Abstract
Exposure to fungal aerosols is of concern in indoor environments. However, sampling limitations have previously made it difficult to assess exposures accurately, especially long-term exposures. A prototype personal aerosol sampler, based on cyclone principles and using a 1.5 ml microcentrifuge tube as a particle collection receptacle has been designed and fabricated. Collection efficiency for aerosol particles in the size range of fungal spores has been evaluated for different types of microcentrifuge tubes, together with the effect of a polyethylene glycol coating on the inside of the tube and the effect of adding water to the tube. Monodisperse, fluorescently tagged polymer microspheres with median diameters of 0.5, 1, 2, 3, 6, 11, and 16 μm were used to evaluate sampler performance with particle diameter. The microcentrifuge-tube sampler was tested at flow rates of 2 and 4 liters per minute (l/min). Experimental results indicate that the microcentrifuge-tube sampler has an aspiration efficiency of 100% in calm air for particles up to 16 μm. At 4 l/min, the microcentrifuge-tube sampler is able to collect nearly 100% of particles greater than 3 μm and > 90% of particles between 2.5 and 3 μm. The 50% cutoff size is 1.5 μm. The performance of the sampler did not vary with the different brands of tubes tested or with the presence or absence of a coating on the tube surface. Furthermore, the addition of water to the tube resulted in a slight increase in collection efficiency. A sampling time of 5 h was feasible at 45–50% relative humidity before evaporation led to significant water loss.
The cutoff size of 1.5 μm is comparable to many commercially available bioaerosol samplers. Besides being easy to use, simple to fabricate, and inexpensive, this novel sampler has several advantages over conventional samplers: long-term samples are possible (the limitation of impaction methods); there is no sample transfer loss since the transfer step has been eliminated (the limitation of filter cassettes); laboratory analyses are not dependent solely upon a single analysis method (the limitation of impaction methods), and there is no sampler adherence loss (the limitation of trying to wash microorganisms from filters). In addition, use of the sampler would be applicable in a variety of occupational settings from low bioaerosol concentrations (i.e., indoor environments) to high bioaerosol concentrations (i.e., agricultural setting) by varying sampling time periods and using sensitive analytical methods.
INTRODUCTION
Occupational exposure to microbiological agents is of concern in a number of workplaces ranging from schools and offices with indoor air quality problems to agricultural poultry farms with high levels of diverse microbial species. Health effects of exposures to microbiological agents include allergic reactions, toxic effects, and infectious diseases. Fungi are of particular concern. Many different environments have the potential to favor fungal growth, especially when moisture levels are high. Exposure to fungal spores is a recognized asthma risk factor in indoor environments (CitationNeas et al. 1996; CitationDelfino et al. 1997). However, in general the deficiencies of current exposure assessment techniques have made it difficult to document a causal link between fungal exposure and health effects (CitationRao et al. 1996).
Conventional approaches to detecting and quantifying fungal bioaerosols often use short-term area grab samples. These are not time discriminant and are rarely representative of personal exposures. Temporal and spatial variations in airborne fungal concentrations and populations can affect collection of an appropriate exposure sample (CitationVerhoeff and Burge 1997). Fungal spores are often aerosolized in blooms that depend on environmental conditions (e.g., temperature and relative humidity) and physical disturbance. Short-term (e.g., less than 15 min) sampling using an impactor-type device is not likely to characterize the whole work period. Filter or impinger collection over the whole work period is another option. However, spores may not survive the longer sampling times and are thus difficult to analyze with culture-based monitoring techniques. In addition, filter and impinger collection methods have higher limits of detection than an impactor-type device, which may be an issue in relatively low fungal concentration situations such as non industrial locales (CitationAmerican Industrial Hygiene Association 1996).
Laboratory analysis of collected samples can also be an issue in accurately assessing exposures. Generally, only a fraction of the recovered fungi are culturable. For example, certain fungal species may only grow on specialized media (CitationMacher 2001). In addition, the health effects from exposures may be independent of the culturability and even viability of the fungi. Fungi have allergenic and toxic potential (CitationVerhoeff and Burge 1997). This makes data interpretation and health effects correlation problematic. Spore traps enable direct spore collection onto microscope slides, and their subsequent identification and quantification overcomes some of the issues associated with culture-based analysis (CitationMacher et al. 1995). Both culturable and nonculturable spores are accounted for, and time-sensitive samples can be collected in spore traps. However, true identification of the majority of fungal species still requires culturing. In addition, the expertise, expense, and time that spore counting entails are out of the realm of large-scale epidemiology studies.
Recent attention paid to biological warfare agents, SARS (severe acute respiratory syndrome) epidemics, and indoor “toxic” molds has revealed a need for highly specific and sensitive molecular techniques, such as enzyme-linked immunosorbent assays (ELISA) and polymerase chain reactions (PCR), in detecting microorganisms. A number of researchers have developed techniques for detecting specific or “total” indoor fungal species (CitationHaugland et al. 1999; CitationZhou et al. 2000; CitationCruz-Perez et al. 2001; CitationSchmechel et al. 2003; CitationChen et al. 2002). The progress made in these analytical techniques has the potential to permit accurate, reproducible, and quantitative measurement for fungal species in the samples. However, current air sampling methodologies are not amenable to these advanced analytical techniques.
The goal of this study was to develop a personal bioaerosol sampler that allows sample analysis without the need for a sample transfer step. It was the intention that this sampler would be applicable in a variety of occupational settings, from low bioaerosol concentrations (i.e., indoor environments) to high bioaerosol concentrations (i.e., agricultural setting), by varying sampling time periods and using sensitive analytical methods. Such an approach would allow industrial hygienists and field researchers to collect breathing zone samples from workers, biologists to use molecular assays for fungal quantification, and public health specialists to develop and implement control strategies and assess their effectiveness of intervention.
SAMPLER DESIGN AND PRELIMINARY RESULTS
The following criteria were considered for a practical bioaerosol sampler to be used in the field:
• | small, simple, and inexpensive; | ||||
• | easy to fabricate and use with a disposable collection medium; | ||||
• | able to be used for personal as well as area sampling; | ||||
• | appropriate for both short-term (15 min) and long-term (8 hr) sampling; | ||||
• | applicable in a variety of occupational settings from low (i.e., indoor environments) to high bioaerosol concentrations (i.e., agricultural setting); | ||||
• | high aspiration efficiency through the sampler's inlet and collection efficiency on the collection medium, for microbes of interest; | ||||
• | simple sample preparation for analysis with minimal extraction and transfer loss; and | ||||
• | suitable for both conventional counting methods and advanced molecular analyses. |
There is a lack of sampling devices that are capable of fulfilling these requirements. Impaction methods are commonly limited to short-term grab sampling rather than a whole work-shift assessments, due to potential overloading and particle re-entrainment. In addition, they depend solely upon a single analysis method such as culture or spore counts. Although the use of filters (e.g., in 37 mm filter cassettes) has been used for long-term sampling, studies have shown that efficient filter extraction of fungal spores is not only dependent upon filter medium and extraction methods, but also fungal species (CitationSchmechel et al. 2003). There are no standardized filter sampling and extraction methods for fungi that are ideal for all species of fungi.
Use of Microcentrifuge Tubes as the Collection Receptacle
In designing a new bioaerosol sampler, several types of collection receptacles were considered. The criteria for selection were effective microbial deposit, minimal loss during sample preparation, low cost, and ease of operation. Microcentrifuge tubes are widely used in molecular assays for sonicating, homogenizing, separating, and transferring samples. Generally, the tubes are cylindrical, tapering to a conical shape at the bottom similar to the configuration in a cyclone-based personal aerosol sampler (). As in a cyclone, air is tangentially introduced at the top of a microcentrifuge tube and extracted from the tube axis, creating a double vortex flow within the tube. In this flow field, particles having sufficient inertia impact onto the tube walls. The particles either retain on the wall or gradually migrate to the bottom of the tube due to the secondary flow in the boundary layer (CitationRanz 1985). This concept forms a basis of using microcentrifuge tubes in the design of a new bioaerosol sampler. Unlike conventional respirable aerosol samplers, the objective of this sampler design is to deposit particles of interest in the cyclone (tube) itself, which would be directly used as the collection container for subsequent analysis.
FIG. 1 Schematic diagram of the microcentrifuge-tube sampler: the microcentrifuge tube and the cyclone attachment.
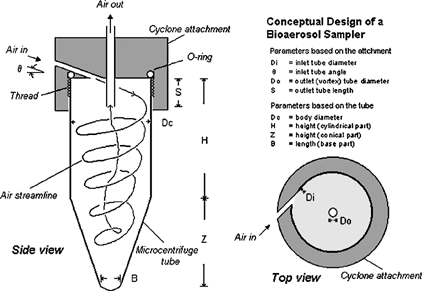
A number of microcentrifuge tubes with capacities between 1.5 and 2.0 ml were surveyed, and tubes from four different vendors were considered (). Besides the general selection criteria described above, additional requirements of a screw-top cap and polypropylene tube were adopted to ensure sample integrity during collection, preparation, and autoclaving.
TABLE 1 Description and physical dimensions of the 9 microcentrifuge tubes surveyed in this study
Generally, all tubes had a similar body diameter, Dc, around 0.82–0.83 cm (). Depending on the capacity of the tube and whether it was self-stand or not, the heights of cylindrical portion (H) and conical portion (Z) varied, but the total height (H + Z) remained approximately 44 ± 1 mm. The threads of the tubes were similar in pitch and threads per unit length but differed in thread length.
Conceptual Design of the Cyclone Bioaerosol Sampler
The new bioaerosol sampler consists of a brass cyclone attachment and a microcentrifuge tube (). The attachment was designed primarily to fit the tube and, at a commonly used flow rate of 2 l/min, to achieve a desired 50% cutoff size (D50) around 1–2 μ m. It consists of an inlet and an outlet, and when threaded to the tube it provides an enclosure similar to that in a conventional air cyclone. Although the dimensions of tubes are fixed and their relative values (H/Dc, Z/Dc, and S/Dc) do not follow those recommended in conventional cyclone designs, the inlet and outlet in the brass attachment were fabricated based on the design of the SRI-III cyclone (CitationSmith et al. 1979) and the sharp-cut cyclone (CitationKenny and Gussman 2000), in which Di = 0.24 Dc, Do = 0.27 Dc, and S = 0.35 Dc. Because all tubes used in the study have a similar Dc, their attachments have the same dimensions for Di (= 1.99 mm), Do (= 2.24 mm), and S (= 2.91 mm) except for the thread, which was fabricated to match the corresponding tube type.
Rather than tangentially placed at the top as in conventional cyclones, the cyclone inlet is introduced with an inclined angle, θ, of approximately 40° with respect to the horizontal direction. This design is intended to lead to initial particle impaction onto the tube wall, rather than onto the attachment surface, and to maximize collection of desired particles in the tube for analysis.
Based on the equation for the sharp-cut cyclone,
Preliminary Results
Six types of tubes were selected for a preliminary investigation of the cyclone bioaerosol sampler (i.e., tube types a, c, e, g, h, and i). Tubes were excluded if they were similar in dimension to another tested tube type (i.e., tube types b, d, and f). Leakage checks were performed on the six microcentrifuge tubes threaded to the corresponding sampling attachments. The integrity of each sampler was quantified by a series of pressure measurements on a sealed system that was initially brought to a pressure slightly below ambient pressure (CitationMokler and White 1983). With the use of an o-ring inserted in the attachment of each sampler (see ), there was no observable leakage present in any of the samplers.
Experiments were conducted in an aerosol test chamber by using fluorescently tagged polystyrene latex (PSL) particles of 1.94 μ m nominal diameter (Dp). The detailed procedures of aerosol generation, sampling, and analysis have been described by CitationFeather and Chen (2003). For each prototype sampler, a 13 mm backup filter was attached to the outlet of the attachment, allowing particles penetrating the sampler to be collected. Assuming that the microcentrifuge-tube sampler exhibits the same collection characteristics as the sharp-cut cyclone, the 1.94 μ m particles were expected to have a collection efficiency of over 50% at 2 l/min. It was, however, not the case based on the preliminary results. shows the fractional deposition of 1.94 μ m particles on the surfaces of the tube, filter, and attachment. The mean collection efficiency in the tubes varied with the type of tubes used and ranged between 13–21% at 2 l/min, which clearly does not follow the > 50% collection efficiency as predicted for the sharp-cut cyclone. The results show clearly that the sampler performance is not directly comparable to the short-cut cyclone, meaning that the equation for the cyclone cannot be used for predicting D50 of the sampler. These results were not surprising, however, given that the presence of inclined angle θ would create a steeper spiral and decrease collection efficiency through fewer vortex turns compared to a conventional cyclone, not to mention that the dimensional ratios were outside the recommended ranges.
TABLE 2 Fractional deposition (mean and 1 standard error, SE, n = 4) of 1.94 μ m particles on the different parts of the 6 samplers used in the preliminary study
also shows that, at Q = 4 l/min, the sampler performed better and resulted in higher tube collection efficiencies of 65–78% for 1.94 μ m particles, indicating that the sampler can exhibit higher collection efficiency and fulfill the requirement of over 50% collection for particles between 1–2 μ m by operating at a higher flow rate. Among the six tubes, tubes e, g, and h gave the highest collection efficiencies (> 20% at Q = 2 l/min and > 72% at Q = 4 l/min). Thus, they were selected for full-scale evaluation at 2 l/min and 4 l/min.
FULL-SCALE EVALUATION
Laboratory Testing Using Monodisperse Aerosols in a Calm Air Chamber
A calm-air chamber system described by CitationFeather and Chen (2003) was used in this study. The chamber is capable of providing a uniform distribution of monodisperse aerosols for sampler testing in a low-air-velocity environment (< 1.5 cm/s, the detection limit of velocity sensor), a condition that mimics normal workplaces (CitationBaldwin and Maynard 1998). In addition, it uses a reliable method of using horizontal- and vertical (upward)-facing sharp-edged probes for determining reference concentrations of aerosol particles up to 45 μ m in aerodynamic diameter.
Monodisperse fluorescently tagged polymer microspheres (Duke Scientific, Palo Alto, CA, USA) with median aerodynamic diameters of 0.5, 1, 2, 3, 6, 11, and 16 μ m were aerosolized using a liquid nebulizer for particles ≤3 μ m (Hospitak Inc., Cat. No. 952, Farmingdale, NY, USA) or a dry-powder venturi disperser for particles ≥6 μ m (In-Tox Products, Albuquerque, NM, USA). A diffusion dryer was used in series with the nebulizer to remove water from the aerosol. When the venturi disperser was used, a solenoid valve was installed to create pulsating flow in the air stream to help disperse the powder. Depending on the test particle size and the generation system, the particle concentration in the chamber varied, and thus sampling time could be adjusted from 15 min to 2.5 h to collect sufficient particles for analysis.
The mean flow rate from the generation nozzle was regulated at 55 l/min. Excess air entering the calm air chamber was passed through an automobile air filter at the top of the chamber. For each run, six test samplers (operating at either 2 or 4 l/min) and two reference samplers, one horizontal and one vertical facing (with the same flow rate as used in test samplers), were symmetrically placed inside the chamber. To avoid any effects due to electrostatic charges, brass sampler attachments and stainless-steel filter holders (for backup filters and reference samplers) were used. An aerodynamic particle sizer (APS; TSI Inc., Model 3320, St. Paul, MN, USA) was used to monitor the particle size and monodispersity of test aerosols.
A honeycomb flow straightener and a bipolar ion source, commonly used for neutralizing the electric charges on aerosol particles, were not installed in the system. This is because when air stream passed through the surface of the flow straightener or through the ion source, test particles deposited from the previous runs could be re-entrained. Previous studies indicated the problem to be especially severe for test particles larger than 6 μ m (CitationFeather and Chen 2003).
To minimize sampling bias caused by charged particles, an electrostatic field meter (Chapman Corp., Model EOS 100, Portland, ME, USA) was used prior to each run to ensure that no accumulated charge was present in the sampling area. Measurements taken in the vicinity of each sampler showed that there was no detectable electrostatic field associated with an accumulated charge (accuracy ± 5 volts).
Aerosol Homogeneity Test
To reduce the potential concentration bias caused by the dispersion nozzle, the nozzle was manually rotated once (180°) during each experimental run to enhance the homogeneity of the particle distribution inside the chamber. This was applied only to the particles larger than 6 μ m (CitationFeather and Chen 2003). Homogeneity tests were conducted prior to experimental runs with bioaerosol samplers. Six 13 mm filter holders, each containing a glass-fiber filter, were vertically placed in a symmetric manner in the sampling plane inside the chamber. Flow rates similar to those used in sampler evaluations were used. At the end of each test run, particle deposits on each filter were determined by measuring the fluorescence of filter extracts (see below). The coefficient of variation of the six sampling locations within the test chamber ranged from 0.62 to 3.08%.
Data Analysis
For each run, six samplers containing the tubes e, g, and h (two of each) were symmetrically placed inside the chamber. One horizontal and one vertical sharp-edged sampler were used for measuring reference concentrations and subsequently calculating the aspiration efficiencies of the samplers. At the end of each run, particle deposits on the surfaces of the sampling attachment (containing both the inlet and outlet), microcentrifuge tube, and outlet filter were separately analyzed to determine the collection efficiency and internal losses for each sampler. Ethyl acetate was used to extract fluorescent dye in each sample, and the fluorescence intensity was analyzed using a spectrofluorometer (Photo Technology International, Model C-60, Monmouth Junction, NJ, USA). The emission maximum was measured at 487 nm when the excitation maximum was set at 468 nm, as recommended by the manufacturer. Efficiencies and losses at the different particle sizes (0.5, 1, 2, 3, 6, 11, and 16 μ m), flow rates (2 and 4 l/min), and tubes (e, g, and h) were calculated and used for selecting the most suitable microcentrifuge tube for sampling fungal spore aerosols. For particles of a given size, the aspiration efficiency (Ea), collection efficiency (Ec), and internal loss (IL) were computed from
Deposition Profile
Prior to using ethyl acetate to extract the fluorescent dye from the microcentrifuge tube surface, the deposition patterns of fluorescence-tagged particles were observed macroscopically. Detailed deposition profiles were recorded by shining a black-ray lamp (UVP, Inc., Model B-100A, Upland, CA, USA) at the tubes and taking digital photographs (Canon USA Inc., PowerShot S330, Lake Success, NY, USA) of the fluorescent patterns inside the tubes. Cyclones operating at a flow rate of 3 l/min were also included in this study to examine any potential change in the deposition pattern due to flow transition between 2 and 4 l/min.
Effect of Coating on Tube Performance
The effect of thin-layer coatings on the collection efficiency in the tubes was studied. Any difference in collection efficiency with and without coating material would reveal the significance of particle bounce and re-entrainment inside the tubes. The internal surface of each tube was coated with polyethylene glycol (Dow Chemical, E900NF, Midland, MI, USA) to determine if a higher collection efficiency could be achieved, as in the case of conventional impactors. The material was selected because it did not result in any detectable background fluorescence when tested with ethyl acetate. In this study fluorescence PSL particles of 1.94 μ m were used at flow rates of 2 and 4 l/min and tube types e, g, and h.
Effect of Liquid Presence in the Tube
Although the cyclone bioaerosol sampler is intended for use to collect fungal spores in a dry tube, it was speculated that the presence of liquid in the microcentrifuge tube may increase sampler performance. Experiments were designed to determine liquid evaporation rate and particle retention efficiency.
In determining liquid evaporation rate, two setups with different air environments were used: (1) place the sampler in the laboratory (outside the chamber) and draw the air directly from the room and (2) place the sampler in the chamber and supply the dry clean air as in a normal run but with no aerosol particles being generated. Both tests were necessary—the latter because it would help interpret data on particle retention efficiency in the presence of liquid under a dry-air control environment, and the former because it would provide a realistic evaluation of evaporation rate in an indoor environment with modest moisture content. Although the mechanism of evaporation is complex and depends on the thermodynamic properties and the contact surface area between the liquid and air, only distilled water was used as the liquid to provide basic information on the rate of evaporation at a given sampling time. In the first study, samplers containing 200 μ l of distilled water in the microcentrifuge tubes were placed in the laboratory room and operated at a flow rate of 4 l/min. Liquid volume was measured every 30 min up to 5 h. Relative humidity was monitored with a VelociCalc® (TSI Inc., Model 8386, St. Paul, MN, USA).
To investigate the particle collection (retention) efficiency in the presence of liquid (using the second setup), the experiments were conducted in the chamber using the monodisperse fluorescence PSL particles of 1.0, 1.94, and 3.0 μ m, respectively. For the side-by-side comparison, six samplers, three with 200 μ l distilled water in the tube and three without, were symmetrically placed in the chamber. The sampling time were varied from 15 min to 2 h. The maximum time of 2 h was selected as preliminary results indicated that, under the dry air condition in the chamber, distilled water would evaporate in 2 h at a flow rate of 4 l/min. At the end of a given run, the samplers were taken out of the chamber, and fluorescence intensities from different sections of the samplers were measured as described above. For the sampler with liquid, the procedures were slightly different in that the liquid in the tube was measured separately for its volume and then analyzed for the fluorescence intensity, Fl. The fluorescence intensity from particles deposited in the tube but not in the liquid was analyzed as Ft− l . The total fractional intensity in the tube was therefore given by Ft = F l + Ft− l and the percent contribution of liquid in the total tube fluorescence intensity as F l /Ft.
RESULTS AND DISCUSSION
Deposition Profile in the Microcentrifuge Tube
shows the top and side views of the 1.94 μ m fluorescent PSL deposits in microcentrifuge tubes. Samplers were operated at 2, 3, and 4 l/min. The top views are similar to the spiral pattern of fluidized dust shown by CitationRanz (1985) on cyclone walls. The deposits demonstrate that a vortex flow pattern was created inside the microcentrifuge tubes in the presence of air flow and that particles having sufficient inertia cross the air streamlines and can impact onto the tube walls. In addition, the deposition band is narrower and more distinctive, and the tube contains a greater quantity of deposit (mainly at the bottom) at higher flow rates. This was expected, as higher flow rates will have resulted in a smaller 50% cutoff diameter, thus generally yielding a heavier deposit on the tube surface (). From , the fractional deposit would be 72% at 4 l/min compared to only 20% at 2 l/min. Finally, the heavy deposit at the bottom may have been due to wall flow in the boundary layer playing an important role in transporting the particles along the walls to the bottom of the cyclone cone (CitationRanz 1985).
FIG. 2 Photographs showing the top and side views of particle deposition patterns in microcentrifuge tubes (Tube e) at 2, 3, and 4 l/min.
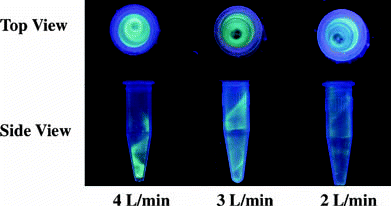
FIG. 3 Particle fractional deposition in the tubes (collection efficiency), on the attachments (internal loss), and on the filters (penetration efficiency) at the flow rate of (a) 2 and (b) 4 l/min. Three different tubes (e, g, and h) were tested, and mean values from each tube type were shown (n = 4); error bars were not shown because they are smaller than the symbols. The curves are smooth lines that connect the data points.
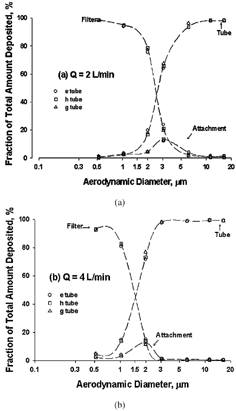
TABLE 3 Operating parameters and fractional deposition values in the microcentrifuge-tube sampler
Sampler Performance
Sampler Aspiration Efficiency
shows the aspiration efficiency of aerosol particles into the sampler. Each point represents the mean ± 1 standard deviation of 12 measurements, 4 per tube type. No differences in aspiration efficiency among the three tube types e, g, and h were observed. The fluorescence intensity measurements obtained from the vertical and horizontal references were very close (CV < 3%) for all the test particles, and thus mean values from the two references were adopted for calculating the aspiration efficiency (CitationFeather and Chen 2003). The results indicate that the sampler has an aspiration efficiency of 97–102% for the particles tested in the calm-air chamber, which is very consistent with the data of other samplers (CitationFeather and Chen 2003) and follows well with the proposed calm-air inhalable convention (CitationKenny et al. 1999). To test the sampler for the complete size range of the inhalable curve, future evaluation will include particles larger than 20 μ m.
Sampler Tube Collection Efficiency and Internal Wall Loss
As the average flow rate increases so does the inlet air velocity, the flow Reynolds number, the Stokes number, and the mean fractional deposit in the tube (). These data were collected from the samplers using the e tubes.
The fractional deposition associated with the three tested microcentrifuge tubes e, g, and h was similar at 2 and 4 l/min (). The “filter” curves represent the percentage of particles that penetrate through the cyclone and collected on the backup filter. The “attachment” curves show the fractional deposits in the attachment section, which essentially represents internal wall losses in the sampler. It is interesting to note that the peaks of the “attachment” curves match well with the 50% cutoff size (see below), a phenomenon that has been seen to occur in other inertial devices such as virtual impactors (CitationMcFarland et al. 1978; CitationChen et al. 1985). The “tube” curves represent those particles collected and retained in the microcentrifuge tube, which is the focus in this study. According to the “tube” curve in , at a flow rate of 2 l/min the collection efficiency in the tube increases with particle size, ranging from 1–2% for 0.5 μ m to 98–99% for 16 μ m. The higher flow rate of 4 l/min resulted in a better sampling efficiency for a given particle size ( and ). In addition, the similar results among the three tubes e, g, and h seem to imply that the other 1.5 ml microcentrifuge tube, d, and even the 1.7 ml microcentrifuge tubes, b and c, would probably have the similar performance since they have the similar physical dimensions as the tested tubes.
D 50 (the 50% Cutoff Diameter)
Collection efficiency measurements at 2 and 4 l/min are well described by an “S” shape curve (). At the flow rate of 2 l/min, the 50% cutoff aerodynamic diameter was 2.5 μ m. At the higher flow rate, the curve shifts to the left, with a smaller 50% cutoff size of 1.5 μ m. Again, there is no difference in efficiency curves among the three different tubes tested.
FIG. 5 The tube collection efficiency curves at two different flow rates. Each data represents the mean of the data from three types of tubes shown in , and the error bar represents ± 1 SD. The curves are smooth lines that connect the data points.
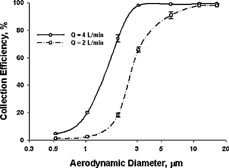
Although the microcentrifuge-tube sampler is unique in its own class and is different from the well-characterized cyclones, it is of interest to compare its performance with other cyclones. The normalized cutoff diameter, D50/Dc, rather than the conventional cutoff diameter, D50, is used for comparison by plotting versus flow rate, as described by CitationZhu et al. (2001). Compared to the straight-line plot in the low-flow-rate region (CitationZhu et al. 2001), the normalized cutoff size of this microcentrifuge-tube sampler fits very well with the straight-line plot at 2 l/min and is slightly higher than the corresponding value on the line at 4 l/min, indicating that this microcentrifuge-tube sampler performs well as compared to conventional cyclone samplers.
Sharpness in Tube Collection Efficiency
In addition to the normalized cutoff size, the sharpness of the efficiency curve is another useful parameter for characterizing the sampling effectiveness when comparing with other cyclones. For the microcentrifuge-tube sampler, the sharpness of the curves in is determined as (D84/D16)1/2. D84 and D16 represent the diameters of particles that would result in 84% and 16% of collection efficiency in the tube by interpolating the data points from the particles tested. The sharpness of the tube collection efficiency curve in this microcentrifuge-tube sampler is estimated to be 1.42 at 4 l/min and 1.67 at 2 l/min. Although the latter value is larger than those of conventional cyclones, the former is in the range of 1.3–1.5, which is considered to provide a sharp cutoff and a well-defined size separation (CitationWatson and Chow 1993).
Consolidation of Efficiency Curves
Attempts have been made, but without success, to use the Stokes number for consolidating the two efficiency curves. This seems to indicate that the characteristic of dynamic similarity for the Stokes number, which occurs in other devices (CitationChen et al. 1985), may not apply to this sampler. However, a dimensionless normalized particle diameter, (Dp−D50)/D50, is able to result in a single curve for all the data collected at two different flow rates, and it would help estimate the collection efficiency for particle sizes not tested in the study ().
Empirical Model
As described above, the dimensions of Di, Do, and S in the sampler's attachment are based on the relative dimension relationships and EquationEquation (1) (CitationKenny and Gussman 2000). The equation was primarily used for range finding of a preliminary D50 as well as examining the feasibility of using microcentrifuge tubes as the collection medium. It was expected that the D50 values resulting from the study would not be the same as those predicted by the equation, partly because the equation was established for particles penetrating through the cyclone rather than those retained in the cyclone. Thus, unless there is no internal loss on the top surface of the cyclone, D50 values determined based on the collection (or “tube” curve) would always be larger than those based on the separation (or “filter” curve; see ).
More importantly, the sampler would not perform according to the equation by CitationKenny and Gussman (2000) because of the constraint of a tilted inlet angle and the fixed dimensions in the microcentrifuge tube, which are very different from the geometry and relative dimensions used in the equation. As discussed in the preliminary results, the inclined angle θ of the inlet would provide a much sharper spiral pattern and result in much fewer vortex turns as compared to those in a sharp-cut cyclone, not to mention that the values of dimensional ratios were outside the recommended ranges.
Because only two D50 values at 2 and 4 l/min are available from this study, it is not adequate to fit these data with the equation of D50 = ea Dc bQ1 −b and estimate the empirical constants a and b using nonlinear least-squares regression (CitationKenny et al. 2000). However, with more D50 data obtained from different flow rates Q or using tubes of different Dc, it would be possible to establish equations with constants that enable us to predict collection efficiency values for microcentrifuge samplers operated at different flow rates or to design similar cyclone-based samplers that would have desired D50 values at specific flow rates.
Effect of Coating
shows the comparison of tube deposition data with and without a coating of polyethylene glycol. The mean values for the coated tubes were slightly higher than those on the uncoated tube at the flow rate of 2 l/min and slightly lower for the coated tubes at the flow rate of 4 l/min (). There was no significant difference in the collection efficiency between coated and uncoated tubes (assessed by t test) for either flow rate.
TABLE 4 Comparison of fractional depositions of 1.94 μ m particles between the uncoated and polyethylene glycol coated tubes
The results of no difference with and without a coating seem to echo the finding reported in the deposition profile in the tube, in which the particles did not impact and overload the wall surface, but instead mainly settled at the bottom of the tube. This implies that particle bounce and re-entrainment of the polymer microspheres did not cause problems, and coating, as commonly used in a conventional impactor, would not be needed.
Effect of Liquid in the Microcentrifuge Tube
The evaporation rate of distilled water in the tube at a flow rate of 4 l/min was lower at higher relative humidities (i.e., room air at ∼ 45% relative humidity versus chamber air at ∼ 6% relative humidity, ). Each point represents the average of two independent runs. The results clearly demonstrate that water in the tube is retained much longer by using ambient air in the laboratory than using the drier dilution air in the chamber.
FIG. 7 Evaporation rate curves of distilled water in the tube at a flow rate of 4 l/min: (a) ambient air with a 45–50% relative humidity in a room and (b) dry dilution air with a 6–7% relative humidity in the chamber (n = 2).
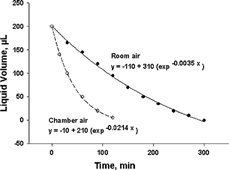
indicates that, when operating the sampler using the ambient laboratory air, the 200 μ l water will evaporate as a function of time and will eventually dry out after 5 h of sampling at 4 l/min. The data on the curve can also be used even when the original water content is different from 200 μ l. For example, assuming that the original water content is 145 μ l, there would still be 95 μ l remaining in the tube after 1 h of sampling. However, when operating the sampler in the chamber, the amount of 200 μ l would dry out in approximately 2 h with a faster rate of evaporation. This information is important when setting up the sampling time for comparing the particle deposits with and without water in the tube.
shows that, depending on the use of room air or chamber air, there would be 145 or 50 μ l of water remaining in the tube after 1 h. shows the mean fractional fluorescence intensity data from sampling fluorescently tagged PSL particles in the presence of water compared to that without water in the tube. The aspiration efficiencies in both cases are close to 100%. However, the presence of water in the tube seems to result in a slightly higher particle deposition efficiency in the tube for all three sizes tested. Although the mechanisms leading to increased deposition are unclear, it is likely that the air streamlines and/or particle trajectories changed sufficiently in the water-containing tubes to lead to greater deposition probability for a given particle size. In addition, there would be a slight shift in the “tube” curve to the left with a smaller D50. shows that the fluorescence intensity of liquid portion amounts to 6–10% of the total tube intensity among the three particle sizes and three tubes tested. This value may be underestimated because some particles could have been in the liquid yet adhered to the bottom surface of the tube, and their intensities not measured as part of the liquid. Although this value was obtained using chamber air, it provides a reasonable estimate for the percent particle deposit in the liquid under ambient air as long as the final liquid level is the same. This could have an application in real-time diagnosis when certain particulate agents deposited in the tube react with liquid assay present in the tube and result in color change for fast detection.
TABLE 5 Comparison of particle fractional deposition on different parts of the sampler with and without water in the microcentrifuge tube
CONCLUSIONS
A prototype personal sampler using microcentrifuge tubes as the collection receptacle has been designed and fabricated, and its performance evaluated for collecting aerosol particles in the size range of fungal spores. The microcentrifuge tube sampler, operating at a flow rate of 4 l/min with a cutoff diameter of 1.5 μ m, will provide good collection efficiency for fungal spore-sized particles. The major advantage of this sampler is that no additional sample transfer or handling is necessary since the collection container is an integral part of the sampler. Experimental results have shown that the concept of using microcentrifuge tubes for particle collection works, and that the sampler behaves like a conventional air cyclone. In addition, the results indicate that, unlike conventional impactors, the sampler's collection efficiency will not be influenced by whether or not there is coating on the tube surface. The performance of the sampler was not affected by the different brands of tubes tested as long as the physical dimensions were similar. The sampler has a 100% aspiration efficiency for particles up to 16 μ m at 2 l/min and 4 l/min. At 4 l/min, nearly 100% of particles greater than 3 μ m and > 90% of particles between 2.5 and 3 μ m were collected in the microcentrifuge tube. These sampling efficiency parameters are comparable to commercially available fungal spore samplers.
Acknowledgments
The authors are indebted to several researchers at NIOSH: Bill Wallace, Dan Lewis, and Sid Soderholm for their encouragement of this research, and Jon Volkwein, Martin Harper, and Paul Baron for their review of this manuscript. The apparatus and analysis methods of the personal sampler for collecting fungal spores are patent pending. Any commercial use of the personal sampler and analysis methods requires a license from the Technology Transfer Office of the Centers for Disease Control and Prevention.
Disclaimer: Mention of a specific product or company does not constitute endorsement by the Centers for Disease Control and Prevention.
Notes
*F l /Ft in %, where F l is the fluorescence intensity in the liquid and Ft is the total fluorescence intensity in the tube (including the liquid).
REFERENCES
- Macher , J. M. , Chatigny , M. A. and Burge , H. A. 1995 . “ Sampling Airborne Microorganisms and Aeroenergyens ” . In Air Sampling Instruments for Evaluation of Atmospheric Contaminants , Edited by: Cohen , B. S. and Hering , S. V. 589 – 617 . Cincinnati , OH : American Conference of Governmental Industrial Hygienists . In
- Willeke , K. and Macher , J. M. 1999 . “ Air Sampling ” . In Bioaerosols: Assessments and Control , Edited by: Macher , J. M. pp. 11.1 – 11.25 . Cincinnati , OH : American Conference of Governmental Industrial Hygienists . In
- American Industrial Hygiene Association . 1996 . Field Guide for the Determination of Biological Contaminants in Environmental Samples , Edited by: Dillon , H. K. , Heinsohn , P. A. and Miller , J. D. pp. 45 – 46 . Fairfax , VA : American Industrial Hygiene Association .
- Baldwin , P. E. J. and Maynard , A. D. 1998 . A survey of wind speeds in indoor workplaces . Ann. Occup. Hyg. , 42 : 303 – 313 .
- Chen , B. T. , Keswani , J. , Zhou , G. and Ong , T. 2002 . “ Using PCR to detect indoor fungi ” . In Proceedings of the 9th International Conference on Indoor Air Quality and Climate Edited by: Levin , H. pp. 63 – 68 . Santa Cruz , CA Indoor Air 2002
- Chen , B. T. , Yeh , H. C. and Cheng , Y. S. 1985 . A Novel Virtual Impactor: Calibration and Use . J. Aerosol Sci. , 16 : 343 – 354 .
- Cruz-Perez , P. , Buttner , M. P. and Stetzenbach , L. D. 2001 . Detection and Quantitation of Aspergillus fumigatus in Pure Culture Using Polymerase Chain Reaction . Mol. Cell. Probes , 15 : 81 – 88 .
- Delfino , R. J. , Zeiger , R. S. , Seltzer , J. M. , Street , D. H. , Matteucci , R. M. , Anderson , P. R. and Koutrakis , P. 1997 . The Effect of Outdoor Fungal Spore Concentrations on Daily Asthma Severity . Environ. Health Perspect. , 105 ( 6 ) : 622 – 635 .
- Feather , G. A. and Chen , B. T. 2003 . Design and Use of a Settling Chamber for Sampler Evaluation under Calm-air Conditions . Aerosol Sci. Technol. , 37 : 261 – 270 .
- Haugland , R. A. , Vesper , S. J. and Wymer , L. J. 1999 . Quantitative Measurement of Stachybotrys chartarum Conidia Using Real Time Detection of PCR Products with the TaqMan™ Fluorogenic Probe System . Mol. Cell. Probes , 13 : 329 – 340 .
- Kenny , L. C. , Aitken , R. J. , Baldwin , P. E. , Beaumont , G. C. and Maynard , A. D. 1999 . The Sampling Efficiency of Personal Inhalable Aerosol Samplers in Low Air Movement Environments . J. Aerosol Sci. , 30 : 627 – 638 .
- Kenny , L. C. and Gussman , R. A. 2000 . A Direct Approach to the Design of Cyclones for Aerosol-Monitoring Applications . J. Aerosol Sci. , 31 : 1407 – 1420 .
- Kenny , L. C. , Gussman , R. A. and Meyer , M. 2000 . Development of a Sharp-Cut Cyclone for Ambient Aerosol Monitoring Applications . Aerosol Sci. Technol. , 32 : 338 – 358 .
- Levetin , E. 1995 . “ Fungi ” . In Bioaerosols , Edited by: Burge , H. A. pp. 87 – 120 . Boca Raton , FL : CRC Press, Inc. . In:
- Macher , J. M. 2001 . Evaluation of a Procedure to Isolate Culturable Microorganisms from Carpet Dust . Indoor Air , 11 : 134 – 140 .
- McFarland , A. R. , Ortiz , C. A. and Bertch , R. W. Jr. 1978 . Particle Collection Characteristics of a Single-Stage Dichotomous Sampler . Environ. Sci. Technol. , 12 : 679 – 682 .
- Mokler , B. V. and White , R. K. 1983 . Quantitative Standard for Exposure Chamber Integrity . Am. Ind. Hyg. Assoc. J. , 44 : 292 – 295 .
- Neas , L. M. , Dockery , D. W. , Burge , H. , Koutrakis , P. and Speizer , F. E. 1996 . Fungus Spores, Air Pollutants, and Other Determinants of Peak Expiratory Flow Rate in Children . Am. J. Epidemiol. , 43 : 797 – 807 .
- Ranz , W. E. 1985 . Wall Flows in a Cyclone Separator: A Description of Internal Phenomena . Aerosol Sci. Technol. , 4 : 417 – 432 .
- Rao , C. Y. , Burge , H. A. and Chang , J. C. S. 1996 . Review of Quantitative Standards and Guidelines for Fungi in Indoor Air . J. Air Waste Manag. Assoc. , 46 ( 9 ) : 899 – 908 .
- Reponen , T. , Willeke , K. , Ulevicius , V. , Reponen , A. and Grinshpun , S. A. 1996 . Effect of Relative Humidity on the Aerodynamic Diameter and Respiratory Deposition of Fungal Spores . Atmos. Environ. , 30 ( 23 ) : 3967 – 3974 .
- Schmechel , D. , Gorny , R. L. , Simpson , J. P. , Reponen , T. , Grinshpun , S. A. and Lewis , D. M. 2003 . Limitations of Monoclonal Antibodies for Monitoring of Fungal Aerosols Using Penicillium brevicompactum as a Model Fungus . J. Immunol. Methods , 283 : 235 – 245 .
- Smith , W. B. , Wilson , R. R. and Bruce , D. B. 1979 . A Five-stage Cyclone System for in situ Sampling . Environ. Sci. Technol. , 13 : 1387 – 1392 .
- Verhoeff , A. P. and Burge , H. A. 1997 . Health Risk Assessment of Fungi in Home Environments . Ann. Allergy Asthma Immunol. , 78 : 544 – 556 .
- Watson , J. G. and Chow , J. C. 1993 . “ Ambient Air Sampling ” . In Aerosol Measurement: Principles, Techniques, and Applications , Edited by: Willeke , K. and Baron , P. A. 622 – 639 . New York : Van Nostrand Reinhold . In
- Zhou , G. , Whong , W. , Ong , T. and Chen , B. T. 2000 . Development of a Fungus-Specific PCR Assay for Detecting Low-level Fungi in an Indoor Environment . Mol. Cell. Probes , 14 : 339 – 348 .
- Zhu , Y. , Kim , M. C. , Lee , K. W. , Park , Y. O. and Kuhlman , M. R. 2001 . Design and Performance Evaluation of a Novel Double Cyclone . Aerosol Sci. Technol. , 34 : 373 – 380 .