions. These results demonstrated that the phase functions illuminated by RCP and LCP beam have almost indistinguishable features for PSL and Tryp, but noticeable differences for the bioaerosol particles BS and MS2. The absolute magnitude for CIDS is always less than 0.05 for PSL and Tryp with an even positive or negative peak distribution, while the CIDS signal is positive and negative in the forward and backward scattering direction, respectively, for BS and MS2 with much higher peak intensities.
Figure 2. Typical scattering phase functions from single tryptophan particle, PSL microspheres, B. subtilis, and MS2 aggregates using the illuminations of 532 nm LCP (dashed blue line) and RCP (solid red line) laser beams. Insets are their corresponding CIDS phase functions.
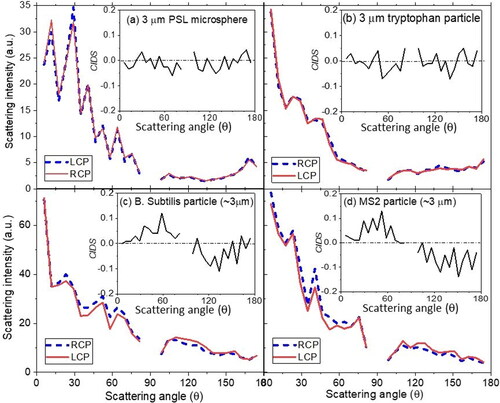
demonstrates 5 typical scattering phase functions, which are indicated by different colors, from 5 single particles of B. subtilis spores by the illuminations of 532 nm LCP (dashed line) and RCP (solid line) laser beams. They have significant variations in intensity and features with a maximum peak that varied from 50 to 170, due to the differences in particle size, shape and morphology, despite all of them being generated by the IJAG with a median size of 3 µm in diameter. However, the two scattering phase functions via LCP and RCP illuminations, which were measured within 8 µs time separation, were very similar for all 5 particles, indicating that the particle was in the limited pattern distortion range during the 8 µs travel time through the 80 µm space near the focal point of the reflector. It also indicates that the aerosol flow, after the nozzle, is laminar and moved in a near vertically straight trajectory. Although the scattering phase functions between the 5 particles are different, their corresponding CIDS phase functions are similar (, inset). These results demonstrate that single particle CIDS measurements can be made consistently and reliably with this system.
Figure 3. Typical scattering phase functions from 5 single particles (different colors) for B. subtilis spores using the illuminations of 532 nm LCP (dashed line) and RCP (solid line) laser beams. Inset shows their corresponding CIDS phase functions.
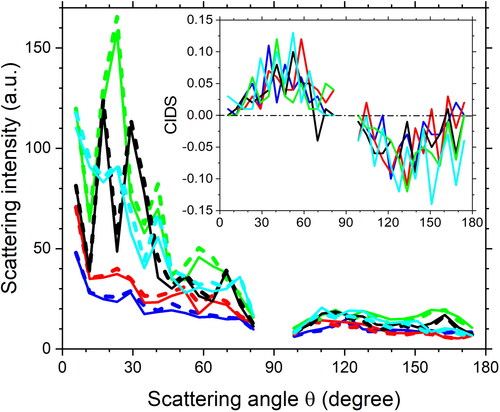
summarizes the CIDS phase functions from Tryp, PSL, E. coli, BS, Yr, and MS2 averaged of 200 single particles. Normalized differential phase functions were calculated from the scattering phase functions via RCP and LCP illuminations for every single particle. The results in demonstrated that the CIDS phase function in all angles is quite weak (peak |CIDS| < 0.01) for Tryp and PSL particles. Theoretically, they should be zero in all angles as they do not contain any helical structures in molecules or chiral morphology in the particles. In contrast, all bioaerosol particles (E. coli, BS, Yr, and MS2) showed relatively strong signals (peak |CIDS| > 0.035). The peak magnitudes from bioaerosols were at least 3 times stronger than those from non-bioaerosols. Meanwhile, all CIDS phase functions of the bioaerosol particles shared a similar curve with a positive value in the near forward direction (scattering angle 10°-60°) and a negative value in the near backward direction (110°–170°). The results verified that the CIDS phase functions have beneficial discriminating ability between bioaerosol and non-bioaerosol particles.
Figure 4. Averaged CIDS phase functions from 200 single tryptophan particles, polystyrene latex microspheres, aggregates of Escherichia coli, Bacillus subtilis spores, Yersinia rohdei, and bacteriophage MS2.
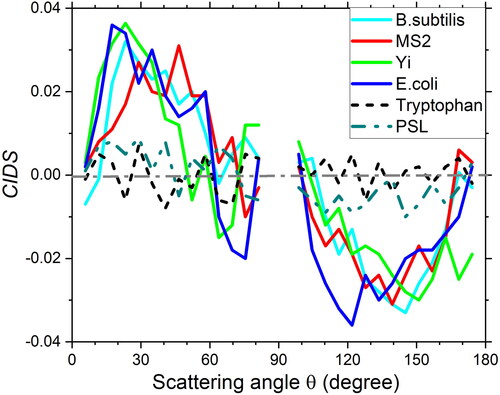
Traditionally, in order to measure a CIDS phase function from all scattering angles, a point photodetector on a rotating goniometer were used to record the signals at different angles and to complete a measurement with tens of minutes (Nothelfer, Foschum, and Kienle Citation2019). By introducing the elliptical reflector, the scattering light at different angles can be recorded simultaneously with a one- or two-dimensional (1-D or 2-D) photodetector such as the 2-D ICCD previously used (Pan, Kalume, et al. Citation2022) and the 1-D 32-PMT used here. To obtain the two scattering phase functions from exactly the same particle, as it is within the depth field around the focal point of the reflector while it is illuminated by the RCP and LCP beams, the displacement between the two positions for the same particle being illuminated during data acquisition should be less than 50 µm from the focal point of the reflector. This is critical to avoid the pattern distortion caused by the particle drifting away from the focal point [35]. To achieve this goal, we need an ultrafast shutter or switcher to turn-on and turn–off the RCP and/or LCP beam in a few µs, or a high frame rate detector.
Three ultrafast light shutters/switchers, which were an acousto-optic modulator (AOM), a Pockels cell polarization rotator, and a photo-elastic modulator (PEM), were tested. They were all fast enough (<1 µs) to turn ON/OFF the horizontal/vertical polarization beam or rotate the polarization to let an RCP and an LCP laser beam to interact with the particle successively or alternatively, they all were able to make CIDS measurement from exactly the same single particle possible. However, the circular purity and cross-talk of the RCP and LCP beam produced by the three switchers were far worse than that generated by the Glan-laser polarizer with an extinction ratio 5 × 105:1 in the present setup. In addition, these three switchers are both cumbersome and costly.
In general, the frame rate of a ICCD is <10 patterns/sec (in full image model), <100 spectra/sec (in 1-D spectral model), or <1000 spectra/sec (in a binned 1-D spectral model). This is not fast enough to record two phase functions as a particle is flowing through the focal volume of a reflector within 8 µs. The electronics (IQSP480, Vertilon Corp.) for recording data from the 32-PMT can reach 150,000 events/sec (a time interval of 6.667µs between two events), and this enabled to record two scattering phase functions from individual particles successively, via both RCP and LCP illuminations, while within the acceptable pattern distortion range of the elliptical reflector. Therefore, a quality CIDS phase function could obtain by subtracting two phase functions from the exact same particle. An IQSP580 has a frame rate of 390 KHz for the 32-PMT, which equals to 2.57 µsec interval between two events. However, this would make it hard to avoid the cross talk of the scatterings from the two parallel beams, so the IQSP480 was selected in the present experimental arrangement. We recorded the scattering phase functions using a 1 µs integration window, and 8 µs time separation to match the time for a particle to travel from the LCP to the RCP beam with an 80 µm gap between the two beams (particle moves ∼10 m/sec). It took less than 17 µs to finish a complete measurement, which included 1µs integration with LCP beam, 8µs for a particle to travel between two beams, 1µs integration with RCP beam, and 6.667 µs for data reading and saving until accepting next measurement. This reached a max measurement rate >1 particle/17 µs = 58,824 particles/sec > 50,000 particles/sec.
The 32-PMT has 32 pixels, each of them has an active aera of 0.8 mm (width) × 7mm (height), with a total active area of 25.6 mm × 7 mm to cover the scattering angle from 0° to 180° along the 25.6 mm dimension. The ICCD has 1024 × 1024 pixels, and an active area of 25.4 mm × 25.4 mm with a size 25.4 mm along the scattering angle direction. Therefore, the 32-PMT has a much lower angle resolution of the phase function, but a significantly higher detection sensitivity and faster measurement speed (<1ns response time) than the ICCD. This also allowed us to use a very low power (10 mW) 532 nm laser in the measurements, which is far less than 500 mW as required in the previous experiment (Pan, Kalume, et al. Citation2022). The replacement of the ICCD detector with a multiple-anode PMT not only made it possible to measure CIDS phase functions from individual single flowing through particles at high rate (>50,000 particles/sec), but also greatly reduced the physical dimensions for both the detector and the laser source, which is advantageous for future applications.
There are two key noise sources in the CIDS measurement. (1) Small differences in particle trajectories, since not all particles move perfectly along the vertical central line of the focusing nozzle. As a result, particles can be at slightly different positions with respect to the focal point of the reflector during particle-laser interactions, leading to random distortions of the phase functions during data acquisitions; (2) for most biological aerosols, the CIDS signal was estimated to be around 10−3 to 10−6, and the total polarization anisotropy of the illuminating beams should be controlled to be below 1% for a quality CIDS measurement (Wells et al. Citation1986). However, the smallest degree of ellipticity or anisotropy we could achieve was 1.2%, i.e., [(Imax-Imin)/(Imax+Imin)] × 100% = 1.2%, where Imax and Imin are the maximum and minimum intensities around all angles after a linear polarization analyzer. The anisotropy from other components might be bigger than 1%. These limited circular polarization purities and the above position fluctuations greatly impacted the accuracy of the CIDS measurement. Providentially, for some alighted chirality contained particles, the magnitude of the circular-polarization signal S14/S11 or S34/S11 can be greater than 10% (Bickel and Stafford Citation1996; Gassó and Knobelspiesse Citation2022; Surkov et al. Citation2023). This allowed the CIDS measurement possible here for a system with the anisotropy bigger than 1%.
In theory, a left-handed spiral particle will have a none-zone CIDS signal with a positive value in the near forward direction and a negative value in the near backward direction; while the right-handed spiral particle shall have a opposite values of the CIDS phase function (Surkov et al. Citation2023). It was noticed that the circular polarization signal of atmospheric aerosols can be observed only when the particles are aligned in the presence of an electromagnetic field rather than a randomly distributed aerosol assemble (Gassó and Knobelspiesse Citation2022). However, the CIDS phase functions () of bioaerosols observed in this paper all looked like left-handed spiral particle, but we cannot tell if these samples were all left-handed spiral particles in their DNA or RNA molecular structures, or they were symmetrically orientated by an electronic field, which was produced by a tiny current flow for warming the metal or resistor at the nozzle of the IJAG cartridge to vaporize suspension into a tiny steam bubble and to form a droplet in the aerosol generating process. Further study is needed to confirm these hypotheses.
4. Summary
We report an advanced optical method that is able to measure the circular intensity differential scattering (CIDS), i.e., the normalized Mueller matrix element -S14/S11 along all the scattering angles, from individual single flowing through aerosol particles at a maximum rate of 50,000 particles/sec. The two scattering phase functions for obtaining the CIDS phase function were measured from the same single particle when it was sequentially illuminated by a left and then a right circularly polarized laser beams with an 8 µs interval. The system does not require polarization modulator, moving goniometer, or lock-in amplifier, which were, in general, key parts or devices in the traditional arrangement. This new method presents noteworthy advantages in possible instrumentation for detecting bioaerosol particles from complex ambient environments.
Author agreement statement
We the undersigned declare that this manuscript is original, has not been published before and is not currently being considered for publication elsewhere. We confirm that the manuscript has been read and approved by all named authors and that there are no other persons who satisfied the criteria for authorship but are not listed. We further confirm that the order of authors listed in the manuscript has been approved by all of us. We understand that the Corresponding Author is the sole contact for the Editorial process. He/she is responsible for communicating with the other authors about progress, submissions of revisions and final approval of proofs.
Disclosure statement of competing interest
The authors declare the following financial interests/personal relationships which may be considered as potential competing interests: Yong-Le Pan and Joshua Santarpia report financial support was provided by the Defense Threat Reduction Agency (DTRA).
Additional information
Funding
References
- Ashraf, M. W., R. Ranjan, and A. Diaspro. 2021. Circular intensity differential scattering of light to characterize the coronavirus particles. J. Opt. Soc. Am. B 38 (5):1702–9. doi:10.1364/JOSAB.422646.
- Bickel, W. S., and M. E. Stafford. 1981. Polarized light scattering from biological systems: A technique for cell differentiation. J. Biol. Phys. 9 (2):53–66. doi:10.1007/BF01987283.
- Bickel, W. S., and M. E. Stafford. 1996. Polarized scattered light as a probe for structure and change in bioparticles. In Ultrasensitive biochemical diagnostics, Vol. 2680, ed. G. E. Cohn, S. A. Soper, and S. H. W. Chen, 4–15. New York: SPIE.
- Bickel, W. S., J. F. Davidson, D. R. Huffman, and R. Kilkson. 1976. Application of polarization effects in light-scattering - new biophysical tool. Proc. Natl. Acad. Sci. U S A 73 (2) (2):486–90. doi:10.1073/pnas.73.2.486.
- Boreisho, A. S., M. A. Konyaev, and A. E. Orlov. 2022. Measuring the atmospheric boundary layer parameters with a coherent doppler lidar. Russ. Meteorol. Hydrol. 47 (12):931–7. doi:10.3103/S1068373922120044.
- Bustamante, C., M. F. Maestre, and I. Tinoco. 1980. Circular intensity differential scattering of light by helical structures.1. Theory. J. Chem. Phys. 73 (9):4273–81. doi:10.1063/1.440709.
- Cox, D. M., and S. A. Barnett. 2023. A method for time-resolved characterization of polarization-induced solid oxide cell microstructure evolution. J. Electrochem. Soc. 170 (2):024514. doi:10.1149/1945-7111/acbb30.
- Diaspro, A., M. Bertolotto, L. Vergani, and C. Nicolini. 1991. Polarized-light scattering of nucleosomes and polynucleosomes - insitu and invitro studies. IEEE Trans. Biomed. Eng. 38 (7):670–8. doi:10.1109/10.83568.
- Dorman, B. P., and M. F. Maestre. 1973. Experimental differential light-scattering correction to circular-dichroism of bacteriophage t2. Proc. Natl. Acad. Sci. U S A 70 (1):255–9. doi:10.1073/pnas.70.1.255.
- Fernandes, G. E., Y. L. Pan, R. K. Chang, K. Aptowicz, and R. G. Pinnick. 2006. Simultaneous forward- and backward-hemisphere elastic-light-scattering patterns of respirable-size aerosols. Opt. Lett. 31 (20):3034–6. doi:10.1364/ol.31.003034.
- Gassó, S., and K. D. Knobelspiesse. 2022. Circular polarization in atmospheric aerosols. Atmos. Chem. Phys. 22 (20):13581–605. doi:10.5194/acp-22-13581-2022.
- Gross, C. T., H. Salamon, A. J. Hunt, R. I. Macey, F. Orme, and A. T. Quintanilha. 1991. Hemoglobin polymerization in sickle cells studied by circular polarized-light scattering. Biochim. Biophys. Acta 1079 (2):152–60. doi:10.1016/0167-4838(91)90120-o.
- Hunt, A. J., and D. R. Huffman. 1973. New polarization-modulated light-scattering instrument. Rev. Sci. Instrum. 44 (12):1753–62. doi:10.1063/1.1686049.
- Kaye, P. H., J. E. Barton, E. Hirst, and J. M. Clark. 2000. Simultaneous light scattering and intrinsic fluorescence measurement for the classification of airborne particles. Appl. Opt. 39 (21):3738–45. doi:10.1364/ao.39.003738.
- Kaye, P. H., W. R. Stanley, E. Hirst, E. V. Foot, K. L. Baxter, and S. J. Barrington. 2005. Single particle multichannel bio-aerosol fluorescence sensor. Opt. Express. 13 (10):3583–93. doi:10.1364/opex.13.003583.
- Le Gratiet, A., R. Marongiu, and A. Diaspro. 2020. Circular intensity differential scattering for label-free chromatin characterization: A review for optical microscopy. Polymers (Basel) 12 (10):2428. doi:10.3390/polym12102428.
- Maestre, M. F., C. Bustamante, T. L. Hayes, J. A. Subirana, and I. Tinoco. 1982. Differential scattering of circularly polarized-light by the helical sperm head from the octopus Eledone-cirrhosa. Nature 298 (5876):773–4. doi:10.1038/298773a0.
- Marongiu, R., A. Le Gratiet, L. Pesce, P. Bianchini, and A. Diaspro. 2020. ExCIDS: A combined approach coupling expansion microscopy (ExM) and circular intensity differential scattering (CIDS) for chromatin-DNA imaging. OSA Continuum 3 (7):1770–80. doi:10.1364/OSAC.388868.
- Mishchenko, M. I., J. Hovenier, and L. D. Travis, eds. 1999. Light scattering by nonspherical particles: Theory, measurements, and applications. 720. Washington, DC: Academic Press.
- Mishchenko, M. I., Y. S. Yatskiv, V. K. Rosenbush, and G. Videen, eds. 2011. Polarimetric detection, characterization and remote sensing. In Nato science for peace and security series c: Environmental security, 174–202. The Netharlands: Springer Dordrecht.
- Nothelfer, S., F. Foschum, and A. Kienle. 2019. Goniometer for determination of the spectrally resolved scattering phase function of suspended particles. Rev. Sci. Instrum. 90 (8):083110–22.
- Pan, Y. L., A. Kalume, J. Arnold, L. Beresnev, C. J. Wang, D. N. Rivera, K. K. Crown, and J. Santarpia. 2022. Measurement of circular intensity differential scattering (CIDS) from single airborne aerosol particles for bioaerosol detection and identification. Opt. Express. 30 (2):1442–51. doi:10.1364/OE.448288.
- Pan, Y. L., K. Aptowicz, J. Arnold, S. Cheng, A. Kalume, P. Piedra, C. J. Wang, J. Santarpia, and G. Videen. 2022. Review of elastic light scattering from single aerosol particles and application in bioaerosol detection. J. Quantum Spectrosc. Radiat. Transfer 279:108076–89.
- Pan, Y. L., K. B. Aptowicz, R. K. Chang, M. Hart, and J. D. Eversole. 2003. Characterizing and monitoring respiratory aerosols by light scattering. Opt. Lett. 28 (8):589–91. doi:10.1364/ol.28.000589.
- Pan, Y. L., P. Cobler, S. Rhodes, A. Potter, T. Chou, S. Holler, R. K. Chang, R. G. Pinnick, and J. P. Wolf. 2001. High-speed, high-sensitivity aerosol fluorescence spectrum detection using a 32-anode photomultiplier tube detector. Rev. Sci. Instrum. 72 (3):1831–6. doi:10.1063/1.1344179.
- Pan, Y. L., S. Holler, R. K. Chang, S. C. Hill, R. G. Pinnick, S. Niles, and J. R. Bottiger. 1999. Single-shot fluorescence spectra of individual micrometer-sized bioaerosols illuminated by a 351- or a 266-nm ultraviolet laser. Opt. Lett. 24 (2):116–8. doi:10.1364/ol.24.000116.
- Patterson, C. W., S. B. Singham, G. C. Salzman, and C. Bustamante. 1986. Circular intensity differential scattering of light by hierarchical molecular-structures. J. Chem. Phys. 84 (3):1916–21. doi:10.1063/1.450441.
- Piedra, P., A. Kalume, E. Zubko, D. Mackowski, Y. L. Pan, and G. Videen. 2019. Particle-shape classification using light scattering: An exercise in deep learning. J. Quantum Spectrosc. Radiat. Transfer 231:140–56. doi:10.1016/j.jqsrt.2019.04.013.
- Shapiro, D. B., M. F. Maestre, W. M. McClain, P. G. Hull, Y. Shi, M. S. Quinby-Hunt, J. E. Hearst, and A. J. Hunt. 1994. Determination of the average orientation of DNA in the octopus sperm eledone-cirrhossa through polarized-light scattering. Appl. Opt. 33 (24):5733–44. doi:10.1364/AO.33.005733.
- Sivaprakasam, V., J. Czege, and J. Eversole. 2013. Wavelength resolved polarized elastic scatter measurements from micron-sized single particles. In SPIE Proceedings. doi:10.1117/12.2016398.
- Stachelek, P., L. MacKenzie, D. Parker, and R. Pal. 2022. Circularly polarised luminescence laser scanning confocal microscopy to study live cell chiral molecular interactions. Nat. Commun. 13 (1):553. doi:10.1038/s41467-022-28220-z.
- Surkov, Y., Y. Shkuratov, Y. L. Pan, A. Kalume, J. Santarpia, E. Zubko, Y. X. Hu, and G. Videen. 2023. Light scattering from spiral particles. J. Quantum Spectrosc. Radiat. Transfer 298:108494–501. doi:10.1016/j.jqsrt.2023.108494.
- Tanre, D., F. M. Breon, J. L. Deuze, O. Dubovik, F. Ducos, P. Francois, P. Goloub, M. Herman, A. Lifermann, and F. Waquet. 2011. Remote sensing of aerosols by using polarized, directional and spectral measurements within the a-train: The parasol mission. Atmos. Meas. Tech. 4 (7):1383–95. doi:10.5194/amt-4-1383-2011.
- Walters, S., J. Zallie, G. Seymour, Y. L. Pan, G. Videen, and K. B. Aptowicz. 2019. Characterizing the size and absorption of single nonspherical aerosol particles from angularly-resolved elastic light scattering. J. Quantum Spectrosc. Radiat. Transfer 224:439–44. doi:10.1016/j.jqsrt.2018.12.005.
- Wells, K. S., D. A. Beach, D. Keller, and C. Bustamante. 1986. An analysis of circular intensity differential scattering measurements - studies on the sperm cell of eledone-cirrhosa. Biopolymers 25 (11):2043–64. doi:10.1002/bip.360251103.