Abstract
Background. Electron spin resonance (EPR) is used to determine the absorbed dose of alanine dosimeters exposed to clinical photon beams in a solid-water phantom. Alanine is potentially suitable for medical reference dosimetry, because of its near water equivalence over a wide energy spectrum, low signal fading, non-destructive measurement and small dosimeter size. Material and Methods. A Bruker EMX-micro EPR spectrometer with a rectangular cavity and a measurement time of two minutes per dosimeter was used for reading of irradiated alanine dosimeters. Under these conditions a new algorithm based on scaling of known spectra was developed to extract the alanine signal. Results. The dose accuracy, including calibration uncertainty, is less than 2% (k=1) above 4 Gy (n=4). The measurement uncertainty is fairly constant in absolute terms (∼30 mGy) and the relative uncertainty therefore rises for dose measurements below 4 Gy. Typical reproducibility is <1% (k=1) above 10 Gy and <2% between 4 and 10 Gy. Below 4 Gy the uncertainty is higher. A depth dose curve measurement was performed in a solid-water phantom irradiated to a dose of 20 Gy at the maximum dose point (dmax) in 6 and 18 MV photon beams. The typical difference between the dose measured with alanine in solid water and the dose measured with an ion chamber in a water tank was about 1%. A difference of 2% between 6 and 18 MV was found, possibly due to non-water equivalence of the applied phantom. Discussion. Compared to previously published methods the proposed algorithm can be applied without normalisation of phase shifts caused by changes in the g-value of the cavity. The study shows that alanine dosimetry is a suitable candidate for medical reference dosimetry especially for quality control applications.
Radiation oncology requires a highly accurate delivery of dose to the patient. Dose errors of the order of 7% are clinically detectable and 5% errors can result in 10–20% changes in tumour control probability and up to 20–30% changes in normal tissue complication probabilities Citation[1]. New radiotherapy modalities (for example, intensity-modulated radiation therapy, volumetric arc therapy, or radiotherapy with heavy charged particles) are introduced in the clinics and challenge the requirement for dosimetry in connection with accelerator commissioning, dose verification and other quality control procedures. As a supplement to ionometry and calorimetry (that are difficult to apply in small fields) standard laboratories like National Physics Laboratory (NPL), UK, and Physikalisch-Technische Bundesanstalt (PTB), Germany, are developing reference dosimetry based on EPR of alanine Citation[2–4]. This paper presents a new method for analyzing EPR alanine spectra using new spectrometer facilities at Risø-DTU and investigates the capabilities of the method in the dose range from 1 to 100 Gy for clinically relevant beams.
Electron spin resonance (EPR) measurements can be used to determine the amount of free radicals generated in a sample of alanine as a result of exposure to ionizing radiation Citation[5–7]. Alanine has several features that make it suitable for medical dosimetry: near water equivalence (Z = 6.2) Citation[8] and thus a low energy dependence of the response over a wide energy range, low fading, non-destructive measurement and small dosimeter size (ø5×3 mm). Correction factors are small compared to e.g. TLD dosimetry. If the dosimeters are irradiated in a pre-packed phantom, the downtime of the accelerator can be limited to a few minutes. However, there are also some drawbacks that have to be taken into consideration: EPR spectrometers are expensive and at therapeutic dose levels the alanine signal is affected significantly by noise.
Methods
Alanine dosimeters
Alanine dosimeters from Harwell Dosimeters (Batch AH592C) were used. These dosimeters consisted of 90.9% L-alpha-alanine and 9.1% paraffin wax as binder. The dosimeters were 4.8 mm in diameter and 2.8 mm in height.
The temperature coefficient of the alanine dosimeters used has been measured at Risø High Dose Reference Laboratory to be +0.14% °C−1. The EPR response of alanine is reported to be 0.3 to 0.6% lower in clinical photon beams than when irradiated with Cobalt-60 Citation[9–13]. A correction factor of 1.006 was used in this work.
Verification of calibration
Eleven sets of four alanine dosimeters were irradiated to doses between 1 and 100 Gy in a Cobalt-60 gammacell at NPL. The irradiation was carried out at 16°C. Dose is delivered as dose to water and is traceable to a primary national standard with an accuracy of 1.4% (k=1).
Depth dose measurements in solid-water phantom
A solid-water phantom (Gammex), consisting of 30×30 cm slabs with a total height of 35 cm, was used. Slabs with thicknesses of 5, 20 and 50 mm were used. 6 and 18 MV clinical photon beams at Copenhagen University Hospital were utilized.
The field size was 10×10 cm at a source to surface distance (SSD) of 100 cm. The phantom was irradiated to a dose of 20 Gy at the maximum dose point (dmax). A total of four irradiations were carried out, two at 6 MV and two at 18 MV photons. The phantom was irradiated at room temperature (21°C).
For each depth, five dosimeters were placed in a three cm wide cross-like pattern in the centre of the field.
Ion chamber measurements
A measurement of the depth dose curve in water was performed using a PTW semiflex tube chamber (type 31002, active volume 0.125 cm3, cavity diameter 0.55 cm). The measured depth dose curve was normalized to 20 Gy at dmax. The overall uncertainty of the measurement is estimated to 1.4% (k=1). The major components of the measurement uncertainty are the conversion factor (cf. TRS-398) Citation[14] and uncertainties due to the experimental setup.
EPR measurements
A Bruker EMX-micro EPR spectrometer was used for measurements of the alanine dosimeters. The spectrometer is equipped with a rectangular cavity. This type of cavity has a higher sensitivity than a circular cavity, but is also more sensitive to changes in environmental parameters such as temperature and humidity. The spectrometer is placed in an air-conditioned room (22±1°C) and the cooling water to the micro-wave bridge is controlled to ±0.02°C.
To acquire the alanine spectrum the dosimeter was placed in a quartz tube inserted into the cavity and six 20 seconds scans were performed with a 90° rotation of the dosimeter between the 3rd and 4th scan. The measurement parameters are:
microwave power: 8 mW
modulation amplitude: 1.0 mT
field sweep from 346 to 354 mT
time constant: 21 ms
conversion time: 21 ms
The reference frequency of the rectangular cavity is prone to small frequency shifts, i.e. that the spectrum is shifted along the magnetic field axis depending of the load of the cavity. Frequency shifts may result from e.g. rotating or replacing the quartz tube.
Data analysis
The algorithm used to derive the absorbed dose based on the measured alanine spectra is based on a method developed by NPL Citation[2], Citation[12]. We have modified the algorithm to make it suitable for high sensitivity cavities where shifts in the x-axis of the measured spectra can occur.
a and 1b show a background spectrum and the spectrum of a dosimeter irradiated to 1 Gy, respectively. c shows a 100 Gy spectrum for comparison. For doses above 20 Gy a simple peak-to-peak measurement of the central peaks of the alanine spectrum can be used as the measurement signal, but for doses below 20 Gy other methods must be used to obtain the level of accuracy that is required in medical dosimetry.
Figure 1. Typical EPR spectra of alanine: A) Background spectrum. B) Dosimeter irradiated to 1 Gy. C) Dosimeter irradiated to 100 Gy.
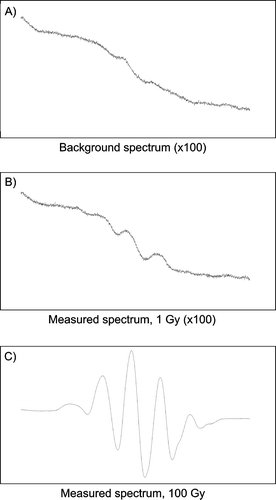
The algorithm used in this work is using the measured spectrum of a given dosimeter, together with spectra of the background (un-irradiated dosimeters) and of dosimeters irradiated to a known dose with a high signal-to-noise ratio (e.g. 100 Gy).
The background spectrum is subtracted from both the spectrum from the dosimeter in question and the known spectrum. The position of the central peaks of the alanine spectrum is determined from the known spectrum (see ). The EPR intensities between the central peaks of the given alanine spectrum are plotted as function of the corresponding intensities in the reference spectrum. The slope determines the ratio of amplitude between the known spectrum and the spectrum from the dosimeter in question, and a first estimate of the dose is found by multiplying the slope with the known dose. The slope is found by a least-square fit of the part of the segment that gives the highest R2-value.
Figure 2. Determination of ratio between the amplitude of EPR spectra of dosimeters irradiated to known dose and dosimeters for which the dose is to be evaluated. A) The segment between the main peaks of the alanine EPR spectrum is used for the analysis. B) The EPR response of the dosimeter in question is plotted as a function of the EPR response of the dosimeter irradiated to a known dose. The slope determines the ratio of amplitude.
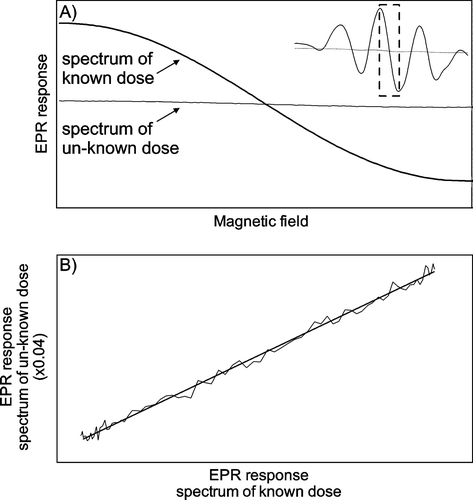
The advantage of this method is that it is not sensitive to small shifts in the x-axis, because such a shift merely will result in an offset but not a change of the slope.
As in the algorithm developed by NPL a pellet-dependent background is found and subtracted from the original spectrum and the algorithm is repeated until the estimated dose value converges, usually after 2–3 iterations.
In this work, the known spectrum is based on dosimeters irradiated to 100 Gy at NPL. The spectrum is composed as an average of four dosimeters, each scanned 12 times to obtain a high signal-to-noise ratio. The 100 Gy dosimeters are together with un-irradiated dosimeters measured in the following sequence: one before, two during and one after the measurements of the dosimeters for which the dose is calculated.
Results
Verification of calibration
A linear response is assumed up to 100 Gy and this is verified by measuring dosimeters irradiated to known doses traceable to a primary standard in the range from 1 to 100 Gy at NPL.
Reconstructed dose as a function of the dose certified by NPL is shown in a. A one-to-one relationship is observed with an offset of 30 mGy. The general trend that the certified dose is overestimated by a few tens of milli-grays is confirmed by the average residual of 19 mGy. The average standard deviation is 107 mGy (b). The magnitude of the residual and standard deviation is nearly constant below 10 Gy. The average residual is less than 1% for doses from 4 Gy and above (c).
Figure 3. A) Reconstructed dose as a function of dose certified by NPL. Solid line: one-to-one ratio. B) Average residual in Gy. C) Average residual in percent. The error bars show the standard deviation of the mean (n=4).
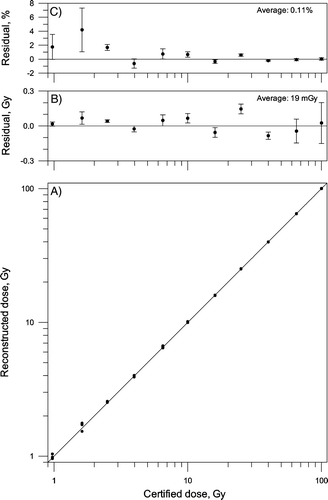
The standard deviation is below 0.8% for measurements above 10 Gy, below 1.5% for measurements between 2.5 and 10 Gy, and below 7% for measurements between 1.0 and 2.5 Gy.
Depth dose measurements in solid-water phantom
Sets of five dosimeters were irradiated at different depths in a solid-water phantom with 6 MV and 18 MV photons. Two irradiations were performed for each energy and the phantom was irradiated to 20 Gy at dmax. and show depth dose curves given by alanine dosimetry and the ionization chamber, together with residuals between the estimates. No corrections for the effective point of measurement were made Citation[15].
Figure 4. Depth dose curve of 6 MV photons. A) Solid line: Measured in water using a PTW semiflex ionisation chamber in water. Circles: Measured with alanine dosimeters placed in a solid-water phantom. Two experiments were made. B) Average residual in percent. The error bars show the standard deviation of the mean (n=5).
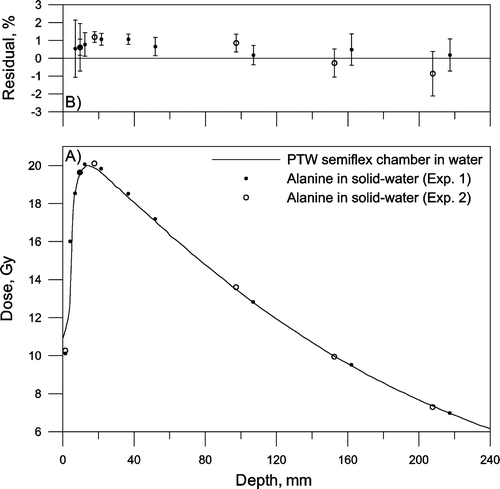
Figure 5. Depth dose curve of 18 MV photons. A) Solid line: Measured in water using a PTW semiflex ionisation chamber in water. Circles: Measured with alanine dosimeters placed in a solid-water phantom. Two experiments were made. B) Average residual in percent. The error bars show the standard deviation of the mean (n=5).
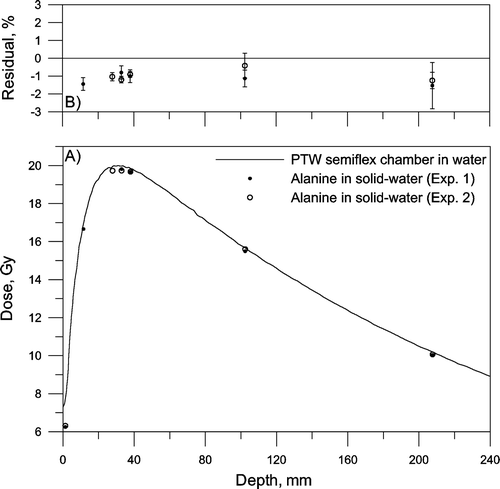
For both photon energies the residual is close to ±1% at dmax (15 mm and 32 mm) Citation[16], comparable with the combined measurement and calibration uncertainty of the EPR system at 20 Gy (1.4%, k=1; measurement uncertainty: 0.7%, calibration uncertainty: 1.2%). There seems to be a significant difference of about 2% between alanine irradiated with 6 and 18 MV photons in solid-water. This deference could possibly be explained by energy dependence of the solid-water material or alanine, or by differences between the experimental setup and the reference data.
Discussion and conclusions
This paper demonstrates the feasibility of EPR alanine dosimetry in clinical beams using a newly developed algorithm aimed at measurement in the 1–100 Gy range with a rectangular-cavity EPR spectrometry (Bruker EMX micro).
A rectangular cavity was used for reading the alanine dosimeters. This type of cavity is more sensitive than a circular cavity, and is therefore more susceptible for environmental influences and frequency shifts as discussed below.
To correct for sensitivity changes, caused by environmental influences such as humidity and temperature, a chrome-reference marker (supplied by Bruker) can be mounted in a fixed position inside the cavity. The signal of the reference marker is located outside the main alanine signal, and the changes in the amplitude of the reference marker signal can be used to correct for sensitivity changes of the EPR spectrometer. Initially the magnitude of the reference marker signal was set to be equivalent to an alanine signal of approximately 10 Gy. However, for the instrument settings used in this experiment, corrections based on the reference marker only added to the overall uncertainty, and the use of the reference marker was omitted. As an alternative the stability of the EPR spectrometer can be checked by measuring dosimeters irradiated to known doses during the measurement session.
A change of the g-value of the cavity results in a shift in the resonance frequency of the cavity that is reflected in a shift of the spectrum along the x-axis. A frequency shift can be caused by e.g. differences in humidity, positioning of the dosimeters or if different quartz tubes are used to mount the dosimeter in the cavity. Therefore scaling a reference spectrum to reconstruct a given alanine spectrum will result in a less accurate dose estimate if the two spectra are not aligned. The shift between two spectra can in principle be calculated if the microwave frequency is recorded during measurement. A normalized cross-correlation function can also be used to align the spectra Citation[4].
The new scaling algorithm can be applied without frequency normalisation or prior knowledge about changes in the g-value between spectra.
We measured depth dose curves in a solid-water phantom irradiated to 20 Gy at dmax for 6 and 18 MV photons. Although of practical interest few publications are actually available in the open literature that investigates alanine dosimeters placed in solid phantoms irradiated with clinical x-ray beams Citation[17–19]. We found excellent agreement between the 6 MV depth dose curves measured with alanine in the solid-water phantom and the ion chamber reference data measured in liquid water. We did, however, note a significant difference of about 2% between alanine irradiated with 6 and 18 MV photons in solid-water. Further studies are needed to establish if this difference is caused by non-water equivalence of the applied solid-water phantom or alanine, or by differences between the experimental setup and the reference data.
A formal uncertainty budget has not been established, but the uncertainty of the individual measurements is assessed to be below 2% for doses above 4 Gy for a readout time of only two minutes per dosimeter. It is possible to improve the accuracy by prolonging the measurement time or by optimisation of equipment and algorithms. Other groups are considering other EPR-materials than alanine Citation[20], Citation[21].
In agreement with other studies Citation[2], Citation[4], Citation[22], Citation[23] the study suggest that alanine dosimetry indeed is a suitable candidate for medical reference dosimetry, for a wide range of applications, because of its near water equivalence, small and well-known correction factors.
Contrary to TLD dosimeters the readout of alanine is non-destructive and coupled with a low fading in the order of 1% per year Citation[24] it is possible to re-measure alanine dosimeters at any given time. The ability to retain the intact dosimeter for documentation makes alanine dosimetry ideal for quality assurance, e.g. during commissioning or periodical checks of medical accelerators. For quality control applications the dose can be raised to a level where the measurement uncertainty is small.
Acknowledgements
This work was supported by the Danish Cancer Society under the contract: “The dosimetric impact of respiratory motion in breast cancer radiotherapy”.
References
- Chetty IJ, Curran B, Cygler JE, DeMarco JJ, Ezzell G, Faddegon BA, et al. Report of the AAPM Task Group No. 105: Issues associated with clinical implementation of Monte Carlo-based photon and electron external beam treatment planning. Med Phys 2007; 34: 4818–53
- Sharpe PHG, Rajendran K, Sephton JP. Progress towards an alanine/ESR therapy level reference dosimetry service at NPL. Appl Radiat Isot 1996; 47: 1171–5
- Proceedings of the 216th PTB Seminar. Alanine Dosimetry for clinical applications. May 8th–9th 2006. Physikalisch-Technische Bundesanstalt, Seminarzentrum Kohlrausch-Bau, Bundesallee 100, 38116, Braunschweig, Germany.
- Anton M. Development of a secondary standard for the absorbed dose to water based on the alanine EPR dosimetry system. Appl Radiat Isot 2005; 62: 779–95
- Bradshaw WW, Cadena DG, Crawford GW, Spetzler HAW. The use of alanine as a solid dosimeter. Radiat Res 1962; 17: 11–21
- Regulla DF, Deffner U. Dosimetry by ESR spectroscopy of Alanine. Int J Appl Radiat Isot 1982; 33: 1101–14
- American Society for Testing and Materials ASTM. Standard practice for use of the Alanine-EPR dosimetry system, 1999. ASTM E1607–9, June.
- McEwen MR, Niven D. Characterization of Virtual Water™ for dosimetry. Med Phys 2006; 33: 876–87
- Anton M, Kapsch R-P, Krystek M, Renner F. Response of the alanine/ESR dosimetry system to MV x-rays relative to 60Co radiation. Phys Med Biol 2008; 53: 2753–70
- Sharpe PHG. Progress report on radiation dosimetry at NPL, BIPM report CCRI(I)-03-14 (Sèvres).
- Zeng GG, McEwen MR, Rogers DWO, Klassen NV. An experimental and Monte Carlo investigation of the energy dependence of alanine/EPR dosimetry: I. Clinical x-ray beams. Phys Med Biol 2004; 49: 257–70
- Sharpe PHG, Sephton JP. Therapy level alanine dosimetry at the NPL. In: Proceedings of the 216th PTB Seminar, Alanine Dosimetry for clinical applications, 2006, PTB, Braunschweig, Germany.
- Olsen KJ, Hansen JW, Wille M. Response of the alanine radiation dosimeter to high-energy photon and electron beams. Phys Med Biol 1990; 35: 43–52
- IAEA TRS-398. Absorbed dose determination in external beam radiotherapy: An International Code of Practice for Dosimetry based on standards of absorbed dose to water. International Atomic Energy Agency, Vienna, 2000, ISSN 1011-4289.
- Ramani R, Kohli K, Cao F, Heaton R. Dosimetric evaluation of Plastic Water Diagnostic Therapy. J Appl Clin Med Phys 2008; 9: 98–111
- BJR Supplement 25. Central axis depth dose data for use in radiotherapy: 1996. Published by the British Institute of Radiology.
- Østerås BH, Hole EO, Olsen DR, Malinen E. EPR dosimetry of radiotherapy photon beams in inhomogeneous media using alanine films. Phys Med Biol 2006; 51: 6315–28
- Kuntz F, Pabst JY, Delpech JP, Wagner JP, Marchioni E. Alanine-ESR In Vivo dosimetry: A feasibility study and possible applications. Appl Radiat Isot 1996; 47: 1183–8
- Feist H, Regulla D, Wieser A. Is Alanine/ESR dosimetry now an alternative to ferrous sulphate dosimetry?. Appl Radiat Isot 1993; 44: 47–51
- Gustafsson H. Development of sensitive EPR dosimetry methods. Linköping University Medical Dissertations, No. 1044, 2008. Division of Radiation Physics, Department of Medical and Health Sciences, Faculty of Health Sciences, Linköping University, SE-58185 Linköping, Sweden.
- Lund E, Gustafsson H, Danilczuk M, Sastry MD, Lund A, Vestad TA, et al. Formates and dithionates: Sensitive EPR-dosimeter materials for radiation therapy. Appl Radiat Isot 2005; 62: 317–24
- Olsen KJ, Hansen JW, Waligórski MPR. ESR Dosimetry in calibration intercomparisons with high-energy photons and electrons. Appl Radiat Isot 1989; 40: 985–8
- Bassler N, Hansen JW, Palmans H, Holzscheiter MH, Kovacevic S, the AD-4/ACE Collaboration. The antiproton depth-dose curve measured with alanine detectors. Nucl Instr Meth Phys Res B 2008;266:929–36.
- Arber JM, Sharpe PHG. Fading characteristics of irradiated alanine pellets: The importance of pre-irradiation conditioning. Appl Radiat Isot 1993; 44: 19–22