Abstract
Background
Exercise and physical activity (PA) are associated with reduced tumor growth and enhanced intra-tumoral immune cell infiltration in mice. We aimed to investigate the role of PA achieved by voluntary wheel running in promoting the immunogenic profile across several murine tumor models, and to explore the potential of checkpoint blockade and PA in the form of voluntary wheel running as combination therapy.
Material and methods
The experiments were performed with C57BL/6 mice bearing subcutaneous tumors while having access to running wheels in their cages, where key immunoregulatory molecules expressed in the tumor tissue were measured by qPCR. Furthermore, we tested the hypothesis that wheel running combined with PD-L1 -or PD-1 inhibitor treatment could lead to an additive effect on tumor growth in mice bearing B16 melanoma tumors.
Results
Wheel running increased immune checkpoint expression (PD-1, PD-L1, PD-L2, CD28, B7.1 and B7.2) in B16 tumor-bearing mice, while induction of only PD-L2 was found in E0771 breast cancer and Lewis Lung Cancer. In studies combining voluntary wheel running with PD-1 -and PD-L1 inhibitors we found significant effects of wheel running on attenuating B16 melanoma tumor growth, in line with previous studies. We did, however, not find an additive effect of combining either of the two immunotherapeutic treatments with access to running wheels.
Conclusion
B16 tumors displayed upregulated expression of immune regulatory molecules and decreased tumor growth in response to PA. However, combining PA with PD-1 or PD-L1 blockade did not lead to a further augmented inhibition of tumor growth.
Introduction
Collective evidence from more than 670 unique exercise intervention studies in cancer patients highlights the safety and feasibility of exercise training across the entire cancer trajectory, with the interventions leading to improved physical function and health-related quality of life [Citation1]. Furthermore, accumulating evidence from preclinical studies indicates that exercise may control disease progression, reduce chemotherapy-related toxicities, and improve anti-cancer treatment efficacy [Citation2,Citation3].
We previously showed that voluntary wheel running could reduce tumor growth by more than 50% in both transplanted and toxin-induced tumor models [Citation4]. This PA-dependent control of tumor growth was associated with a high intra-tumoral infiltration of cytotoxic NK- and T cells in running mice, and the anti-neoplastic effect of running was completely abolished if the mice were depleted of NK cells, establishing a mechanistic link between exercise-dependent mobilization of cytotoxic immune cells and tumor growth control [Citation4]. This study sparked interest in combining voluntary wheel running and immunotherapy.
Immune checkpoint therapy has led to important clinical advances as a new treatment modality, which can elicit durable clinical responses in cancer patients [Citation5,Citation6]. Long-term remission achieved with this type of therapy appears to be associated with high degree of cytotoxic immune cell infiltration and immune checkpoint expression [Citation7,Citation8]. For instance, blockade of the Programmed Death protein 1 (PD-1)-axis induces robust clinical responses in cancer patients with high intra-tumoral immune cell infiltration and high expression level of Programmed Death Ligand 1 (PD-L1) [Citation9–12]. In contrast, non-immunogenic tumors without infiltration of cytotoxic immune cells and low PD-L1 expression are less sensitive to immune checkpoint inhibition [Citation13].
While the molecular mechanisms underlying the protective effect of exercise and PA on cancer development continue to be illuminated [Citation14], investigations into combining exercise or PA and immunotherapeutic treatment regimens have only recently emerged [Citation15].
High intra-tumoral expression of PD-L1 is generally associated with greater chances of responding to blockade of the PD-1/PD-L1 axis, and this can also be considered as an indication of an active immune response [Citation16]. Therefore, interventions to enhance checkpoint expression in already immunogenic tumors or induction of these in immunogenically ‘cold’ tumors are warranted in anti-cancer treatment with checkpoint blockade therapy [Citation5].
We test the hypothesis that voluntary wheel running may provide such physiological stimuli in mice, leading to increased checkpoint molecule expression, with the potential of achieving an additive effect of combining voluntary wheel running with checkpoint blockade therapy.
Material and methods
Animal studies
All animal experiments were conducted in compliance with the ARRIVE guidelines and after approval of the experimental protocol by the Danish Animal Experiments Inspectorate (2015-15-0201-00656). The mice were either bred in house with breeding pairs being from Taconic Bioscience, Denmark, or obtained and used directly from the company. Adult (8–16 weeks old) female mice (C57Bl/6NTac or NMRI-Foxn1nu) were housed in standard housing cages in a temperature and humidity-controlled room and maintained on a 12:12-h light-dark cycle with food and water ad libitum. For exercise interventions, running wheels (Starr Life Science, diameter 12 cm) were placed in the home cages as a model of voluntary exercise, and running distance was calculated by converting wheel rotations to kilometers divided by 2, as the mice were housed in pairs to avoid isolation-induced stress. Mice, which did not run more than 0.5 km per day one week prior to tumor inoculation, were excluded from the exercising groups. In all exercise experiments, access to running wheels was given 5 weeks prior to tumor cell inoculation.
Tumor models
We used several tumor models: murine B16F10 melanoma cells, murine E0771 breast cancer cells, and murine Lewis Lung Cancer (LLC), obtained from and authentified by ATCC®, Manassas, VA, USA. The human head and neck squamous cell cancer (HNSCC) line UTSCC45 was kindly provided by Reidar Grenman Department of Otorhinolaryngology Head and Neck Surgery, The Turku University and Turku University Hospital Finland.
In preparation of tumor inoculations, B16F10, LLC and UTSCC45 were grown in Dulbecco’s Modified Eagle Medium (DMEM) (1X), Glutamax™ (ThermoFisher Scientific (Gibco), Waltham, MA, USA), while E0771 cells were grown in RPMI 1640 culture medium (ThermoFisher Scientific). All cells were grown in 10 cm dishes (ThermoFisher Scientific) at 37 °C and 5% C02, and all media were supplemented with 10% fetal bovine serum (FBS) (ThermoFisher Scientific) and 1% Penicillin/Streptomycin (P/S) (ThermoFischer Scientific). For tumor induction, B16F10 (2 × 105 cells in 100 µl PBS/mouse) or LLC (2.5 × 105 cells in 100 µl PBS/mouse) were inoculated subcutaneously at the flank of C57Bl/6NTac, and UTSCC45 (5 × 106 cells in 100 µl PBS/mouse) were inoculated subcutaneously at the flank of immunodeficient NMRI-Foxn1nu. E0771 cells were inoculated in the mammary fat pad (1 × 105 cells in 100 µl PBS/mouse) in C57Bl/6. For data in , the response to exercise training on tumor growth and immune cell infiltration has previously been reported [Citation4].
Figure 1. Exercise impacts intra-tumoral expression of immune regulatory molecules. mRNA expression of immune regulatory molecules in control mice (gray circle) and running mice (orange dot) compared as fold change of ΔΔCt values relative to the control group. (A) B16 melanoma expression of regulatory molecules along with the correlation of Ifnγ expression to Pd-1 (B) and Pd-l1 (C) expression. (D) Immune regulatory molecules in E0771 breast cancer and (E) LLC lung cancer. (F) For UTSCC45 head & neck cancer, ΔΔCt values are shown with gene expressions normalized to the overall ΔCt for mouse Pd-l1 and human PD-L1. All mRNA expressions were normalized to housekeeping genes 18s or Ppia. Statistical significance was tested by multiple t-testing or Mann–Whitney and linear regression analyses with Pearson’s correlation test. Data are depicted as individual datapoints with indication of means. *p < 0.05, **p < 0.01, ***p < 0.001, ****p < 0.0001.
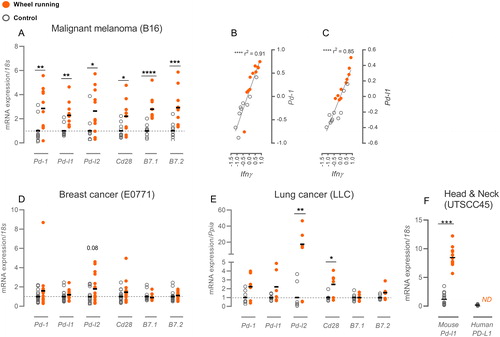
αPD-L1 treatment during voluntary wheel running intervention
Mice were randomly assigned to four groups (n = 14): control sedentary (PBS), control exercising (EX), αPD-L1 treated sedentary (αPD-L1) and αPD-L1 treated exercising (αPD-L1 + EX). After the acclimatization period, B16 melanoma tumors were inoculated, and 4 days later the treatments were initiated. The mice were injected i.p. 3 times per week with 100 µg α-mouse PD-L1 (clone 10 F.9G2 from BioXCell®, Lebanon, NH, USA) antibody in PBS or PBS alone in a volume of 100 µl for a duration of 2 weeks. At termination, tumors were excised, and their size determined by weight and by volume using the calculation V = d1×d2×d3×π/6, where d is the diameter of the tumor (measured with a caliper). Spleens were also excised and their size assed by weight. Body weight was measured at time of set-up, at tumor inoculation and at take-down. Six mice had to be sacrificed before take-down due to development of wounds at the tumor site. These mice are not included in the results shown here. The mice were evenly distributed throughout all groups.
αPD-1 treatment during voluntary wheel running intervention
The set-up was identical to the αPD-L1 study, with 4 groups (n = 14): control sedentary (PBS), control exercising (EX), αPD-1 treated sedentary (αPD-1) and αPD-1 treated exercising (αPD-1 + EX). Here, the mice were injected i.p. 2 times per week with 200 µg α-mouse PD-1 (clone RPM1-14 from BioXCell®) antibody in PBS or PBS alone in a volume of 100 µl for a duration of 2 weeks. As this experiment was terminated on day 13 due to large tumor sizes, the mice received only 3 injection and not 4 as initially intended. At termination, spleens and tumors were excised and their size determined by weight and by volume as described above. Body weight was measured at time of set-up, at tumor inoculation and at take-down. Also in this study, six mice were sacrificed early due to wounds at the tumor side. The mice were evenly distributed throughout all groups.
Cell studies
PBMC killing assay
PBMCs were isolated from blood collected from the mice in the αPD-1 experiment. The blood was collected in EDTA-coated tubes at decapitation and kept at room temperature (RT) until analysis. PBMCs were isolated with Lymphoprep™ (STEMCELL Technologies, Vancouver, Canada) gradient separation following manufacturer’s instructions. YAC-1 target cells purchased from Sigma-Aldrich (St. Louis, MO, USA) were incubated with 15 µM Calcein AM (Invitrogen™, Carlsbad, CA, USA) at a concentration of 106 cells/ml for 30 min at 37 °C, 5% CO2. The experiment was set up in Nunc® (ThermoFisher Scientific) 96-well round bottom plates with triplicate samples in RPMI 1640 with 10.000 target cells pr. well and incubated for 4 h at 37 °C, 5% CO2 before harvesting the supernatants. Fluorescent signal from the supernatants was measured with the Fluostar Optima system at 485 nm excitation and 520 nm emission. Killing capacity in the sample wells with effector-to-target (E:T) ratios of 25:1 was calculated as percentage of fluorescent signal released from positive control wells (target cells in 2% TritonX; Sigma-Aldrich) subtracted the signal released from reference wells. Wells with E:T ratios of 1:1 were set as reference instead of target cells only to account for re-uptake of spontaneously released Calcein by effector cells [Citation17].
Molecular analyses
RNA isolation, cDNA synthesis and RT-qPCR
Total RNA was isolated from frozen tissue samples using Ambion™ TRIzol™ Reagent (InvitrogenTM) according to manufacturer’s instructions and stored at −80 °C. Purity and quantity of the isolated RNA was determined by a Nanodrop 1000 spectrophotometer (ThermoFisher Scientific). Each RNA sample was diluted with Ultrapure Nuclease free water to a final concentration of 25 ng/µl in a total volume of 10 µl. RNA was reverse transcribed into complementary DNA (cDNA), using High-Capacity cDNA Reverse Transcription Kit with random hexamer primers (ThermoFisher Scientific). The resulting cDNA was diluted to a final concentration of 2.5 ng/µl and stored at −20 °C. RT-PCR amplification was performed on the ViiA7 real-time PCR machine (Applied Biosystems, Foster City, CA, USA) using SYBR green as a fluorescent marker (PowerUp SYBR® Green PCR Master Mix, Applied Biosystems). All samples were run in triplicates using 7.5 ng cDNA and 300 nM primer concentrations in MicroAmp® Optical 384-Well Reaction Plate (Life Technologies, Carlsbad, CA, USA) and sealed with MicroAmp™ Optical Adhesive Film (Life Technologies). The qPCR conditions consisted of an initial step of 2 min at 50 °C and 2 min at 95 °C, a second step corresponding to the PCR cycle (40 cycles) with 15 s at 95 °C and 60 s at 60 °C. Data were acquired at the end of this step. A final step was added to obtain a specific denaturation curve from 60 °C to 95 °C with increments of 0.05 °C/s. Purity of the amplified products was checked by observation of a single melting peak. Quantification was performed by using the delta/delta CT method. Expression of target genes was normalized to expression of the reference housekeeping gene 18S. In the case of LLC, Ppia was chosen as the reference housekeeping gene, as we have found this to be most stable across the groups for this tumor model. In comparison of hPD-L1 expression vs. mPd-l1 expression in xenograft tumors, expressions were calculated using the overall average ΔCT of the two genes from experiments using the same primer concentrations. In these tumors, housekeeping gene expression (m18s) varied between the groups with 0.6 CT. All primer sequences are listed in .
Table 1. Primer sequences.
Statistical analysis
All statistical analyses were performed in GraphPad Prism v8.0. The statistical significance of the difference between measurements of mRNA expression between two groups (e.g., Control and Exercise) was obtained from students t-tests or non-parametric Mann–Whitney test if samples did not pass the normality test. For correlation analysis, Pearson correlation test or the non-parametric Spearman correlation was chosen, and values obtained by qPCR were log transformed. For multiple comparisons in the combination therapy studies, two-way ANOVA followed by post hoc tests with Bonferroni corrections were performed. Running data were analyzed with mixed-effects model to account for missing data points due to counter errors. The criterion of significance was set at a probability of less than 0.05.
Results
We investigated the impact of voluntary wheel running on the gene expression of key immune regulatory molecules across several different tumor types, and we submitted mice bearing tumors of the most PA-responsive type to interventions combining checkpoint blockade therapy and voluntary wheel running.
Wheel running regulates intratumoral expression of immune regulatory molecules
Voluntary wheel running led to enhanced expression levels of several key immune regulatory molecules in our B16 melanoma mouse model. The mRNA expression of the checkpoint molecule PD-1 and its two ligands PD-L1 and PD-L2 were increased 2.9-fold (p < 0.01), 2.3-fold (p < 0.01) and 3.1-fold (p < 0.05), respectively, while the mRNA expression of the co-stimulatory molecule CD28 and the two ligands B7.1 and B7.2 increased 2.2-fold (p < 0.05), 2.8-fold (p < 0.0001) and 2.9-fold (p < 0.001), respectively (). In our previous study [Citation4], we have found substantial upregulation of intra-tumoral IFNγ with wheel running, and other studies report induction of PD-L1 expression upon stimulation with IFNγ across several cell types [Citation18]. Correspondingly, we found a tight correlation between intra-tumoral Ifnγ expression and Pd-1 (r2=0.91, p < 0.0001) and Pd-l1 expression (r2=0.85, p < 0.0001) ( and , respectively). In contrast, IFNγ-stimulation of PD-L2 expression was not reported [Citation16], and we found no correlation between Ifnγ and Pd-l2 expression in the tumors (Supplementary Figure 1(A)).
Looking at two other murine tumor models – E0771 breast cancer and Lewis Lung Cancer (LLC) – the 6 immune regulatory molecules did not significantly increase with wheel running in E0771 (), and only Pd-l2 (17-fold, p < 0.01) and Cd28 (2.5-fold, p < 0.05) expression was upregulated in LLC (). In these two models we have not found indications of increased infiltration by immune cells similar to what has been found in B16 melanoma ([Citation19], and Supplementary Figure 1(B)). Interestingly, in both LLC and E0771 we still see pronounced, significant tumor growth-reducing effect of wheel running [Citation19].
As PD-L1 can be expressed on tumor cells, antigen presenting cells (APCs) or stromal fibroblasts [Citation6], we found it relevant to investigate whether wheel running was affecting PD-L1 expression in the stromal compartment. We made use of a human head and neck cancer model (UTSCC45) in mice, which allowed us to differentiate between induction of human PD-L1 mRNA in the tumor cells and murine Pd-l1 expression in the stromal tissue. No induction of the human transcript of PD-L1 was observed with wheel running (), while murine Pd-l1 expression increased 7.1-fold (p < 0.001, ). Primers against human PD-L1 were tested on human tissue as a positive control (data not shown). In this model, tumor growth was also attenuated by voluntary wheel running (unpublished observations).
Combined wheel running and PD-L1 inhibition
Out of the three tumor models reported here, the B16 melanoma model was by far the most responsive to PA with regards to checkpoint molecule expression (). As mentioned, this is also the only model out of these three to show increased immune cell infiltration ([Citation4,Citation19], and Supplementary Figure 1(B)) Thus, we decided that this model was the best suited for investigating a potential benefit from combining wheel running with checkpoint inhibitors in reducing tumor growth. Mice carrying subcutaneous B16 melanoma tumors received 100 µg PD-L1 inhibitor or PBS i.p. thrice per week post tumor injection, whilst being randomized to either control cages or cages with access to running wheels. The running mice had access to the wheels for a period of 5 weeks prior to tumor challenge, and they ran between 0.9 km and 5.8 km per day with no significant difference between the PBS group and the group receiving PD-L1 inhibitor (Supplementary Figure 2(A)). Wheel running significantly suppressed tumor growth (p < 0.05, ), but the statistical test showed no interaction with PD-L1 inhibition. Post hoc analysis showed that wheel running reduced tumor growth by 72% (p = 0.13), while wheel running in combination with PD-L1 inhibitor reduced tumor growth by 83% (p < 0.05) compared with PBS treated mice (). PD-L1 inhibition did not induce any significant changes in body weight () or weight of the spleen (), suggesting that the treatment did not cause major discomfort or affect systemic immune cell expansion.
Figure 2. Combining wheel running and αPD-L1 therapy in B16 melanoma. (A) Tumor volume growth curve of sedentary and wheel running (Wheel) mice treated with PBS or PD-L1 inhibitor annotated ‘αPD-L1’ (n: PBS = 11, Wheel = 8, αPD-L1 = 10, αPD-L1 + Wheel = 11). Growth curves are shown as mean with SD. (C) Tumor weights, (D) change in bodyweight (BW) from time of tumor inoculation, and (E) weight of the spleen were measured at time of euthanization. Data are depicted as individual values with indication of means. Statistical significance was determined by two-way ANOVA with post-hoc Tukey’s multiple comparison test for all panels. *p < 0.05, **p < 0.01, ***p < 0.001.
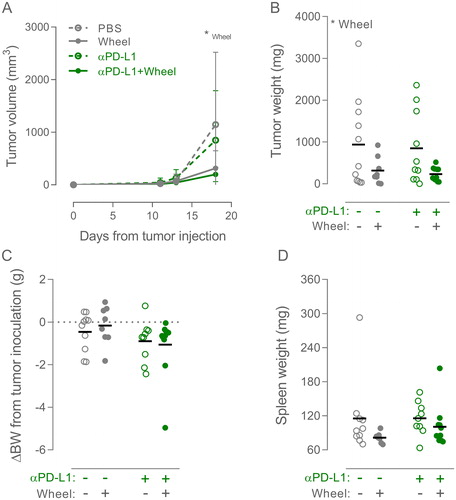
Combined wheel running and PD-1 inhibition
With the PD-L1 inhibitor we observed no additive effect of combination with wheel running, which may partly be explained by the very large suppression in tumor growth by wheel running alone (−72%). Furthermore, some of the PD-1 mediated blockade of T cells might persist with the PD-L1 inhibition, as PD-1 can signal through both PD-L1 and PD-L2 [Citation20]. Considering the finding that wheel running increased both PD-L1 and PD-L2 expression in B16 melanoma (), we investigated the effect of combining wheel running with PD-1 inhibitor treatment.
Mice with or without access to running wheels were inoculated with subcutaneous B16 melanoma tumors and treated with 200 µg PD-1 inhibitor or PBS i.p. twice per week post tumor injection. In this study, the mice ran 4–8 km per day across the groups, with the αPD-1 group running significantly more than the PBS group (Supplementary Figure 2(B)). However, we do not find this difference between the treatment group and the control group to be consistent between the studies (Supplementary Figure 2(A)).
Also in this study, there was significant suppression of tumor growth with wheel running (p < 0.01) (), with the post hoc test showing a 40% reduction compared to the sedentary PBS group (p < 0.05) and a 50% reduction comparing the sedentary group receiving PD-1 inhibitor to the exercising group receiving PD-1 inhibitor (p = 0.07) (). However, we did not find reduced tumor growth as a result of the checkpoint blockade treatment – neither alone nor in combination with wheel running ().
Figure 3. Combining wheel running and αPD-1 therapy in B16 melanoma. (A) Tumor volume growth curve of sedentary or wheel running (Wheel) mice treated with PBS or PD-1 inhibitor annotated ‘αPD-1’ (n: PBS = 13, Wheel = 12, αPD-1 = 12, αPD-1 + Wheel = 12). Growth curves are shown as mean with SD. (B) Tumor weights, (C) change in body weight (BW) from time of tumor inoculation, and (D) weight of the spleen were measured at time of euthanization. (E) Killing capacity of PBMCs isolated from mice from each of the four groups (PBS = 6, EX = 6, αPD-1 = 6, αPD-1 + EX = 7) incubated at E:T ratio of 25:1 with YAC-1 cells. Data are depicted as individual values with indication of means. Statistical significance was determined by two-way ANOVA with post-hoc Tukey’s multiple comparison test for all panels. *p < 0.05, **p < 0.01, ***p < 0.001.
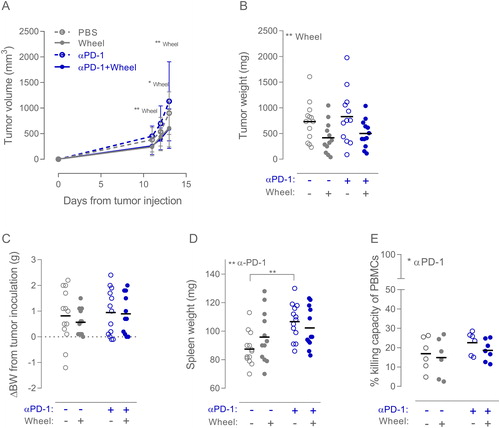
The PD-1 inhibitor did not induce any significant changes in body weight (), but the treatment did increase spleen weight (p < 0.01, ), indicating a systemic immune cell expansion. In line with this, we find a small but significant increase (p < 0.05) in the killing capacity of Peripheral Blood Mononuclear Cells (PBMCs) isolated from the blood of the mice, when they had been receiving PD-1 inhibitor. The average killing capacity of the sedentary PBS group was 17% compared to 23% in the sedentary PD-1 inhibitor group, and 15% in the running group compared to 17% in the running PD-1 inhibitor group (). PBMC cytotoxicity was assessed by Calcein AM release [Citation21] with the NK cell sensitive YAC-1 cell line as target cells.
Discussion
Interventions to obtain a prognostically favorable immunogenic intra-tumoral environment are relevant for successful treatment with immunotherapeutic agents [Citation22]. To this end, it should be recognized that immune cell infiltration and immune checkpoint expression are not static parameters, but dynamic factors, which are regulated by drug treatment and physiological stimuli [Citation2,Citation23]. Here, we demonstrate that PA in the form of voluntary wheel running can provide such physiological stimuli in the B16 melanoma model, leading to enhanced expression of key immune regulatory molecules.
Regulation of the PD-1/PD-L1-axis
We focused on the PD-1/PD-L1-axis regulation, as treatments targeting PD-1 and PD-L1 are the most widely used immunotherapeutic agents at the moment [Citation24].
High expression of the PD-L1 has been associated with improved overall survival in melanoma patients [Citation16]. This was explained by the cytotoxic T cells upregulating their PD-1 expression and secreting IFNγ upon encountering tumor antigens. The secreted IFNγ in turn stimulates both tumor cells and infiltrating immune cells to induce PD-L1 expression [Citation18]. In this context PD-L1 expression could be viewed as a marker of an active anti-tumor immune response.
Recent preclinical studies have investigated the importance of PD-L1 location within tumors, and they collectively demonstrate therapeutic roles of PD-L1 when expressed on either tumor cells or stromal cells, highlighting that regulation of PD-L1 on stromal cells plays a significant role in the response to PD-1/PD-L1 blockade [Citation25–27]. When it comes to the question of whether PA can lead to increased PD-L1 in the stromal tissue, our study with UTSCC45 tumors suggest that this is indeed possible. However, factors stimulated by PA or exercise – such as IFNγ – could induce PD-L1 expression on the tumor cells as well in a syngeneic model. In light of reports stating that PD-L1 induction on both tumor cells and immune cells in the TME can be a symptom of anti-tumor activity [Citation28], this scenario is possible.
Combining exercise and immune checkpoint blockade in mice
Although previous studies did not indicate a role of T-cells in the immunomodulatory effect of wheel running [Citation4], the anti-tumor effects of this intervention, working mainly through NK-cell infiltration, could potentially work additively with T-cell activation using PD1 or PD-L1 blockade. Furthermore, PD-L1 can be expressed on many of the cell types making up a tumor [Citation18] including a number of inflammatory cells such as dendritic cells, macrophages and also NK cells [Citation18,Citation29].
While we hypothesized that combining PD-1/PD-L1 inhibitors with voluntary wheel running could augment tumor growth control further than wheel running alone, this was not the case for either of the two treatments. In line with previously reported studies [Citation2,Citation4], voluntary wheel running reassuringly had a considerable and significant effect on tumor growth, but we did not find an effect of blocking the PD-1/PD-L1-axis; Neither alone or in combination with access to wheels. PD-1 blockade did result in increased spleen weight indicating systemic immune cell expansion, and accordingly, the killing capacity of PBMCs isolated from the mice receiving PD-1 inhibitor was slightly increased. While the adaptations were insufficient in decreasing tumor growth, these data do confirm an effect of the treatment. Furthermore, modulation of the killing capacity of the PBMCs may occur when they reach the tumoral compartment.
These studies suggest that target availability is not the only factor determining the success of PD-1/PD-L1 blockade in the case of B16 melanoma. To this end, we recognize that tumor models in mice do not reflect the full complexity of human cancers [Citation30].
The B16 melanoma tumor model is, however, not the only model not affected by monotherapy using PD-1/PD-L1 blockade. Studies using the syngeneic, subcutaneous MC38 tumors [Citation31] and TUBO tumors [Citation32], or even isografted mouse tumor organoids (MTO) of quadruple-mutant mice, which were reported to develop metastatic intestinal tumors that display key hallmarks of human microsatellite-stable colorectal cancers [Citation33], also do not find an effect of PD1/PD-L1 blockade alone. These three studies did, however, find combination therapies with PD-1/PD-L1 to be effective.
Here we explored the possibility of PA and PD-1/PD-L1 blockade as a combination therapy in a syngeneic tumor model known to respond to PA with regards to both tumor growth reduction, immune cell infiltration, and upregulation of PD-1 and PD-L1 expression. However, our data do not point to an additive effect.
A recently published study investigated the combination of PD-1 blockade and exercise in patient-derived xenograft tumors in mice. Although this study did find the combination to increase tumor necrosis and decrease proliferation marker Ki67, the effects of the treatments on tumor growth were very similar to our observations; while substantial inhibition was observed with exercise, there was no effect of monotherapy with PD-1 blockade on this outcome. Interestingly, the combination treatment in that study did not attenuate tumor growth compared to the control group [Citation15].
Conclusion
This study shows a positive effect of PA with regards to tumor growth and augmented expression of key immune regulatory molecules indicative of a favorable immunogenic tumor microenvironment. This supports existing studies promoting physical activity and exercise as an integrated part of cancer treatment programs in the clinic. The study does not provide evidence of a potential added benefit specifically for patients undergoing immunotherapy with PD-L1 or PD-1 inhibitors.
Author contributions
MLB wrote the manuscript, and MLB and NU designed and performed the experiments and analyzed the data. RS, KSP, TS and MMS assisted with performing the experiments. PH designed the experiments and supervised the work. RS, JFC, BKP and JG contributed with essential ideas and discussion, and JG additionally supervised the writing of the manuscript. All authors have read and approved the final version of the manuscript.
Supplemental Material
Download MS Word (12.8 KB)Supplemental Material
Download TIFF Image (1.3 MB)Supplemental Material
Download TIFF Image (581.9 KB)Disclosure statement
The authors declare no conflict of interest.
Additional information
Funding
References
- Christensen JF, Simonsen C, Hojman P. Exercise training in cancer control and treatment. Compr Physiol. 2018;13:165–205.
- Hojman P, Gehl J, Christensen JF, et al. Molecular mechanisms linking exercise to cancer prevention and treatment. Cell Metab. 2018;27:10–21.
- Betof AS, Lascola CD, Weitzel D, et al. Modulation of murine breast tumor vascularity, hypoxia, and chemotherapeutic response by exercise. J Natl Cancer Inst. 2015;107:1–5.
- Pedersen L, Idorn M, Olofsson G, et al. Voluntary running suppresses tumor growth through epinephrine- and IL-6-dependent NK cell mobilization and redistribution. Cell Metab. 2016;23:554–562.
- Sharma P, Allison JP. Immune checkpoint targeting in cancer therapy: toward combination strategies with curative potential. Cell. 2015;161:205–214.
- Topalian SL, Drake CG, Pardoll DM. Immune checkpoint blockade: a common denominator approach to cancer therapy. Cancer Cell. 2015;27:450–461.
- Herbst R, Soria J, Kowanetz M, et al. Predictive correlates of response to the anti-PD-L1 antibody MPDL3280A in cancer patients. Nature. 2014;515:563–567.
- Powles T, Eder J, Fine G, et al. MPDL3280A (anti-PD-L1) treatment leads to clinical activity in metastatic bladder cancer. Nature. 2014;515:558–562.
- Daud AI, Wolchok JD, Robert C, et al. Programmed death-ligand 1 expression and response to the anti–programmed death 1 antibody pembrolizumab in melanoma. J Cin Oncol. 2016;34:4102–4109.
- Postow MA, Chesney J, Pavli AC, et al. Nivolumab and ipilimumab versus ipilimumab in untreated melanoma. N Engl J Med. 2015;372:2006–2017.
- Weber J, Mandala M, Del Vecchio M, et al. Adjuvant nivolumab versus ipilimumab in resected stage III or IV melanoma. N Engl J Med. 2017;377:1824–1835.
- Robert C, Schachter J, Long G, et al. Pembrolizumab versus ipilimumab in advanced melanoma. N Engl J Med. 2015;372:2521–2532.
- Blank CU, Haanen JB, Ribas A, et al. The “cancer immunogram”. Science. 2016;352:658–660.
- Ruiz-Casado A, Martín-Ruiz A, Pérez LM, et al. Exercise and the hallmarks of cancer. Trends Cancer. 2017;3:423–441.
- Martín-Ruiz A, Fiuza-Luces C, Rincón-Castanedo C, et al. Benefits of exercise and immunotherapy in a murine model of human non-small-cell lung carcinoma. Cancer Metastasis Rev. 2020;39:115–115.
- Taube JM, Anders RA, Young GD, et al. Colocalization of inflammatory response with B7-H1 expression in human melanocytic lesions supports an adaptive resistance mechanism of immune escape. Sci Transl Med. 2012;4:127ra37.
- Iwanowicz LR, Densmore CL, Ottinger CA. Calcein AM release-based cytotoxic cell assay for fish leukocytes. Fish Shell Fish Immunol. 2004;16:127–137.
- Sun C, Mezzadra R, Schumacher TN. Regulation and function of the PD-L1 checkpoint. Immunity. 2018;48:434–452.
- Pedersen KS, Gatto F, Zerahn B, et al. Exercise-mediated lowering of glutamine availability suppresses tumor growth and attenuates muscle wasting. iScience. 2020;23:100978.
- Latchman Y, Wood CR, Chernova T, et al. PD-L2 is a second ligand for PD-1 and inhibits T cell activation. Nat Immunol. 2001;2:261–268.
- Neri S, Mariani E, Meneghetti A, et al. Calcein-acetyoxymethyl cytotoxicity assay: standardization of a method allowing additional analyses on recovered effector cells and supernatants. Clin Diagn Lab Immunol. 2001;8:1131–1135.
- Topalian SL, Hodi FS, Brahmer JR, et al. Safety, activity, and immune correlates of anti-PD-1 antibody in cancer. N Engl J Med. 2012;366:2443–2454.
- Schneider G, Schmidt-Supprian M, Rad R, et al. Tissue-specific tumorigenesis – context matters. Nat Rev Cancer. 2018;17:239–253.
- Sanmamed MF, Chen L. A paradigm shift in cancer immunotherapy: from enhancement to normalization. Cell. 2018;175:313–326.
- Lau J, Cheung J, Navarro A, et al. Tumour and host cell PD-L1 is required to mediate suppression of anti-tumour immunity in mice. Nat Commun. 2017;8:1–11.
- Juneja VR, McGuire KA, Manguso RT, et al. PD-L1 on tumor cells is sufficient for immune evasion in immunogenic tumors and inhibits CD8 T cell cytotoxicity. J Exp Med. 2017;214:895–904.
- Kleinovink JW, Marijt KA, Schoonderwoerd MJA, et al. PD-L1 expression on malignant cells is no prerequisite for checkpoint therapy. Oncoimmunology. 2017;6:e1294299.
- Topalian SL, Taube JM, Anders RA, et al. Mechanism-driven biomarkers to guide immune checkpoint blockade in cancer therapy. Nat Rev Cancer. 2016;16:275–287.
- Dong W, Wu X, Ma S, et al. The mechanism of anti-PD-L1 antibody efficacy against PD-L1 negative tumors identifies NK cells expressing PD-L1 as a cytolytic effector. Cancer Discov. 2019;626:CD-18–1259.
- Olson B, Li Y, Lin Y, et al. Mouse models for cancer immunotherapy research. Cancer Discov. 2018;8:1358–1364.
- Omori R, Eguchi J, Hiroishi K, et al. Effects of interferon-α-transduced tumor cell vaccines and blockade of programmed cell death-1 on the growth of established tumors. Cancer Gene Ther. 2012;19:637–643.
- Deng L, Liang H, Burnette B, et al. Irradiation and anti-PD-L1 treatment synergistically promote antitumor immunity in mice . J Clin Invest. 2014;124:687–695.
- Tauriello DVF, Palomo-Ponce S, Stork D, et al. TGFβ drives immune evasion in genetically reconstituted colon cancer metastasis. Nature. 2018;554:538–543.