Abstract
Radiation therapy for cancer is considered to be immunosuppressive. However, the cellular response after radiation therapy may stimulate or suppress an immune response. The effect may vary with the tumor type and occasionally tumor regressions have been observed outside the irradiated volume, both in animal studies and in the clinic. A renewed interest in the role of immunity for the observed effect of radiation came with the current recognized role of immune checkpoint blockers (ICBs) for control of selected cancer types. We therefore here review preclinical studies and clinical reports on the interaction of ICBs and radiation as a basis for further clinical trials. Some tumor types where the combination of these modalities seems especially promising are also proposed.
Introduction
The 5-year survival is currently about 70% for all cancers [Citation1,Citation2]. Cure is generally ascribed to surgery (49%), radiation therapy (40%) and drugs (11%), but several modalities are often used simultaneously and therefore the role of each modality can be difficult to define. Radiation therapy is considered necessary for about 50% of all cancer patients [Citation3]. The technology for administering radiation is rapidly expanding [Citation4,Citation5] and the implementation of particle radiation (proton and carbon ion therapy) is ongoing [Citation6–8].
Immune checkpoint blockers (ICBs) are now approved for treatment of malignant melanomas, Hodgkin’s lymphoma, lung cancer, renal cancer and tumors with microsatellite instability such as colorectal cancer [Citation9]. Despite many durable responses, the effect is varying within the same tumor entities and only a subset of these tumors respond to ICB treatment. In this paper we review published experimental and clinical studies which have addressed the possibility for combining radiation therapy with ICBs to increase the tumor response.
Methods
The literature was searched for English papers using PubMed with search words ‘immune surveillance’, ‘immunotherapy’, ‘immune checkpoint blockers’, immune checkpoint inhibitors’, ‘immunologic cell death’, ‘radiation’, ‘radiation therapy’, ‘immunotherapy’, ‘immunology’ or combinations of these terms. Specific terms like ‘apoptosis’, ‘autophagy’ and ‘abscopal response’ related to the topics addressed were also used. The reference lists of the selected papers were then scrutinized and all abstracts for relevant papers checked for references used in this narrative review.
Basic radiobiology
Cell killing
Classic radiobiology describes the inactivation of living cells by radiation therapy as clonogenic cell death. The radiation effect in cell lines is given by the surviving fraction, the percentage of cells with ability to form colonies of at least 50 daughter cells which is considered to imply a capacity for unlimited proliferation [Citation10]. The underlying biological mechanisms are considered to be induction of damage caused directly by ionization of DNA or indirectly via reactions of aqueous free radicals formed around DNA [Citation11,Citation12]. It has been calculated that an X-ray dose of 1 Gray (Gy) produces about 3000 damaged bases, 1000 single-strand breaks (SSBs) and 40 double-strand breaks (DSBs) per single cell [Citation13]. However, essentially all the base damage and SSBs are repaired by specialized base repair enzymes or the base excision repair (BER) system, while the majority DSBs are repaired by non-homologous end joining (NHEJ) and homologous recombination (HR) [Citation14].
Radiation influences plasma membranes especially at doses above 10 Gy by dose-dependent activation of acid sphingomyelinase which hydrolyzes sphingomyelin to ceramide, a second messenger which induces apoptosis, and by de novo mitochondrial synthesis of ceramide [Citation15]. In summary, the effects of radiation are production of reactive oxygen species (ROS) which in addition to the effect on DNA interact with numerous cellular targets which regulate the fate of the cell through changes in vital structures controlled by p53 and DNA damage checkpoint control kinases (CHK1 and CHK2) [Citation11,Citation16]. After irradiation, cell growth is arrested so that the damage can be repaired, otherwise, cell death occurs. It is possible to measure this phenomenon by counting stained vital cells and dead cells. The cellular death is the result of several mechanisms such as apoptosis [Citation17,Citation18], autophagy [Citation19,Citation20], mitotic catastrophe [Citation21–23], necrosis and senescence [Citation24–26]. Most reviews find that the final cause of death is apoptosis or a form of necrosis, both may be interrelated [Citation27]. Thus mitotic catastrophe may cause a prolonged growth arrest leading to apoptosis or necrosis dependent on the mutation status of p53 [Citation21,Citation23] or death in senescence [Citation22]. While it was previously considered that apoptosis led to a tolerogenic immunologic effect and only necrosis could expose intracellular antigens, it is now assumed that there may be some immunological stimulation after radiation by both death types, but not strong enough to induce immunological clearance of an established cancer.
Survival response after radiation
In addition to inducing DNA damage which can be lethal unless repaired, radiation also induce a strong pro-survival response. Radiation may therefore simultaneously both kill cells and stimulate a survival response [Citation28,Citation29]. Later a similar response has been documented also after chemotherapy [Citation30,Citation31].
Autophagy induced by radiation is considered as a mechanism promoting tumor survival, growth, invasion and spread [Citation19]. Autophagy provides recycling of molecules for energy production and molecules necessary for growth [Citation20]. However, whether autophagy contributes to tumor growth is still not settled [Citation32]. Another early pro-survival effect is the triggered stimulation of hypoxia-inducible factor 1α (HIF-1α) which is a transcription factor for proteins involved in cell metabolism associated with tumor progression (marked red in ) [Citation33]. Survivin is another activated molecule which inhibits apoptosis and thereby cell death [Citation34].
Figure 1. Mechanisms for stimulation of cytotoxic T-cells (CD8 T-cell) by radiation (IR). Immune stimulating factors marked green, inhibition marked red. After radiation tumor cells release danger or damage associated molecules (DAMPs), high mobility group B 1 (HMGB1), calreticulin, ATP and heat shock proteins (Hsp’s) together with neoantigens. The neoantigens bind to major histocompatibility complex type I (MHC1) on immature antigen presenting cells (APC) which then moves to the lymph nodes where it as dendritic cell (DC) present the neoantigens to T-cell receptors on CD8 T-cells. After stimulation, the CD8 T-cells then move systemically and can attach tumor cells with the specific neoantigens which triggered the CD8 T-cells. Tumor cells also express transforming growth factor β (TGF-β), interferons (IFN) and hypoxia inducible factor 1-α (HIF 1-α) which protects against immune control. (Illustration: Kristin Risa).
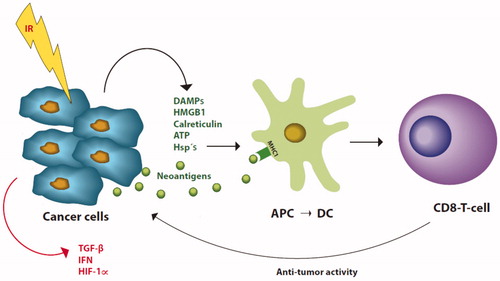
RT and immunosuppression
By previous clinical radiation techniques, large volumes of normal tissues were included in the treatment volumes to cover the macroscopic and microscopic tumor deposits. Often the blood counts, hemoglobin and leucocytes decreased during therapy, and radiation has therefore been considered an immunosuppressive treatment [Citation35–37]. Total body (lymph node) irradiation used to control hematological malignancies is also immunosuppressive. Radiation stimulates production of cytokines, of which transforming growth factor β1 (TGF-β1) has many effects, including inactivation of natural killer cells (NK cells) and stimulation of expression of programmed death receptor ligand 1 (PD-L1), thus protecting the damaged cancer cell from immune surveillance [Citation38]. The protective effect is also mediated through secretion of TGF-β1, Toll-like receptors, nitrogen oxide (NO), different cytokines like interleukin (IL)-10 [Citation38–42].
Microenvironment
It is well known that a low oxygen level can reduce the cellular sensitivity for photon radiation by a factor of about 3. Hypoxic tumors are therefore less likely to be cured by radiation. It is of interest that HIF-1α which increases under hypoxic conditions, in turn, increases cellular expression of PD-L1 which dampens the activity of CD8 T-cells by binding to PD-1 receptors on the surface of these cells [Citation43]. Other factors like IL-1β, IL-6, tumor necrosis factor α (TNFα) and vascular endothelial growth factor (VEGF) produced in the tumor environment stimulates growth of vessels thus improving growth conditions for the tumor cells [Citation44]. Type 2 macrophages, regulatory T-cells (CD4 T-cells) and bone marrow-derived mononuclear suppressor cells surrounding a tumor may also limit the access of infiltrating CD8 T-cells to reach the tumor cells [Citation45].
Immunological effects of radiation therapy
Radiation exposure can induce danger signals on the tumor cell surface which may activate immune cells and macrophages [Citation46]. After radiation exposure immune stimulating molecules appear on to the surface of tumor cells termed danger or damage associated molecular pattern molecules (DAMPs) which function as signals for the immune system’s immature antigen presenting cells (APC) [Citation47–49]. Typical DAMPs are calreticulin, high mobility group box 1 (HMGB1), heat shock protein 70 and 90 (Hsp 70, Hsp 90), extracellular adenosine triphosphate (ATP), S100A4 and uric acid [Citation48–52] (). DAMPs act also as chemo-attractants, which activate immature dendritic cells and attracts macrophages, granulocytes and eosinophils [Citation53–55]. Radiation damage may cause release of double-strand DNA to the cytoplasm, with production of interferon type I via cyclic GMP-AMP synthase (cGAS) and stimulator of interferon genes (STING) pathways, leading to activation of dendritic cells and priming of CD8 T-cells [Citation56,Citation57]. However, if the amount of double-stranded DNA is high in the cytoplasm it activates three prime repair exonuclease (Trex 1) which is an endonuclease that inhibits the immune stimulation [Citation58]. Interferon also stimulates the CD4 T-cells (regulatory T-cells) which generally inhibit CD8 T-cells, but seems to stimulate dendritic cells and the priming of CD8 T-cells in an initial phase. It is also documented that high dose radiation inhibits immune suppression by depletion of myeloid-derived mononuclear suppressor cells, type 2 macrophages, cancer-associated fibroblasts and regulatory T-cells and increase infiltration of CD8 T-cells [Citation59–62]. Low dose radiation can normalize aberrant vasculature and reprogram macrophages from type M2 to type M1 in the tumor microenvironment [Citation62]. The mechanisms described may be tissue and tumor specific and also depend on the dose and fractionation regimens.
Radiation therapy is generally considered to be a local treatment. However, occasionally response in tumor deposits outside the treated volume has been reported, thus suggesting a systemic effect [Citation63–66]. The phenomenon has been described as an abscopal (out of field) effect [Citation67–69]. This rare response can be experimentally shown when mice are treated by radiation and immune modulating therapy [Citation70]. For instance, when Fms-related tyrosine kinase 3 ligand (Flt-3L), a stimulator of hematological cells, was administered to mice inoculated with a mammary carcinoma in both flanks, an abscopal response was observed also in the un-irradiated tumor after radiation to the contralateral flank, but only in immunocompetent mice [Citation71]. The results indicate that the abscopal response is an immunological effect which needs at least functional cytotoxic CD8 T-cells to control the unirradiated tumors [Citation72,Citation73]. In the clinic, abscopal responses have been observed when radiation was administered as palliation after failure of tumor control by ipilimumab mostly in melanoma patients [Citation74–76]. In reviews, the abscopal response occurred 5–6 (range 0–24) months after radiation with a mean duration of 15–19 months [Citation76–78]. Such case reports indicate that combination of inhibitors of CTLA-4 and PD-1/PD-L1 with radiation can make the rare abscopal response a more common phenomenon [Citation70,Citation79,Citation80].
Preclinical combination of radiation and ICBs
Many tumors do not respond to monotherapy with ICBs, therefore researchers have tried to combine these drugs with vaccination regimens in several animal experiments [Citation81,Citation82]. Radiation can be seen as an in situ vaccination. Due to the known activation of DAMPs by radiation, this modality was used in combination with antibodies against CTLA-4, PD-1 and PD-L1 with success in mice () [Citation83]. Not only was a clear growth delay documented, but also a significantly improved survival of the mice, as well as effect on secondary tumors outside the radiated field. Re-challenge with the original tumor cells in mice after a complete response failed to form new tumors. In separate studies, it was documented that radiation increased the expression of major histocompatibility complex 1 (MHC-1) in tumor cells [Citation83–86], stimulated maturation of dendritic cells and increased danger signals and tumor-associated antigens (neoantigens) and adhesion molecules [Citation87,Citation88], and changes in the microenvironment [Citation89]. Radiation seems also to enhance the expression of T-cell receptors in CD8 T-cells [Citation90]. Radiation can modulate both stimulatory and inhibitory signaling molecules for CD8 T-cells [Citation91]. The animal studies include the first generation of experimental triple therapy (two antibodies and radiation) which further increase the response in murine tumors [Citation92,Citation93]. Several new candidate targets for ICBs are identified [Citation94,Citation95], and other factors like the TNF system (CD137), natural killer cells and complement may also modulate the combined effect [Citation96–98].
Table 1. Experimental studies on combination of radiation and immune checkpoint blockers.
Timing and scheduling
In experimental studies, positive results have been shown after giving various ICBs and radiation (10–30 Gy) concurrent (±1 day) and after radiation (10–15 Gy) [Citation92,Citation97,Citation99–104]. Best effect was observed when the drug was administered on day 1 compared to day 5 in a 2 Gy x 5 fractionated regimen or 7 days after radiation [Citation105]. In another study inhibition of CTLA-4 was most effective when administered 7 days before 20 Gy radiation in a breast carcinoma model [Citation106]. High doses (20 Gy or 12 Gy) were better than 3 Gy x 5 in other experiments [Citation39,Citation145].
Concurrent radiation and immunotherapy have been the standard for assessing abscopal responses in animals. In the clinic, the abscopal response has been observed when local radiation has been given after ipilimumab or PD-1/PD-L1 in patients with metastases. It is difficult to separate an abscopal response from an expected systemic effect of an ICB after radiation. A phase I trial showed longer than expected progression-free survival after previous radiation in advanced non-small cell lung cancer (NSCLC) patients treated with pembrolizumab [Citation107]. Treatment with durvalumab 1–42 days after chemoradiotherapy resulted in significantly longer progression-free survival (16.8 versus 5.6 months) and overall survival (2-year survival 66.3% versus 55.6%, respectively) in a large randomized trial [Citation108,Citation109]. These important studies have changed the treatment for advanced NSCLC. In malignant melanoma concurrent radiation and ipilimumab improved overall survival to 19 months compared to 10 months after ipilimumab alone [Citation110]. At present different schedules are under investigation, the best timing and radiation dose, volume and fractionation may depend on the tumor type and site as well as the type of ICB.
Particle therapy
As with photon therapy, tumor cells surviving proton radiation display immunogenic modulation by increasing surface molecules like calmodulin, MHC 1 (HLA complex) and tumor-associated antigens which improve their sensitivity for CD8 T-cells [Citation111]. Local treatment with high dose (30 Gy) carbon ions alone or 6 Gy combined with stimulated dendritic cells reduced the number of lung metastases in a mouse model [Citation112]. Both proton and carbon ion radiation decreased migration, invasion and spread of human fibrosarcoma cells in vitro, in contrast to photon radiation [Citation113]. In another study carbon ion radiation controlled most squamous cell carcinoma tumors in immune competent C3H mice and restricted growth of secondary tumor inoculations, while tumor growth continued in nude mice [Citation114]. The good local control by particle therapy may further benefit from improved control of distant metastases by combination with ICBs [Citation115].
Radiation, hyperthermia and immune therapy
It is well documented in clinical studies that hyperthermia increases the effect of radiation [Citation116]. Multiple mechanisms are involved, like increased DNA damage, change in microenvironment and immune mechanisms, i.e., surface expression of interferon-γ and Hsp70, HMGB1, interferon-γ and Hsp70 which present antigens to and stimulate immature dendritic cells and activate natural killer cells [Citation116–118]. Hyperthermia may therefore potentially contribute to more effective immune response if combined with radiation and ICBs.
Clinical results of combining ICB and radiation
As presented above the introduction of ICBs is a paradigm shift in immunotherapy which represents an important improvement for tumor control in a limited number of tumors which until now have been difficult to treat. Radiation and surgery represent the major curative modalities for local tumors. Based on the positive experimental findings by combining radiation and ICBs, it seems a logical step to test the combination in the clinic. ICBs alone have been most widely used in melanoma patients which is also reflected in the first clinical results for combination of ICBs and radiation. It should be noted that most reports are retrospective studies where it is unclear why single drug therapy or radiation was selected, thus the selection to different therapy groups may be biased. The first clinical observation of response to combined treatment was noted in a metastatic melanoma patient who had progressed on ipilimumab, where successive radiation led to regression in both irradiated and non-irradiated sites [Citation76]. A study then documented prolonged survival from 5 to 21 months when radiation alone was compared with radiation and ipilimumab in 77 patients with brain metastases from malignant melanoma [Citation119]. As shown in several studies have reported similar findings, but two studies could not document any difference. When the anti-PD-L1 antibodies pembrolizumab or nivolumab (group 1) were compared with the anti-CTLA-4 antibody ipilimumab (group 2), a combination of a BRAF inhibitor and a MEK inhibitor (group 3), BRAF inhibitor alone (group 4) or chemotherapy (group 5) in patients treated with single session stereotactic brain radiation for brain metastases from malignant melanoma, the distant brain control rate at 12 months was 38%, 21%, 20%, 8% and 5%, respectively [Citation120]. The first three groups also exhibited improved overall survival. Currently, many clinical trials combining ICBs and radiation are ongoing [Citation121–123].
Table 2. Clinical reports on combination of radiation and immune checkpoint blockers.
Seventy-five clinical trials where radiation is combined with ICBs are registered at ClinicalTrial.gov (). The main focus is on lung cancer, head and neck cancers and melanomas, but many cancer types are included in the trials. Most studies seem to be a continuation of the sporadic reported clinical combined treatments presented in .
Future prospects
The progress of immunotherapy the last 5 years represents a breakthrough in medical oncology with response rates of ⁓10–40%, and even 50–60% by combination of different ICBs, and durable responses for more than 2 years in selected advanced malignancies [Citation124]. Combination of CTLA-4 and PD-1/PDL-1 or PDL-2 inhibitors seems to be particularly effective, reflecting that these drugs have different target sites, CTLA-4 affects activation of CD8 T-cells while PD-1 on activated CD8 T-cells interact with PD-L1 or PD-L2 and therefore reduce the effector function [Citation125]. However, the combination of different ICBs may increase the toxicity. Long-term tumor control can now be obtained in metastatic cancers where we previously had no curative treatment options. The other side of the story is the fact that most tumors cannot be controlled by these drugs due to inherent or acquired resistance. It is important that CD8 T-cells have systemic anticancer effects, attacking cancer cells in any site in the body. Experience indicates that most anticancer drugs, as well as immunotherapy, are most effective when the cancer cell burden is limited.
Radiation therapy remains a main curative and palliative modality to treat cancer together with surgery or concomitant chemotherapy/targeted therapy. A limitation to the curative potential of radiation for solid malignancies is that the modality can only be used with curative intent for local disease or regional (and in some cases of oligometastatic) spread due to normal tissue tolerance. The optimal dose and fractionation to expose tumor antigens are not yet finally settled. It seems necessary to induce sufficient damage in the tumor cells to expose danger signals or molecules (calreticulin, Hsps, HMGB1, ATP etc.) which can attract dendritic cells and CD8 T-cells. Experimental data indicate that moderately hypo-fractionated regimens or high doses release more immunogenic stimulatory factors, thus favoring development of mature dendritic cells, and activate CD8 T-cells [Citation126–128]. Radiation alone can induce abscopal responses, but these seem rare [Citation129,Citation130].
The clinical results presented in show evidence of a positive interaction of radiation and ICBs, but limitations are the retrospective design of most studies and an effect of ICBs alone is difficult to disclose. It is too early to claim a clinical paradigm shift for the combination of ICBs and radiation, but the combination should be seriously addressed. To prove the positive interaction of these two modalities it could be logic to add radiation to patients who do not respond to ICBs or to those who develop resistance [Citation76,Citation131]. Examples of this strategy have been seen in malignant melanoma and lung cancer. Another possibility that might seem attractive in tumor types where the patients tend to develop metastases despite good local tumor control, is to use optimal radiation for best possible local tumor control and simultaneously expose specific tumor antigens for the immune system to successively attack micrometastases. It is presumed that systemic immunotherapy may more efficiently eradicate micrometastases than bulky disease [Citation129]. Locally advanced anal cancer is an example where this strategy may be used. In a series of 41 anal cancer patients, 56% were positive for PD-L1 in archived paraffin blocks from primary tumors [Citation132]. Tumour-infiltrating CD8 T-cells are related to HPV status and infiltration of CD8 T-cells is a good prognostic factor in anal cancer [Citation133,Citation134]. The abscopal response has also been documented after radiation of anal cancer [Citation135]. Recently nivolumab (3 mg/kg) every second week was administered to 37 patients with recurrent anal squamous carcinomas of the anal canal in a phase II study [Citation136]. Two complete responses and 7 partial responses (24% response rate) were reported in this HPV related disease. As a next step, the authors plan to combine nivolumab and ipilimumab. We expect that by selecting high-risk patients without systemic manifested metastases, a better chance for cures can be obtained by this combination of ICB and radiation as initial therapy. Local and systemic failure after radiation with chemotherapy for anal cancer with lymph node metastases without systemic disease is in our experience 30–40% despite an initial complete tumor response [Citation137,Citation138]. Locally advanced anal cancer seems therefore to be a candidate for a randomized study to test whether combination of radiation therapy with immunotherapy will increase tumor control above that of radiation alone.
An abscopal response was documented after use of carbon-ion radiation in recurrent colorectal cancer [Citation139]. Carbon ion radiation seems to expose more tumor antigens for stimulation of CD8 T-cells and can yield an abscopal response. Pancreas cancer has a grim prognosis despite often limited metastases at presentation. Carbon ion radiation suppresses migration and invasiveness of human pancreatic carcinoma cells in contrast to photon radiation in experimental studies [Citation140]. Carbon-ion radiotherapy combined with surgery is used in clinical trials [Citation141]. When carbon-ion therapy was combined with gemcitabine it yielded 83% local control at 2 years, while the patients died of metastases resulting in only 35% 2-year survival [Citation142]. Selecting carbon ion radiation which can control local tumor growth and simultaneously expose tumor antigens which can be attacked by CD8 T-cells released by ICBs, may, therefore, be a new strategy for pancreatic cancer.
Based on the available evidence presented above ICBs aimed at CTLA-4 and the PD-1/PD-L1 axis are actively combined with radiation, but inhibitors of PD-1/PD-L1 yields higher effects. There seem to be more adverse effects associated with CTLA-4 inhibitors, possibly related to the fact that CTLA-4 is also expressed on regulatory T-cells which normally suppress autoimmunity. A combination of both seems to yield the best results with acceptable tolerance despite being hampered with more side effects.
Conclusions
Immune checkpoint inhibition has documented effect in a selected group of cancers, and combination of ICBs with different mechanisms of action is part of our standard armamentarium to control malignant diseases. Experimental studies show that radiation may expose tumor antigens and danger signals which attract immune cells. Both experimental studies and early clinical reports indicate that a combination of radiation with ICBs improves local control of cancer and may influence growth of tumor cells outside the irradiated areas. Hopefully, the high cost of such combined modality therapy will not be a barrier for further controlled clinical studies.
Disclosure statement
No potential conflict of interest was reported by the authors. Supported by Bergen Medical Foundation (Jon Espen Dale research fellow grant).
References
- Burstein HJ, Krilov L, Aragon-Ching JB, et al. Clinical cancer advances 2017: annual report on progress against cancer from the American Society of Clinical Oncology. J Clin Oncol. 2017;35:1341–1367.
- Larsen IK, Møller B, Johannesen TB, et al. Cancer in Norway 2017. Cancer Incidence, Mortality, Survival Preval Norway. 2018;1–103.
- Borras JM, Lievens Y, Barton M, et al. How many new cancer patients in Europe will require radiotherapy by 2025? An ESTRO-HERO analysis. Radiother Oncol. 2016;119:5–11.
- Baumann M, Krause M, Overgaard J, et al. Radiation oncology in the era of precision medicine. Nat Rev Cancer. 2016;16:234–249.
- Hoyer M, Muren LP, Glimelius B. The evolution of radiotherapy techniques in the management of prostate cancer. Acta Oncol. 2015;54:821–824.
- Combs SE, Debus J. Treatment with heavy charged particles: systematic review of clinical data and current clinical (comparative) trials. Acta Oncol. 2013;52:1272–1286.
- Jakobi A, Stutzer K, Bandurska-Luque A, et al. NTCP reduction for advanced head and neck cancer patients using proton therapy for complete or sequential boost treatment versus photon therapy. Acta Oncol. 2015;54:1658–1664.
- Kamada T, Tsujii H, Blakely EA, et al. Carbon ion radiotherapy in Japan: an assessment of 20 years of clinical experience. Lancet Oncol. 2015;16:e93–e100.
- Dahl O, Brydøy M. The pioneers behind immune checkpoint blockers awarded the Nobel Prize in physiology or medicine 2018. Acta Oncologica. 2019;58:
- Puck TT, Morkovin D, Marcus PI, et al. Action of x-rays on mammalian cells. II. Survival curves of cells from normal human tissues. J Exp Med. 1957;106:485–500.
- Iliakis G, Wang Y, Guan J, et al. DNA damage checkpoint control in cells exposed to ionizing radiation. Oncogene. 2003;22:5834–5847.
- Mikkelsen RB, Wardman P. Biological chemistry of reactive oxygen and nitrogen and radiation-induced signal transduction mechanisms. Oncogene. 2003;22:5734–5754.
- Ward JF. DNA damage as the cause of ionizing radiation-induced gene activation. Radiat Res. 1994;138:S85–S88.
- Helleday T, Petermann E, Lundin C, et al. DNA repair pathways as targets for cancer therapy. Nat Rev Cancer. 2008;8:193–204.
- Kolesnick R, Fuks Z. Radiation and ceramide-induced apoptosis. Oncogene. 2003;22:5897–5906.
- Fei P, El-Deiry WS. P53 and radiation responses. Oncogene. 2003;22:5774–5783.
- Balcer-Kubiczek EK. Apoptosis in radiation therapy: a double-edged sword. Exp Oncol. 2012;34:277–285.
- Kerr JF, Wyllie AH, Currie AR. Apoptosis: a basic biological phenomenon with wide-ranging implications in tissue kinetics. Br J Cancer. 1972;26:239–257.
- Amaravadi R, Kimmelman AC, White E. Recent insights into the function of autophagy in cancer. Genes Dev. 2016;30:1913–1930.
- White E, Mehnert JM, Chan CS. Autophagy, metabolism, and cancer. Clin Cancer Res. 2015;21:5037–5046.
- Firat E, Gaedicke S, Tsurumi C, et al. Delayed cell death associated with mitotic catastrophe in γ-irradiated stem-like glioma cells. Radiat Oncol. 2011;6:71.
- Mc Gee MM. Targeting the mitotic catastrophe signaling pathway in cancer. Mediators Inflamm. 2015;2015:146282.
- Vakifahmetoglu H, Olsson M, Zhivotovsky B. Death through a tragedy: mitotic catastrophe. Cell Death Differ. 2008;15:1153–1162.
- Campisi J, d'Adda di Fagagna F. Cellular senescence: when bad things happen to good cells. Nat Rev Mol Cell Biol. 2007;8:729–740.
- Gewirtz DA, Alotaibi M, Yakovlev VA, et al. Tumor cell recovery from senescence induced by radiation with PARP inhibition. Radiat Res. 2016;186:327–332.
- Wunderlich R, Ruehle PF, Deloch L, et al. Interconnection between DNA damage, senescence, inflammation, and cancer. Front Biosci (Landmark Ed). 2017;22:348–369.
- Matt S, Hofmann TG. The DNA damage-induced cell death response: a roadmap to kill cancer cells. Cell Mol Life Sci. 2016;73:2829–2850.
- Rodemann HP, Blaese MA. Responses of normal cells to ionizing radiation. Semin Radiat Oncol. 2007;17:81–88.
- Roos WP, Thomas AD, Kaina B. DNA damage and the balance between survival and death in cancer biology. Nat Rev Cancer. 2016;16:20–33.
- Kurtova AV, Xiao J, Mo Q, et al. Blocking PGE2-induced tumour repopulation abrogates bladder cancer chemoresistance. Nature. 2015;517:209–213.
- Tannock IF. Cancer: resistance through repopulation. Nature. 2015;517:152–153.
- Ondrej M, Cechakova L, Durisova K, et al. To live or let die: unclear task of autophagy in the radiosensitization battle. Radiother Oncol. 2016;119:265–275.
- Kim YH, Yoo KC, Cui YH, et al. Radiation promotes malignant progression of glioma cells through HIF-1alpha stabilization. Cancer Lett. 2014;354:132–141.
- Cordes N, Rodel F, Rodemann HP. Molecular signaling pathways. mechanisms and clinical use. Strahlenther Onkol. 2012;188 Suppl 3:308–311.
- Kachikwu EL, Iwamoto KS, Liao YP, et al. Radiation enhances regulatory T cell representation. Int J Radiat Oncol Biol Phys. 2011;81:1128–1135.
- Order SE. The effects of therapeutic irradiation on lymphocytes and immunity. Cancer. 1977;39:737–743.
- Wara WM, Phillips TL, Wara DW, et al. Immunosuppression following radiation therapy for carcinoma of the nasopharynx. Am J Roentgenol Radium Ther Nucl Med. 1975;123:482–485.
- Anscher MS. Targeting the TGF-beta1 pathway to prevent normal tissue injury after cancer therapy. Oncologist. 2010;15:350–359.
- Derer A, Frey B, Fietkau R, et al. Immune-modulating properties of ionizing radiation: rationale for the treatment of cancer by combination radiotherapy and immune checkpoint inhibitors. Cancer Immunol Immunother. 2016;65:779–786.
- Desai S, Kumar A, Laskar S, et al. Cytokine profile of conditioned medium from human tumor cell lines after acute and fractionated doses of gamma radiation and its effect on survival of bystander tumor cells. Cytokine. 2013;61:54–62.
- Marabelle A, Filatenkov A, Sagiv-Barfi I, et al. Radiotherapy and toll-like receptor agonists. Semin Radiat Oncol. 2015;25:34–39.
- Roses RE, Xu M, Koski GK, et al. Radiation therapy and toll-like receptor signaling: implications for the treatment of cancer. Oncogene. 2008;27:200–207.
- Barsoum IB, Smallwood CA, Siemens DR, et al. A mechanism of hypoxia-mediated escape from adaptive immunity in cancer cells. Cancer Res. 2014;74:665–674.
- Jeong H, Bok S, Hong BJ, et al. Radiation-induced immune responses: mechanisms and therapeutic perspectives. Blood Res. 2016;51:157–163.
- Wennerberg E, Lhuillier C, Vanpouille-Box C, et al. Barriers to radiation-induced in situ tumor vaccination. Front Immunol. 2017;8:229.
- McBride WH, Chiang CS, Olson JL, et al. A sense of danger from radiation. Radiat Res. 2004;162:1–19.
- Fuchs EJ, Matzinger P. Is cancer dangerous to the immune system? Semin Immunol. 1996;8:271–280.
- Garg AD, Galluzzi L, Apetoh L, et al. Molecular and translational classifications of DAMPs in immunogenic cell death. Front Immunol. 2015;6:588.
- Pradeu T, Cooper EL. The danger theory: 20 years later. Front Immunol. 2012;3:287.
- Eisenbacher JL, Schrezenmeier H, Jahrsdorfer B, et al. S100A4 and uric acid promote mesenchymal stromal cell induction of IL-10+/IDO + lymphocytes. J Immunol. 2014;192:6102–6110.
- Land WG, Agostinis P, Gasser S, et al. Transplantation and damage-associated molecular patterns (DAMPs). Am J Transplant. 2016;16:3338–3361.
- Sharabi AB, Lim M, DeWeese TL, et al. Radiation and checkpoint blockade immunotherapy: radiosensitisation and potential mechanisms of synergy. Lancet Oncol. 2015;16:e498–e509.
- Lotfi R, Kaltenmeier C, Lotze MT, et al. Until death do us part: necrosis and oxidation promote the tumor microenvironment. Transfus Med Hemother. 2016;43:120–132.
- Ohta A. A metabolic immune checkpoint: adenosine in tumor microenvironment. Front Immunol. 2016;7:e109.
- Vega VL, Rodriguez-Silva M, Frey T, et al. Hsp70 translocates into the plasma membrane after stress and is released into the extracellular environment in a membrane-associated form that activates macrophages. J Immunol. 2008;180:4299–4307.
- Li K, Qu S, Chen X, et al. Promising targets for cancer immunotherapy: TLRs, RLRs, and STING-mediated innate immune pathways. Int J Mol Sci. 2017;18:E404.
- Vanpouille-Box C, Alard A, Aryankalayil MJ, et al. DNA exonuclease Trex1 regulates radiotherapy-induced tumour immunogenicity. Nat Comms. 2017;8:15618.
- Liang H, Deng L, Hou Y, et al. Host STING-dependent MDSC mobilization drives extrinsic radiation resistance. Nat Commun. 2017;8:1736.
- Filatenkov A, Baker J, Mueller AM, et al. Ablative tumor radiation can change the tumor immune cell microenvironment to induce durable complete remissions. Clin Cancer Res. 2015;21:3727–3739.
- Golden EB, Frances D, Pellicciotta I, et al. Radiation fosters dose-dependent and chemotherapy-induced immunogenic cell death. Oncoimmunology. 2014;3:e28518.
- Lugade AA, Moran JP, Gerber SA, et al. Local radiation therapy of B16 melanoma tumors increases the generation of tumor antigen-specific effector cells that traffic to the tumor. J Immunol. 2005;174:7516–7523.
- Muraro E, Furlan C, Avanzo M, et al. Local high-dose radiotherapy induces systemic immunomodulating effects of potential therapeutic relevance in oligometastatic breast cancer. Front Immunol. 2017;8:1476.
- DeGiorgi LS. Regression of pulmonary metastases during radiation to a hypernephroma. Immunity and cancer. Cancer. 1972;30:895–899.
- Formenti SC, Demaria S. Systemic effects of local radiotherapy. Lancet Oncol. 2009;10:718–726.
- Michot JM, Mazeron R, Dercle L, et al. Abscopal effect in a Hodgkin lymphoma patient treated by an anti-programmed death 1 antibody. Eur J Cancer. 2016;66:91–94.
- Reynders K, Illidge T, Siva S, et al. The abscopal effect of local radiotherapy: using immunotherapy to make a rare event clinically relevant. Cancer Treat Rev. 2015;41:503–510.
- Mole RH. Whole body irradiation; radiobiology or medicine? Br J Radiol. 1953;26:234–241.
- Formenti SC, Demaria S. Combining radiotherapy and cancer immunotherapy: a paradigm shift. J Natl Cancer Inst. 2013;105:256–265.
- Lock M, Muinuddin A, Kocha WI, et al. Abscopal effects: case report and emerging opportunities. Cureus. 2015;7:e344.
- Rubner Y, Wunderlich R, Ruhle PF, et al. How does ionizing irradiation contribute to the induction of anti-tumor immunity? Front Oncol. 2012;2:75.
- Demaria S, Ng B, Devitt ML, et al. Ionizing radiation inhibition of distant untreated tumors (abscopal effect) is immune mediated. Int J Radiat Oncol Biol Phys. 2004;58:862–870.
- Chakravarty PK, Alfieri A, Thomas EK, et al. Flt3-ligand administration after radiation therapy prolongs survival in a murine model of metastatic lung cancer. Cancer Res. 1999;59:6028–6032.
- Grass GD, Krishna N, Kim S. The immune mechanisms of abscopal effect in radiation therapy. Curr Probl Cancer. 2016;40:10–24.
- Chandra RA, Wilhite TJ, Balboni TA, et al. A systematic evaluation of abscopal responses following radiotherapy in patients with metastatic melanoma treated with ipilimumab. Oncoimmunology. 2015;4:e1046028.
- Grimaldi AM, Simeone E, Giannarelli D, et al. Abscopal effects of radiotherapy on advanced melanoma patients who progressed after ipilimumab immunotherapy. Oncoimmunology. 2014;3:e28780.
- Postow MA, Callahan MK, Barker CA, et al. Immunologic correlates of the abscopal effect in a patient with melanoma. N Engl J Med. 2012;366:925–931.
- Chicas-Sett R, Morales-Orue I, Rodriguez-Abreu D, et al. Combining radiotherapy and ipilimumab induces clinically relevant radiation-induced abscopal effects in metastatic melanoma patients: a systematic review. Clin Transl Radiat Oncol. 2018;9:5–11.
- Siva S, MacManus MP, Martin RF, et al. Abscopal effects of radiation therapy: a clinical review for the radiobiologist. Cancer Lett. 2015;356:82–90.
- Levy A, Chargari C, Marabelle A, et al. Can immunostimulatory agents enhance the abscopal effect of radiotherapy? Eur J Cancer. 2016;62:36–45.
- Ngwa W, Irabor OC, Schoenfeld JD, et al. Using immunotherapy to boost the abscopal effect. Nat Rev Cancer. 2018;18:313–322.
- Ishihara D, Pop L, Takeshima T, et al. Rationale and evidence to combine radiation therapy and immunotherapy for cancer treatment. Cancer Immunol Immunother. 2017;66:281–298.
- Klebanoff CA, Acquavella N, Yu Z, et al. Therapeutic cancer vaccines: are we there yet? Immunol Rev. 2011;239:27–44.
- Tang C, Wang X, Soh H, et al. Combining radiation and immunotherapy: a new systemic therapy for solid tumors? Cancer Immunol Res. 2014;2:831–838.
- Liao YP, Wang CC, Butterfield LH, et al. Ionizing radiation affects human MART-1 melanoma antigen processing and presentation by dendritic cells. J Immunol. 2004;173:2462–2469.
- Reits EA, Hodge JW, Herberts CA, et al. Radiation modulates the peptide repertoire, enhances MHC class I expression, and induces successful antitumor immunotherapy. J Exp Med. 2006;203:1259–1271.
- Zhang B, Bowerman NA, Salama JK, et al. Induced sensitization of tumor stroma leads to eradication of established cancer by T cells. J Exp Med. 2007;204:49–55.
- Frey B, Derer A, Scheithauer H, et al. Cancer cell death-inducing radiotherapy: Impact on local tumour control, tumour cell proliferation and induction of systemic anti-tumour immunity. Adv Exp Med Biol. 2016;930:151–172.
- Wattenberg MM, Fahim A, Ahmed MM, et al. Unlocking the combination: potentiation of radiation-induced antitumor responses with immunotherapy. Radiat Res. 2014;182:126–138.
- Demaria S, Bhardwaj N, McBride WH, et al. Combining radiotherapy and immunotherapy: a revived partnership. Int J Radiat Oncol Biol Phys. 2005;63:655–666.
- Twyman-Saint Victor C, Rech AJ, Maity A, et al. Radiation and dual checkpoint blockade activate non-redundant immune mechanisms in cancer. Nature. 2015;520:373–377.
- Bernstein MB, Garnett CT, Zhang H, et al. Radiation-induced modulation of costimulatory and coinhibitory T-cell signaling molecules on human prostate carcinoma cells promotes productive antitumor immune interactions. Cancer Biother Radiopharm. 2014;29:153–161.
- Kim JE, Patel MA, Mangraviti A, et al. Combination therapy with anti-PD-1, anti-TIM-3, and focal radiation results in regression of murine gliomas. Clin Cancer Res. 2017;23:124–136.
- Verbrugge I, Gasparini A, Haynes NM, et al. The curative outcome of radioimmunotherapy in a mouse breast cancer model relies on mTOR signaling. Radiat Res. 2014;182:219–229.
- Li X, Hu W, Zheng X, et al. Emerging immune checkpoints for cancer therapy. Acta Oncol. 2015;54:1706–1713.
- Sharma P, Allison JP. Immune checkpoint targeting in cancer therapy: toward combination strategies with curative potential. Cell. 2015;161:205–214.
- Elvington M, Scheiber M, Yang X, et al. Complement-dependent modulation of antitumor immunity following radiation therapy. Cell Rep. 2014;8:818–830.
- Pilones KA, Kawashima N, Yang AM, et al. Invariant natural killer T cells regulate breast cancer response to radiation and CTLA-4 blockade. Clin Cancer Res. 2009;15:597–606.
- Verbrugge I, Hagekyriakou J, Sharp LL, et al. Radiotherapy increases the permissiveness of established mammary tumors to rejection by immunomodulatory antibodies. Cancer Res. 2012;72:3163–3174.
- Belcaid Z, Phallen JA, Zeng J, et al. Focal radiation therapy combined with 4-1BB activation and CTLA-4 blockade yields long-term survival and a protective antigen-specific memory response in a murine glioma model. PLoS One. 2014;9:e101764.
- Demaria S, Kawashima N, Yang AM, et al. Immune-mediated inhibition of metastases after treatment with local radiation and CTLA-4 blockade in a mouse model of breast cancer. Clin Cancer Res. 2005;11:728–734.
- Park SS, Dong H, Liu X, et al. PD-1 restrains radiotherapy-induced abscopal effect. Cancer Immunol Res. 2015;3:610–619.
- Deng L, Liang H, Burnette B, et al. Irradiation and anti-PD-L1 treatment synergistically promote antitumor immunity in mice. J Clin Invest. 2014;124:687–695.
- Wu CT, Chen WC, Chang YH, et al. The role of PD-L1 in the radiation response and clinical outcome for bladder cancer. Sci Rep. 2016;6:19740.
- Zeng J, See AP, Phallen J, et al. Anti-PD-1 blockade and stereotactic radiation produce long-term survival in mice with intracranial gliomas. Int J Radiat Oncol Biol Phys. 2013;86:343–349.
- Dovedi SJ, Adlard AL, Lipowska-Bhalla G, et al. Acquired resistance to fractionated radiotherapy can be overcome by concurrent PD-L1 blockade. Cancer Res. 2014;74:5458–5468.
- Young KH, Baird JR, Savage T, et al. Optimizing timing of immunotherapy improves control of tumors by hypofractionated radiation therapy. PLoS One. 2016;11:e0157164.
- Shaverdian N, Lisberg AE, Bornazyan K, et al. Previous radiotherapy and the clinical activity and toxicity of pembrolizumab in the treatment of non-small-cell lung cancer: a secondary analysis of the KEYNOTE-001 phase 1 trial. Lancet Oncol. 2017;18:895–903.
- Antonia SJ, Villegas A, Daniel D, et al. Durvalumab after chemoradiotherapy in stage III non-small-cell lung cancer. N Engl J Med. 2017;377:1919–1929.
- Antonia SJ, Villegas A, Daniel D, et al. Overall survival with durvalumab after chemoradiotherapy in stage III NSCLC. N Engl J Med 2018;Sept 25. Epub.
- Koller KM, Mackley HB, Liu J, et al. Improved survival and complete response rates in patients with advanced melanoma treated with concurrent ipilimumab and radiotherapy versus ipilimumab alone. Cancer Biol Ther. 2017;18:36–42.
- Gameiro SR, Malamas AS, Bernstein MB, et al. Tumor cells surviving exposure to proton or photon radiation share a common immunogenic modulation signature, rendering them more sensitive to T cell-mediated killing. Int J Radiat Oncol Biol Phys. 2016;95:120–130.
- Ohkubo Y, Iwakawa M, Seino K, et al. Combining carbon ion radiotherapy and local injection of alpha-galactosylceramide-pulsed dendritic cells inhibits lung metastases in an in vivo murine model. Int J Radiat Oncol Biol Phys. 2010;78:1524–1531.
- Ogata T, Teshima T, Kagawa K, et al. Particle irradiation suppresses metastatic potential of cancer cells. Cancer Res. 2005;65:113–120.
- Matsunaga A, Ueda Y, Yamada S, et al. Carbon-ion beam treatment induces systemic antitumor immunity against murine squamous cell carcinoma. Cancer. 2010;116:3740–3748.
- Shimokawa T, Ma L, Ando K, et al. The future of combining carbon-ion radiotherapy with immunotherapy: evidence and progress in mouse models. Int J Particle Ther 2016. 2016;3:61–70.
- Datta NR, Ordonez SG, Gaipl US, et al. Local hyperthermia combined with radiotherapy and-/or chemotherapy: recent advances and promises for the future. Cancer Treat Rev. 2015;41:742–753.
- Issels R, Kampmann E, Kanaar R, et al. Hallmarks of hyperthermia in driving the future of clinical hyperthermia as targeted therapy: translation into clinical application. Int J Hyperthermia. 2016;32:89–95.
- Werthmoller N, Frey B, Ruckert M, et al. Combination of ionising radiation with hyperthermia increases the immunogenic potential of B16-F10 melanoma cells in vitro and in vivo. Int J Hyperthermia. 2016;32:23–30.
- Knisely JP, Yu JB, Flanigan J, et al. Radiosurgery for melanoma brain metastases in the ipilimumab era and the possibility of longer survival. J Neurosurg. 2012;117:227–233.
- Ahmed KA, Abuodeh YA, Echevarria MI, et al. Clinical outcomes of melanoma brain metastases treated with stereotactic radiosurgery and anti-PD-1 therapy, anti-CTLA-4 therapy, BRAF/MEK inhibitors, BRAF inhibitor, or conventional chemotherapy. Ann Oncol. 2016;27:2288–2294.
- Bernstein MB, Krishnan S, Hodge JW, et al. Immunotherapy and stereotactic ablative radiotherapy (ISABR): a curative approach? Nat Rev Clin Oncol. 2016;13:516–524.
- Kalbasi A, June CH, Haas N, et al. Radiation and immunotherapy: a synergistic combination. J Clin Invest. 2013;123:2756–2763.
- Kang J, Demaria S, Formenti S. Current clinical trials testing the combination of immunotherapy with radiotherapy. J Immunother Cancer. 2016;4:51.
- Smyth MJ, Ngiow SF, Ribas A, et al. Combination cancer immunotherapies tailored to the tumour microenvironment. Nat Rev Clin Oncol. 2016;13:143–158.
- Boussiotis VA. Molecular and biochemical aspects of the PD-1 checkpoint pathway. N Engl J Med. 2016;375:1767–1778.
- Deloch L, Derer A, Hartmann J, et al. Modern radiotherapy concepts and the impact of radiation on immune activation. Front Oncol. 2016;6:141.
- Frey B, Ruckert M, Weber J, et al. Hypofractionated irradiation has immune stimulatory potential and induces a timely restricted infiltration of immune cells in colon cancer tumors. Front Immunol. 2017;8:231.
- Kulzer L, Rubner Y, Deloch L, et al. Norm- and hypo-fractionated radiotherapy is capable of activating human dendritic cells. J Immunotoxicol. 2014;11:328–336.
- Brooks ED, Schoenhals JE, Tang C, et al. Stereotactic ablative radiation therapy combined with immunotherapy for solid tumors. Cancer J. 2016;22:257–266.
- Popp I, Grosu AL, Niedermann G, et al. Immune modulation by hypofractionated stereotactic radiation therapy: therapeutic implications. Radiother Oncol. 2016;120:185–194.
- Schoenhals JE, Seyedin SN, Tang C, et al. Preclinical rationale and clinical considerations for radiotherapy plus immunotherapy: going beyond local control. Cancer J. 2016;22:130–137.
- Govindarajan R, Gujja S, Siegel ER, et al. Programmed cell death-ligand 1 (PD-L1) expression in anal cancer. Am J Clin Oncol. 2016;41:638–642.
- Balermpas P, Martin D, Wieland U, et al. Human papilloma virus load and PD-1/PD-L1, CD8(+) and FOXP3 in anal cancer patients treated with chemoradiotherapy: rationale for immunotherapy. Oncoimmunology. 2017;6:e1288331.
- Martin D, Rodel F, Balermpas P, et al. The immune microenvironment and HPV in anal cancer: Rationale to complement chemoradiation with immunotherapy. Biochim Biophys Acta Rev Cancer. 2017;1868:221–230.
- Joe MB, Lum JJ, Watson PH, et al. Radiation generates an abscopal response and complete resolution of metastatic squamous cell carcinoma of the anal canal: a case report. J Gastrointest Oncol. 2017;8:E84–E89.
- Morris VK, Salem ME, Nimeiri H, et al. Nivolumab for previously treated unresectable metastatic anal cancer (NCI9673): a multicentre, single-arm, phase 2 study. Lancet Oncol. 2017;18:446–453.
- Dale JE, Sebjornsen S, Leh S, et al. Multimodal therapy is feasible in elderly anal cancer patients. Acta Oncol. 2017;56:81–87.
- Leon O, Guren M, Hagberg O, et al. Anal carcinoma - survival and recurrence in a large cohort of patients treated according to Nordic guidelines. Radiother Oncol. 2014;113:352–358.
- Ebner DK, Kamada T, Yamada S. Abscopal effect in recurrent colorectal cancer treated with carbon-ion radiation therapy: 2 case reports. Adv Radiat Oncol. 2017;2:333–338.
- Fujita M, Imadome K, Shoji Y, et al. Carbon-ion irradiation suppresses migration and invasiveness of human pancreatic carcinoma cells MIAPaCa-2 via Rac1 and RhoA degradation. Int J Radiat Oncol Biol Phys. 2015;93:173–180.
- Cobianchi L, Fossati P, Peloso A, et al. Carbon ion radiotherapy and completion pancreatectomy. A feasible model to explore a new integrated approach? Pancreatology. 2017;17:19–21.
- Shinoto M, Yamada S, Terashima K, et al. Carbon ion radiation therapy with concurrent gemcitabine for patients with locally advanced pancreatic cancer. Int J Radiat Oncol Biol Phys. 2016;95:498–504.
- Dewan MZ, Galloway AE, Kawashima N, et al. Fractionated but not single-dose radiotherapy induces an immune-mediated abscopal effect when combined with anti-CTLA-4 antibody. Clin Cancer Res. 2009;15:5379–5388.
- Yoshimoto Y, Suzuki Y, Mimura K, et al. Radiotherapy-induced anti-tumor immunity contributes to the therapeutic efficacy of irradiation and can be augmented by CTLA-4 blockade in a mouse model. PLoS One. 2014;9:e92572.
- Azad A, Yin Lim S, D'Costa Z, et al. PD-L1 blockade enhances response of pancreatic ductal adenocarcinoma to radiotherapy. EMBO Mol Med. 2017;9:167–180.
- Stamell EF, Wolchok JD, Gnjatic S, et al. The abscopal effect associated with a systemic anti-melanoma immune response. Int J Radiat Oncol Biol Phys. 2013;85:293–295.
- Golden EB, Demaria S, Schiff PB, et al. An abscopal response to radiation and ipilimumab in a patient with metastatic non-small cell lung cancer. Cancer Immunol Res. 2013;1:365–372.
- Slovin SF, Higano CS, Hamid O, et al. Ipilimumab alone or in combination with radiotherapy in metastatic castration-resistant prostate cancer: results from an open-label, multicenter phase I/II study. Ann Oncol. 2013;24:1813–1821.
- Mathew M, Tam M, Ott PA, et al. Ipilimumab in melanoma with limited brain metastases treated with stereotactic radiosurgery. Melanoma Res. 2013;23:191–195.
- Barker CA, Postow MA, Khan SA, et al. Concurrent radiotherapy and ipilimumab immunotherapy for patients with melanoma. Cancer Immunol Res. 2013;1:92–98.
- Silk AW, Bassetti MF, West BT, et al. Ipilimumab and radiation therapy for melanoma brain metastases. Cancer Med. 2013;2:899–906.
- Kwon ED, Drake CG, Scher HI, et al. Ipilimumab versus placebo after radiotherapy in patients with metastatic castration-resistant prostate cancer that had progressed after docetaxel chemotherapy (CA184-043): a multicentre, randomised, double-blind, phase 3 trial. Lancet Oncol. 2014;15:700–712.
- Kiess AP, Wolchok JD, Barker CA, et al. Stereotactic radiosurgery for melanoma brain metastases in patients receiving ipilimumab: safety profile and efficacy of combined treatment. Int J Radiat Oncol Biol Phys. 2015;92:368–375.
- Patel KR, Shoukat S, Oliver DE, et al. Ipilimumab and stereotactic radiosurgery versus stereotactic radiosurgery alone for newly diagnosed melanoma brain metastases. Am J Clin Oncol 2015;40:444–450.
- Qin R, Olson A, Singh B, et al. Safety and efficacy of radiation therapy in advanced melanoma patients treated with ipilimumab. Int J Radiat Oncol Biol Phys. 2016;96:72–77.
- Hiniker SM, Reddy SA, Maecker HT, et al. A prospective clinical trial combining radiation therapy with systemic immunotherapy in metastatic melanoma. Int J Radiat Oncol Biol Phys. 2016;96:578–588.
- Kropp LM, De Los Santos JF, McKee SB, et al. Radiotherapy to control limited melanoma progression following ipilimumab. J Immunother. 2016;39:373–378.
- Levy A, Massard C, Soria JC, et al. Concurrent irradiation with the anti-programmed cell death ligand-1 immune checkpoint blocker durvalumab: single centre subset analysis from a phase 1/2 trial. Eur J Cancer. 2016;68:156–162.
- Ahmed KA, Kim S, Arrington J, et al. Outcomes targeting the PD-1/PD-L1 axis in conjunction with stereotactic radiation for patients with non-small cell lung cancer brain metastases. J Neurooncol. 2017;133:331–338.
- Williams NL, Wuthrick EJ, Kim H, et al. Phase 1 study of ipilimumab combined with whole brain radiation therapy or radiosurgery for melanoma patients with brain metastases. Int J Radiat Oncol Biol Phys. 2017;99:22–30.
- Pike LRG, Bang A, Ott P, et al. Radiation and PD-1 inhibition: favorable outcomes after brain-directed radiation. Radiother Oncol. 2017;124:98–103.