Abstract
To evaluate our initial experience with image guided respiratory gated H-SBRT for liver and lung tumors. The system combines a stereoscopic x-ray imaging system (ExacTrac® X-Ray 6D) with a dedicated conformal stereotactic radiosurgery and radiotherapy linear accelerator (Novalis) and ExacTrac® Adaptive Gating for dynamic adaptive treatment. Moving targets are located and tracked by x-ray imaging of implanted fiducial markers defined in the treatment planning computed tomography (CT). The marker position is compared with the position in verification stereoscopic x-ray images, using fully automated marker detection software. The required shift for a correct, gated set-up is calculated and automatically applied. We present our acceptance testing and initial experience in patients with liver and lung tumors. For treatment planning CT and Fluorodeoxyglucose-Positron Emission Tomography (FDG-PET) as well as magnetic resonance imaging (MRI) taken at free breathing and expiration breath hold with internal and external fiducials present were used. Patients were treated with 8–11 consecutive fractions to a dose of 74.8–79.2 Gy. Phantom tests demonstrated targeting accuracy with a moving target to within ±1 mm. Inter- and intrafractional patient set-up displacements, as corrected by the gated set-up and not detectable by a conventional set-up, were up to 30 mm. Verification imaging to determine target location during treatment showed an average marker position deviation from the expected position of up to 4 mm on real patients. This initial evaluation shows the accuracy of the system and feasibility of image guided real-time respiratory gated H-SBRT for liver and lung tumors.
Despite recent technology advances in radiation oncology the risk of adverse radiation normal tissue effects may preclude delivery of sufficient dose with SBRT (Stereotactic Body Radiation Therapy) to control respiratory dependent moving liver and lung tumors. Currently several approaches to monitor respiratory tumor motion are on the verge to clinical implementation Citation[1].
Real-time indirect surveillance of a moving tumor seeks to relate its trajectory to an external coordinate system via treatment planning computed tomography (CT) and optical tracking of external fiducial markers attached to a patient in treatment position Citation[2–5]. This method assumes a rigid relationship between external fiducial markers and the internal target. However, both inter- and intrafraction target localization may be affected by re-fixation of markers, respiratory irregularities, change in residual lung volume, as well as skin shift and internal soft tissue deformation Citation[4–8]. To accomplish real-time direct surveillance of a moving tumor optical tracking of external fiducials may be combined with real-time stereoscopic x-ray images of internal landmarks or implanted fiducials acquired at the linac Citation[2], Citation[9], Citation[10]. The recorded data transfer to the linear accelerator allows that the radiation beam is only turned on when the localization of a respiratory dependent moving tumor corresponds to a preset gating window at the exhale respiration level.
We report the clinical implementation of ExacTrac® Adaptive Gating (BrainLAB, Heimstetten, Germany) at the Charité and our initial experience with the system for H-SBRT with regard to the accuracy of patient set-up and treatment of respiratory dependent moving liver and lung tumors.
Material and methods
Phantom measurements
For accuracy and verification measurements a computer-controlled phantom simulating a breathing motion was developed. It includes a vertically moving platform for passive infrared markers and a longitudinally moving dose cube. In the dose cube different type markers are imbedded for positioning and verification purposes. For real-time surveillance of a simulated respiratory dependent moving target a radio-opaque marker, similar to those implanted for treatments, was used. For a gated Winston-Lutz-Test a 5 mm tungsten sphere was implemented in the bottom plate of the moving dose cube with a radiographic film right below in the non-moving part of the phantom. To simulate the treatment process the phantom was CT-scanned. Following data transfer to the treatment planning system (BrainSCAN v 5.31, BrainLAB AG) IR (infra-red) and “implanted” markers were identified. The planning data were exported to the Novalis and used to gate the linac accordingly. For the gated Winston-Lutz test the phantom was positioned under motion and x-ray guidance, with the center of the tungsten sphere defined as treatment isocenter, to a high gradient point of the motion phase. The gated Winston-Lutz test was applied with a 10 mm collimator and a narrow beam-on window of 10% to verify the complete positioning and gating process. The film (X-Omat V, Kodak™) in the non-moving compartment was scanned and the position deviation of the center of the shadow created by the tungsten sphere to the center of the circular radiation field from the conical collimator was measured.
CT and ultrasound-guided gold marker implantation
For CT guided marker implantation into liver and lung tumors a single slice CT (Somatom 4, Siemens, Erlangen, Germany) with fluoroscopy was used. Marker implantation with an ultrasound device (HDI 5000, Philips Ultrasound) using a dynamic 2 to 5 MHz sector scanner was only performed in liver tumors. In all cases 0.75×30 mm gold markers were implanted into the tumor (VISICOIL™, Radiomed, Tyngsboro, MA, USA). In all cases, after local anesthesia with lidocaine 1% and under sterile conditions using an 18 or 17 Gauge coaxial needle (Super-Core, InterV, Gainesville, FL, USA) a VISICOIL™ marker was implanted into the tumor center. For the implantation the inner needle was removed and the marker with delivery system inserted. After removal of the delivery system the marker was pushed with the inner needle of the coaxial needle until it reached the needle end. The outer needle was withdrawn while keeping the inner needle in position, which resulted in a straight-lined gold marker within the tumor center.
Patient treatment and delivery
Patients were first immobilized in an individually molded whole vacuum cushion. For target localization and treatment planning at least four CT-scans per patient with a maximum axial slice thickness of 3 mm with and without contrast under free breathing and breath-hold conditions and IR markers attached to the patient's chest and abdomen in suitable positions to yield a good breathing signal were acquired. Following transfer to the TPS the reference CT was localized and correlated by image fusion with all other CTs, a Fluorodeoxyglucose-Positron Emission Tomography (FDG-PET), and for liver tumors an additional high resolution gadolinium enhanced MRI carried out with a 1.5 Tesla Magnetom (Siemens, Erlangen, Germany). For target definition the composite tumor volume shown on the CT, MRI and FDG-PET was segmented as GTV with no expansion for the clinical target volume (CTV). A 5 mm margin in all directions was added as physical target volume (PTV). The respiratory dependent tumor movement was defined as motion envelope on the FDG-PET. The motion envelope had in all cases an ellipsoidal shape. As an estimate for the respiratory dependent tumor movement the longest axis of the motion envelope is supplied. Treatment planning was accomplished with five to seven coplanar static beams. Treatment plans were optimized using DVHs (dose volume histograms) of any irradiated vital organ, e.g. lung, liver, spinal cord, stomach, cardiac ventricles, esophagus and kidneys. The combined left and right lung DVH was used to calculate the percentage of total lung volume (excluding GTV) receiving ≥10 Gy (V10) and ≥20 Gy (V20). Thereafter, the coordinates of the tumor isocenter, the external passive IR markers, and the implanted marker were transferred to the ExacTrac® Adaptive Gating platform. Treatment delivery is accomplished using ExacTrac® Adaptive Gating in combination with Novalis, a single 6 MV linear accelerator equipped with a M3 micro-multileaf collimator (BrainLAB, Heimstetten, Germany). The initial acceptance testing, commissioning and dosimetry as well as the rationale for treatment planning and delivery have been reported previously Citation[11–14]. In brief, two kilo voltage (kV) x-ray tubes, two amorphous silicon imaging detectors, two IR and one video camera facilitate optical and x-ray tracking to detect the external, real-time breathing motion as well as motion of the internal target. External passive IR markers attached to the patient's skin are used for the initial set-up and to determine the breathing pattern. The respiratory moving target is positioned, under IR and x-ray guidance to a defined point within the patients’ end expiratory breathing cycle. The gating reference level (red line) and additional x-ray imaging levels are defined to evaluate target motion due to respiration (blue line) (A). B shows the x-ray image of a lung tumor patient with the implanted marker for the gated set-up after automatic marker detection following x-ray acquisition at the gating reference and additional imaging levels. C displays an example for an on-target x-ray verification in treatment mode for the same patient at the gating reference level with superposition of the expected marker position plus the defined tolerance threshold of 3 mm. The localization is accomplished using the ends of the implanted gold marker, which have been pre-defined on the treatment planning CT together with the treatment isocenter and the body markers. The reconstruction of the location of the implanted marker is projected on the daily x-rays and makes it possible that the marker position is automatically detected by the software to allow the calculation of the positioning shift and subsequent verification. The calculated shift, corresponding to the difference of set-up and planned patient location, is applied to the treatment couch, and the patient is automatically repositioned so that the target is at the isocenter of the beam when the breathing curve intersects with the gating reference level. The treatment beam is then gated based on this breathing cycle. The percentage of the breathing cycle around the gating reference level that will be used for treatment can be individually selected, based on previous measurements. The size of the beam-on window should be determined based on the target motion as detected by the additional imaging levels and the amount of safety margin used for treatment planning. Once the machine is clinically ready and the beam-on is pressed, the radiation beam will automatically be turned on when the breathing curve is within the beam-on window, and off once it is outside. Verification x-rays can be taken at any point during the treatment.
Patient eligibility and characteristic
All patients were treated after written consent according to the SBRT protocols of the Department of Radiation Oncology CCM reviewed by the institutional review board. From October 2004 to June 2005 three patients with lung and three with liver tumors received image guided real-time respiratory gated H-SBRT using ExacTrac® Adaptive Gating. Eligibility criteria were (1) primary or metastatic liver and lung tumors at first presentation or recurrence, (2) clearly defined margins and contrast enhancement on CT, FDG-PET and/or MRI, (3) histologic tumor verification at initial diagnosis or recurrence, (4) full recovery from side effects of any prior therapy (i.e. no residual grade 3/4 toxicity) with an interval of at least 4 weeks since any prior chemotherapy and at least 12 weeks since completion of external beam radiotherapy (EBRT) for the primary tumor side, (5) ECOG performance status < 2, (6) adequate bone marrow function (WBC > 3.5×109/l Granulocytes > 1.5×109/l Platelets >100×109/l Haemoglobin >9 g/dl, after transfusion, if needed), liver (Serum bilirubin < 2.0 mg%, SGOT, SGPT and Alkaline Phosphatase < two-times the upper limit of the reference range) and renal function (Serum creatinine <1.5 mg% or creatinine clearance >60 ml/min), (7) life-expectancy of at least 3 months, and (8) written informed consent. Exclusion criteria were one or more of the following (1) small cell lung cancer and multifocal disease, (3) pregnancy or breast feeding, (4) history of drug, medication or alcohol abuse, (5) participation in another trial with an investigational drug within at least 1 month prior to trial entry, and (6) known of pronounced acute, sub acute and late adverse radiation reaction EBRT.
All presented six patients with seven lesions were treated between October 2004 and June 2005. The median follow-up is 18.5 months ±3.3 months with a range of 12 to 21 months. H-SBRT was delivered in 8 to 11 fractions on consecutive days to a dose between 74.8 and 79.2 Gy prescribed to the isocenter with six to eight equal weighted and spaced coplanar beams. All PTVs were covered by the 80% isodose. For data analysis stereoscopic x-ray verification images taken prior and during treatment were evaluated. The patient characteristics are detailed in .
Table I. Patient characteristics, including average treatment times and number of x-ray verification images evaluated for data analysis. KPS (Karnofsky Performance Status), COPD (chronic obstructive pulmonary disease), NSCLC (non small cell lung cancer)
Endpoints
Phantom measurements were used to assess the overall accuracy of H-SBRT in combination with ExacTrac® Adaptive Gating.
Under clinical conditions conventional set-up accuracy using IR markers was compared with set-up accuracy under gating conditions with ExacTrac x-ray. The on-target verification was assessed by comparing under free breathing conditions the implanted marker position to the supposed marker position at the gating reference level in treatment position. The analysis is based on the evaluation of 72 fractions and 937 x-ray verification images.
Patient outcome measures were treatment related liver and pulmonary morbidity defined as any new or worsening functional impairment not attributable to tumor progression and scored according to CTCAE v 3.0 Citation[15]. Tumor response was determined according to the Response Evaluation Criteria in Solid Tumors (RECIST) Citation[16]. Functional independent survival was assessed by interviewing patients about their ability to engage in activities of daily life, and Karnofsky performance status (KPS).
Follow-up evaluations
Patients returned to the clinic for evaluation 4 to 6 weeks after H-SBRT, and then every 3 months depending on their clinical condition. Every patient underwent a detailed examination and appropriate diagnostic imaging. CTs before and after H-SBRT were evaluated volumetrically by image fusion.
Statistical methods
Reference time points were date of H-SBRT and last follow-up. The marker end points were defined in every x-ray image and the deviation to the expected marker position from the treatment planning CT was calculated. From this the displacement vector for every verification image and an average displacement value for each fraction and the entire treatment were calculated.
Results
Phantom measurements
The phantom measurement for the gated Winston-Lutz Test showed an overall system accuracy of ±1 mm (). Film dosimetry measurements demonstrated in cross-plan profiles for a 2 cm square field a substantial dose blurring leading to an increase in penumbra and loss of target coverage under non-gated conditions. A good agreement in target coverage between the planned and delivered dose distribution was recorded under gating conditions. For a 10% beam-on window no difference in the 80–20% penumbra was found compared to a static field, while for a 20% beam-on window a 2 mm difference was measured ().
Initial clinical experience
The maximum and average deviation between IR guided and gated x-ray set-up in the vertical, longitudinal and lateral direction is shown for all patients in . For both patients (patient 1 and 2) with peripheral located lung tumors the average difference plus standard deviation (SD) between IR guided and gated x-ray set-up was in the vertical direction ≤6.5 mm, in the longitudinal direction ≤8.9 mm and in the lateral direction ≤4.9 mm. For the third patient with a centrally located metastasis in segment 7 of the right lung in close proximity to the heart the average difference plus SD between IR guided and x-ray set-up was in the longitudinal direction ≤33.9 mm, in the vertical direction <7 mm, and in the lateral direction <3.9 mm. A representative example for the considerable random non-predictable interfraction variability between IR and x-ray set-up seen in all patients is shown for patient 1 with a lung metastasis in A.
Figure 4. Histogram comparison of interfractional variation between conventional versus gated set-up for patient 1 with a lung and patient 3 with a liver tumor.
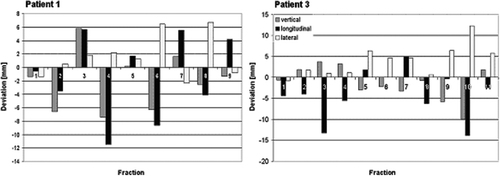
Table II. Maximum and average deviation between IR guided and gated x-ray set-up ±SD in mm as well as average deviation of expected to gated x-ray verification of implanted marker position ±SD in mm for individual patients and tumor localizations
The on target verification under free breathing conditions showed a substantial variation between the real and expected intra tumor marker position for the patients with lung tumors (). The largest difference between the real and expected marker position was recorded for the second patient with a constantly changing breathing cycle due to her oxygen dependent chronic obstructive pulmonary disease (COPD). A shows for patient 1 that there was also a considerable random interfraction variation between the real and expected intra tumor marker position under gated treatment conditions.
Figure 5. Intrafractional average marker displacement±SD and interfractional variation of on target verification for all fractions for patient 1 with a lung and patient 3 with a liver tumor.
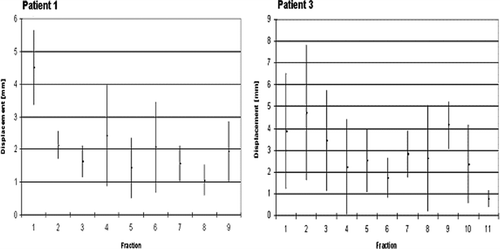
For patients with liver tumors maximum differences >10 mm between IR guided and gated x-ray set-up were found in the longitudinal and lateral direction. The maximum average difference plus SD of ≤6.3 mm in the vertical direction between IR guided and gated x-ray set-up was comparable to the average difference for patients with lung tumors. The maximum average difference plus SD was in the longitudinal direction with ≤11.9 mm and in the lateral direction with ≤12.6 mm considerably larger compared to the patients with lung tumors. It is of note that in the patient with two liver metastases the one located in the periphery of segment eighth showed less respiration dependent movement than those located in the liver center at the porta hepatis. B displays, as example for the considerable random interfraction differences between IR guided and gated x-ray set-up seen in all patients the data for patient 3 with a liver metastasis.
Only for the liver metastasis located at the periphery of segment eight the interfraction differences between the patient with the IR guided and gated x-ray set-up were negligible (data not shown).
The on-target verification under free breathing conditions showed also for the patients with liver tumors a considerable variation between the real and expected intra tumor marker position (). The maximum average difference plus SD between the real and expected marker position was ≤4.8 mm recorded for the fifth patient with a metastasis located at the porta hepatis. B shows for patient 3 that there was also a considerable random interfraction variation between the real and expected intra tumor marker position under gated treatment conditions.
At present none of the patients developed any toxicity. All of the treated lesions are locally controlled. H-SBRT lead to a complete remission in the second patient with a non small cell lung cancer (NSCLC) in the lower lobe of the left lung and in the fifth patient with a liver metastasis in the porta hepatis from breast cancer. In the sixth patient with a liver metastasis at the porta hepatis located from an adenocarcinoma of unknown origin liver metastasis a partial remission was found. However, the fourth developed 3 months following H-SBRT and the fifth at 14 months a regional failure.
Discussion
The risk of adverse normal tissue reactions is one of the most important factors contributing to the success of SBRT in respiratory dependent moving liver and lung tumors. Techniques effectively controlling and correcting potential set-up inaccuracies and organ motion hold the potential to reduce unduly normal tissue irradiation. Conventional set-up accuracy has been reported for non moving tumors to be no better than 1 cm and even worse for tumors showing respiratory dependent movement Citation[17].
We report the implementation and initial clinical experience with regard to the accuracy of patient set-up and treatment feasibility of respiratory dependent moving liver and lung tumors using ExacTrac® Adaptive Gating for real-time image guided respiratory gated H-SBRT.
In recent years several groups have assessed x-ray and infrared systems to improve the conventional set-up accuracy and to introduce gating systems into clinical routine Citation[3–6], Citation[18–27]. The accuracy for these systems has been reported between 0.8 and 1.6 mm under static conditions. Our results indicating an overall system accuracy of 1 mm for the gated Winston Lutz test, and a substantial improvement in the 80–20% penumbra as well as more homogenous dose distributions within the target are in line with previously reported data.
The clinical data show considerable errors after IR guided set-up, which were only detected because of the x-ray gated set-up. This observation reinforces that conventional set-up methods even if facilitated by IR guidance may notably obscure the real set-up accuracy and necessary PTV margins for a respiratory dependent moving target.
The recorded intrafractional differences between expected and actual target position during the exhale phase of the breathing cycle were smaller than the set-up errors. This seems to be in line with observations that in the exhale phase the tumor position seems to be most stable and reproducible Citation[28], Citation[29]. On the other hand, Engelsman et al. Citation[30] reported that the effect of random set-up errors and breathing motion on the cumulative dose is smaller compared to systematic set-up errors. From our data we are at present not able to decide whether this is entirely correct. However, the expected intrafractional tumor motion may be confounded by bony anatomy and incorrect CT or imaging studies prior to treatment, especially in patients with constantly changing breathing pattern. Structures such as the external chest or abdomen motion, breathing cycles determined by air flow, and the diaphragm position detected on fluoroscopic x-rays only allow an indirect verification of tumor position. On the contrary, implanted fiducials allow a comparatively easy direct fluoroscopic x-ray verification of tumor position in real-time with automatic image processing tools such as ExacTrac® Adaptive Gating. Potential disadvantages of implanted fiducial markers are the risk of complications, e.g. infection and pneumothorax, and that they can move in tissue between the planning CT and treatment planning and delivery, and potentially extended treatment times. The coiled-wire design of the VISICOIL™ marker provides extra stability within tumor or tissue and due to its good visibility on fluoroscopy x-ray images translational and rotational topic information. For lung tumors we have chosen to implant fiducials under CT guidance. None of the reported patients with lung tumors developed a pneumothorax. As an alternative we used in two patients with liver tumors ultrasound for the placement of fiducials. The fiducials were well localized in all patients on treatment planning CT-scans and stereoscopic x-ray images. CT-scans during follow-up at least 4 weeks after treatment revealed only minimal if at all migration of fiducials. However, in patients with tumors that can be reached by bronchoscope this approach may be considered as an alternative to the placement under CT guidance. The initial patient experience shows treatment times comparable to other SBRT approaches.
The initial experience with ExacTrac® Adaptive Gating for image guided real-time respiratory gated H-SBRT with regard to the accuracy of patient set-up and treatment of respiratory dependent moving liver and lung tumors will enable us to further assess the technique toward continuous improvement and possibly definition of patient specific margins and beam-on windows for treatment. This may lead to treatment protocols to prove the usefulness of ExacTrac® Adaptive Gating. Adaptive Gating of respiratory dependent moving tumors has the potential to reduce treatment uncertainties due to set-up accuracy and respiratory movement rather than compensating for these via dose margins or delivery of potential excess radiation dose for local tumor control.
We gratefully acknowledge the outstanding dedication and efforts with regard to the integration of image guided respiratory gated H-SBRT into the clinical workflow by S. Bresch, M. Dahms and G. Torwesten (Radiation Therapists at the Department of Radiation Oncology Campus Charité Mitte). The authors of this manuscript have no financial or personal relationships that inappropriately influence the presentation of the results of the present study. However, we acknowledge BrainLAB's support of a university-industry research transfer cooperation initially funded by a grant from the German Bundesministerium für Bildung und Forschung awarded to R. E. Wurm.
References
- Mackie TR, Kapatoes J, Ruchala K, et al. Image guidance for precise conformal radiotherapy. Int J Radiat Oncol Biol Phys 2003; 56: 89–105
- Meeks SL, Tome WA, Willoughby TR, et al. Optically guided patient positioning techniques. Semin Radiat Oncol 2005; 15: 192–20
- Soete G, Van de Steene J, Verellen D, et al. Initial clinical experience with infrared-reflecting skin markers in the positioning of patients treated by conformal radiotherapy for prostate cancer. Int J Radiat Oncol Biol Phys 2002; 52: 694–8
- Wang LT, Solberg TD, Medin PM, et al. Infrared patient positioning for stereotactic radiosurgery of extracranial tumors. Comput Biol Med 2001; 31: 101–11
- Yan H, Yin FF, Kim JH. A phantom study on the positioning accuracy of the Novalis Body system. Med Phys 2003; 30: 3052–60
- Weiss E, Vorwerk H, Richter S, et al. Interfractional and intrafractional accuracy during radiotherapy of gynecologic carcinomas: A comprehensive evaluation using the ExacTrac system. Int J Radiat Oncol Biol Phys 2003; 56: 69–79
- Baroni G, Ferrigno G, Orecchia R, et al. Real-time three-dimensional motion analysis for patient positioning verification. Radiother Oncol 2000; 54: 21–7
- Baroni G, Troia A, Riboldi M, et al. Evaluation of methods for opto-electronic body surface sensing applied to patient position control in breast radiation therapy. Med Biol Eng Comput 2003; 41: 679–88
- Medin PM, Solberg TD, De Salles AA, et al. Investigations of a minimally invasive method for treatment of spinal malignancies with LINAC stereotactic radiation therapy: accuracy and animal studies. Int J Radiat Oncol Biol Phys 2002; 52: 1111–22
- Kupelian PA, Willoughby TR, Meeks SL, et al. Intraprostatic fiducials for localization of the prostate gland: Monitoring intermarker distances during radiation therapy to test for marker stability. Int J Radiat Oncol Biol Phys 2005; 62: 1291–6
- Cosgrove VP, Jahn U, Pfaender M, et al. Commissioning of a micro multi-leaf collimator and planning system for stereotactic radiosurgery. Radiother Oncol 1999; 50: 325–36
- Wurm RE, Cosgrove VP, Schlenger L, et al. Commissioning of a micro-multileaf collimator for conformal stereotactic radiosurgery and radiotherapy. Front Radiat Ther Oncol 1999; 33: 64–77
- Pfaender M, Grebe G, Roll M, et al. Polymer gel dosimetry for conformal radiation therapy with a micromultileaf collimator (mMLC) Z Med Phys 2000; 10: 109–18
- Grebe G, Pfaender M, Roll M, et al. Dynamic arc radiosurgery and radiotherapy: Commissioning and verification of dose distributions. Int J Radiat Oncol Biol Phys 2001; 49: 1451–60
- Common Terminology Criteria for Adverse Events version 3.0 (CTCAE v 3.0), Cancer Therapy Evaluation Program 2003. Available at: http://ctep.cancer.gov/reporting/ctc.html. Accessed.
- Therasse P, Arbuck SG, Eisenhauer EA, Wanders J, Kaplan RS, Rubinstein L, et al. New Guidelines to evaluate the response to treatment in solid tumors. J Natl Cancer Inst 2000; 92: 205–16
- Killoran JH, Kooy HM, Gladstone DJ, Welte FJ, Beard CJ. A numerical simulation of organ motion and daily setup uncertainties: Implications for radiation therapy. Int J Radiat Oncol Biol Phys 1997; 37: 213–21
- Meeks SL, Bova FJ, Wagner TH, et al. Image localization for frameless stereotactic radiotherapy. Int J Radiat Oncol Biol Phys 2000; 46: 1291–9
- Verellen D, Soete G, Linthout N, et al. Quality assurance of a system for improved target localization and patient set-up that combines real-time infrared tracking and stereoscopic X-ray imaging. Radiother Oncol 2003; 67: 129–14
- Chang SD, Main W, Martin DP, et al An analysis of the accuracy of the CyberKnife: A robotic frameless stereotactic radiosurgical system. Neurosurgery 2003;52:140–6; Discussion 146–7.
- Murphy MJ, Cox RS. The accuracy of dose localization for an image-guided frameless radiosurgery system. Med Phys 1996; 23: 2043–9
- Ramsey CR, Cordrey IL, Oliver AL. A comparison of beam characteristics for gated and nongated clinical x-ray beams. Med Phys 1999; 26: 2086–209
- Hugo GD, Agazaryan N, Solberg TD. An evaluation of gating window size, delivery method, and composite field dosimetry of respiratory-gated IMRT. Med Phys 2002; 29: 2517–25
- Duan J, Shen S, Fiveash JB, et al. Dosimetric effect of respiration-gated beam on IMRT delivery. Med Phys 2003; 30: 2241–52
- Phillips MH, Singer K, Miller E, et al. Commissioning an image-guided localization system for radiotherapy. Int J Radiat Oncol Biol Phys 2000; 48: 267–76
- Yu C, Main W, Taylor D, et al. An anthropomorphic phantom study of the accuracy of Cyberknife spinal radiosurgery. Neurosurgery 2004; 55: 1138–49
- Yin F-F, Das S, Kirkpatrick J, Oldham M, Wang Z, Zhou S. Physics and imaging for targeting of oligometstases. Sem Radiat Oncol 2006; 16: 85–101
- Seppenwoolde Y, Shirato H, Kitamura K, et al. Precise and real-time measurement of 3D tumor motion in lung due to breathing and heartbeat, measured during radiotherapy. Int J Radiat Oncol Biol Phys 2002; 53: 822–34
- Ross CS, Hussey DH, Pennington EC. Analysis of movement of intrathracic neoplasms using ultrafast computerized tomography. Int J Radiat Oncol Biol Phys 2000; 48: 81–7
- Engelsman M, Damen EM, De Jaeger K, et al. The effect of breathing and setup errors on the cumulative dose to a lung tumor. Radiother Oncol 2001; 60: 95–105