Abstract
Purpose
To investigate the dosimetric impact of breathing motion on robustly optimized proton therapy treatment plans for left-sided breast cancer patients with an indication for locoregional irradiation.
Materials and methods
Clinical Target Volumes (CTVs) (left-sided breast, level 1 to 4 axillary lymph nodes, interpectoral and internal mammary lymph node regions) and organs at risk were delineated on 4 D-CTs of ten female patients. After treatment planning to a prescribed dose of 40.05 Gy(RBE) in 15 fractions on the time-averaged CT, the dose was calculated on all ten phases of the breathing cycle. Robustness to setup (5 mm) and range errors (3%) was evaluated for those ten phases. Correlations were evaluated between the phases of the breathing cycle and the D98% of the CTV and the Dmean of the heart.
Results
Correlations coefficients were between −0.12 and 0.29. At the most extreme values of the 28 robustness scenarios, the clinical goals were met for all but two patients. The mean heart dose was 0.41 Gy(RBE) with a standard deviation of 0.31 Gy(RBE) of proton therapy plans.
Conclusion
The effect of breathing motion on the robustness of proton therapy treatment plans for this patient group is minor and not of clinical significance. Based on this patient group, a deep-inspiration breath hold seems to be unnecessary to improve robustness for these patients.
Introduction
Radiotherapy after breast-conserving surgery reduces the risk of recurrence and cancer-related death and is considered the standard of care nowadays [Citation1]. However, conventional radiation therapy may result in a significant dose to surrounding tissues, especially in left-sided breast cancer. The mean heart dose (MHD) is of importance in predicting radiation-induced toxicity [Citation2]. Darby et al. [Citation3] showed that the rate of major coronary events increases linearly with an increase in the MHD with 7.4% per Gray, or even higher in patients with preexisting cardiac risk factors.
There are several ways of minimizing the heart dose, including breath hold techniques and proton therapy [Citation4]. Mast et al. [Citation5] showed in a planning comparison study that proton therapy resulted in a lower heart and left anterior descending artery dose than intensity-modulated photon therapy with breath hold in patients with left-sided breast cancer. Other planning comparison studies support the use of proton therapy in breast cancer patients, especially in patients that also need treatment of the internal mammary lymph nodes and level 1 to 4 axillary lymph nodes [Citation6–9].
The physical characteristics of dose delivery by protons result in more conformal treatment plans, but also make the treatment more sensitive to patient movement, including breathing motion [Citation10]. Robust planning and optimization is a solution to address these sensitivities [Citation11].
In photon radiotherapy, deep-inspiration breath hold (DIBH) is often used in order to minimize breathing motion and to enlarge organ separation and thus reducing the dose to the organs at risk (OAR). Both computer-controlled and non-computer-controlled methods exist, which come with their own advantages and disadvantages [Citation12]. Either way, not all patients are capable of holding their breath long enough to perform the radiation treatment [Citation12].
Several studies were performed to see if DIBH could also reduce the heart dose in proton therapy. Thus far, no dosimetric differences have been found with the use of DIBH in proton therapy [Citation5,Citation8,Citation13–17]. However, some of these studies point out that the use of DIBH may have a positive effect on the robustness of proton therapy plans because of the reduced intra-fraction motion [Citation5,Citation15]. Robust planning in proton therapy is an alternative to PTV-based plan evaluation, since the dose distribution in intensity modulated proton therapy is not necessarily invariant to errors [Citation18].
Flejmer et al. [Citation19] and Öden et al. [Citation13] evaluated treatment plans on different cycles of the breathing phase and concluded that the robustness of their proton treatment plans for breast cancer patients without lymph node involvement was minimally affected by breathing motion. However, in the Netherlands, proton therapy will mostly be offered to breast cancer patients with lymph node involvement, due to the model-based indication approach [Citation20].
Therefore, the aim of this study is to determine the dosimetric effect of breathing motion on the robustness of proton therapy plans for breast cancer patients with locoregional lymph node involvement, including the internal mammary, interpectoral and level 1 to 4 axillary lymph nodes.
Materials and methods
Data acquisition
Anonymized 4 D-CT scans (Philips Brilliance Big Bore, Philips Medical Systems, United States of America) from ten female patients who were treated for thoracic disease were acquired from Haaglanden Medical Center. The 4 D-CT scans were made without contrast and sorted in ten phases of the breathing cycle, where the 0% phase was defined as maximum inspiration. The scans had a slice thickness of 3 mm and the patients were scanned in a supine position with the arms abducted above the head with the help of a lung board (WingSTEP, Elekta AB, Sweden).
Delineation
The glandular left breast tissue, level 1–4 axillary lymph nodes, interpectoral lymph nodes and internal mammary lymph nodes were delineated as clinical target volume (CTV) according to the ESTRO guidelines on radiotherapy of early stage breast cancer [Citation21,Citation22]. CTVs of the level 1–4 axillary lymph nodes, interpectoral lymph nodes and internal mammary lymph nodes were combined in CTVelective. The heart, lungs, skin at treated area and contralateral breast were defined as organs at risk. For the heart delineation, the atlas by Feng et al. was used [Citation23]. The lungs were delineated semi-automatically with the help of built-in function of the treatment planning system RayStation 7 (RaySearch Laboratories, Stockholm, Sweden). The glandular breast tissue and the contralateral breast were cropped 5 mm under the skin.
Both the target areas and the organs at risk were delineated on all ten phases of the 4 D-CT scans.
Planning
All patients were planned in RayStation 7 (RaySearch Laboratories, Stockholm, Sweden), using the time-averaged 4 D-CT. An RBE of 1.1 was assumed for protons and the prescribed mean dose was 40.05 Gy(RBE) in 15 fractions, without a boost dose. Two Intensity Modulated Proton Therapy (IMPT) beams were used. Beam angles were chosen to be the most favorable for each patient’s anatomy and were either a combination of 50 and 350 degrees or 0 and 45 degrees. Treatment planning was robustly optimized with Monte Carlo dose calculations, according to Korevaar et al. [Citation18]. Uncertainty of 1% was used for Monte Carlo dose calculations. Clinical optimization goals were a minimum 95% dose (38.05 Gy(RBE)) to 98% of the clinical target volume (CTVbreast or CTVelective) and a maximum 107% dose (42.85 Gy(RBE)) to 2% of the target volume. For OARs, a maximum equivalent uniform dose with an a-parameter of 1 and dose fall-off were used. Range shifters of 5 cm were used.
Robustness analysis
After the plans were made, they were applied to all phases of the 4 D-CT scans and the robustness evaluation was repeated for all phases. The robustness analysis replaces the CTV-PTV concept, which is used in photon planning, and calculates the dose for various treatment scenarios. For the robustness evaluations, an isotropic set-up error of 5 mm and a range overshoot and undershoot of 3% were used [Citation18]. Twenty-eight scenarios, which are shown in Appendix 1, were evaluated for each breathing phase.
Data analysis
The amplitude of breathing motion was measured on one axial slice at mid-breast level at the mid-sternum point and at the nipple for each patient. A mark was drawn on the maximum inspiration phase, and the distance was measured between the mark on the maximum inspiration phase and any other phase.
Pair-wise (univariate) correlations between each of the 10 breathing phases and the D98% of the CTV and Dmean of the heart were evaluated, resulting in 30 correlation coefficients in total. The correlation was considered significant at a two-sided p-value below 0.05. Furthermore, a graph was made of the results of all scenarios for each breathing phase, for the aforementioned dosimetric parameters.
Results
The amplitude of the breathing motion had a mean value of 2.1 mm (range = 1.2–4.0 mm, SD 0.8 mm) at the mid-sternum position and 2.2 mm (range = 0.7–3.6 mm, SD 0.9 mm) at the nipple position. The ages of all patients and the amplitudes of the breathing motion can be seen in . All nominal dose parameters are shown in . CTV size and the most extreme values of the robustness analysis on all breathing phases are shown in .
Table 1. Age of patients and amplitude of breathing motion at the mid-sternum and nipple positions.
Table 2. Nominal dose parameters for all ten patients.
Table 3. CTV size and extreme values for each patient. over all robustness scenarios of all phases.
In , the dose distributions for patient 4 and 7 are shown as an example. shows the result of the robustness analysis with their correlation analyses for patient 3, 4 and 7, where every dot represents one scenario.
Figure 1. (a) Dose distribution for patient 4; (b) Dose distribution for patient 4; (c) Dose volume histogram for the planning CT of patient 4. (d) Dose distribution for patient 7.
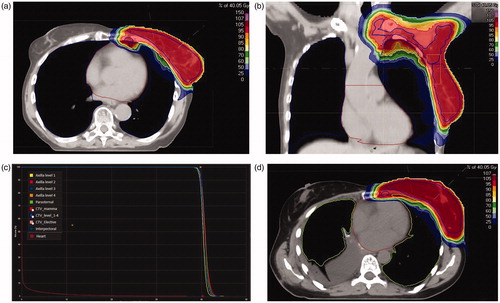
Figure 2. (a) Dose distribution for patient 3; (b) Results of the robustness analysis for patient 4. (c) Results of the robustness analysis for patient 7.
In total, 30 correlation coefficients were computed: the D98% of the CTVs and Dmean of the heart for all ten patients, as shown in . Correlation coefficients (Spearman’s ρ-values) were between −0.12 and 0.29.
Table 4. Correlation coefficients for all patients.
For patient 3, the variation of the D98% for CTVelective is larger in a part of the inspiration phase (70%–90%). For the same phases, the variation in Dmean of the heart seems to be smaller. This effect is not seen in any of the other patients.
For patient 7, there is little variation in the dose for the D98% of the CTV. However, for this patient, there is a larger variation in the MHD: it ranges from 0.4 Gy(RBE) for one scenario in phase 1 to 1.75 Gy(RBE) in phases 6 and 7.
For all patients, the minimum value of the D98% for the CTV was larger than 95% of the prescribed dose. The maximum value of the D2% was smaller than 107% of the prescribed dose for all patients but two, in all breathing phases. For these patients, the maximum value of the D2% were 107.7% and 107.1% of the prescribed dose, respectively. The largest MHD was 1.75 Gy(RBE) and the largest mean dose on the contralateral breast was 0.44 Gy(RBE).
Discussion
It is known that breathing motion has little effect on dosimetric parameters when making proton plans (without robustness evaluation) for breast cancer patients with and without lymph node involvement [Citation5,Citation8,Citation13–17]. Also, the robustness is minimally influenced by the breathing motion when assessing left-sided breast cancer patient without lymph node involvement [Citation13,Citation19]. This study shows that, even with the addition of the level 1 to 4 axillary lymph nodes, internal mammary lymph nodes and interpectoral lymph nodes to the target volume, the effect of the breathing motion on plan robustness is very limited.
For this study, 4 D-CTs from ten female patients who were treated for thoracic disease were used. To be certain the data would be applicable to breast cancer patients, the amplitude of the breathing motion was measured, which had a mean value of 2.1 mm (range: 1.2–4.0 mm) at the mid-sternum position and 2.2 mm (range: 0.7–3.6 mm SD = 0.9 mm) at the nipple. These measurements correspond to breathing motion amplitudes from breast cancer patients in the literature: studies by Richter et al. and Wang et al. reported an average breast movement of 1.8 mm and 2.1 mm [Citation24,Citation25].
In the current study, the correlation between the breathing phase and the D98% for the CTV (split into CTVbreast and CTVelective) and the correlation between the breathing phase and the Dmean for the heart were calculated, since these parameters were considered most important. Corresponding correlation coefficients were between −0.12 and 0.29, indicating a non-existing to very weak correlation.
There were some minor effects visible in the robustness analysis graphs ( and ). However, none of these were consistent throughout all patients. Combined with the non-significant or very weak correlation coefficients, it can be concluded that the breathing motion does not have a meaningful correlation with the D98% of the CTV and the Dmean of the heart. For patient 7, Dmean of the heart was 1.02 Gy(RBE) and a strong dependence of Dmean on breathing phases was observed (see ), probably because of a large heart movement within the breathing cycle. For this patient, the amplitude of the breathing motion was 1.4 and 1.9 mm for mid-sternum and nipple, respectively (see ).
The results of the variation of the MHD of this study should not only be attributed to the breathing movement, but also to the movement of the heart itself. In a study done by Tong et al., the left ventricle displacement was 1.0, 4.1 and 1.9 mm in the X, Y and Z directions [Citation26]. This means that the movement of the heart may result in moving the heart in and out of the treatment beam.
The results also indicate that even when taking into account the most extreme values of the 28 robustness scenarios, the clinical goals are met for all patients but two. For the first patient, the maximum values of the D2% for the CTVelective exceeded the prescribed dose by 7.7%. However, this was the case for all phases of the breathing cycle, indicating that there is no coherence with the breathing motion.
Limitations
Firstly, one of the limitations of this study is the limited sample size.
Also, the left anterior descending artery was not delineated in this study, even though this structure seems to be important in cardiac events related to breast radiotherapy [Citation2]. It was decided to only focus on the mean heart dose because the risk model by Darby, which is also used in the Dutch Indication Protocol for Protons, only includes the mean heart dose [Citation3,Citation27]. Furthermore, no contrast was used in the 4 D-CT scans and as a result, delineation would have been too unreliable.
In this study, the role of the interplay effect was not looked into. In previous research, interplay effects in amplitudes below 3 mm resulted in a PTV dose inhomogeneity of around 1% [Citation28]. Knopf et al. concluded that the impact of the interplay effect of amplitudes below 6 mm is limited [Citation29]. Since the mean amplitude in this study was even lower than these values, we concluded that the interplay effect should not raise any clinical concerns for this patient group.
This study focuses on the intrafractional movement during proton radiation, but not on the interfractional movements. Correct and reproducible patient positioning is crucial to limit the effect of interfractional movements.
In theory, the robustness of proton therapy plans may further improve by using three beams instead of the two beams that were used in this study. This would be an interesting line of research for the future. Ares et al. even use four treatment beams for breast cancer patients with lymph node involvement and a boost dose [Citation6]. While this may be a valid option, the number of beams will be limited by the number of monitor units per beam.
Conclusion
The effect of breathing motion on the robustness of proton therapy treatment plans for left-sided breast cancer patients with locoregional lymph node involvement is minor and not of clinical significance. The mean heart dose was 0.41 Gy(RBE) with a standard deviation of 0.31 Gy(RBE) of proton therapy plans. Based on this study, a deep-inspiration breath hold seems to be unnecessary to improve robustness for this patient group. The use of at least two beams for proton planning is recommended to achieve robust treatment planning. Further research on 4 D-CT for this patient group is needed to explore the effects of breathing motion with larger amplitudes on robustness.
Supplemental Material
Download MS Word (47.5 KB)Acknowledgments
We acknowledge Dr. N.C.M.G. van der Voort van Zyp and Haaglanden Medical Center for supporting this research and Y. Wang for providing the scripts.
Disclosure statement
No potential conflict of interest was reported by the author(s).
References
- Early Breast Cancer Trialists’ Collaborative Group. Effect of radiotherapy after breast-conserving surgery on 10-year recurrence and 15-year breast cancer death: meta-analysis of individual patient data for 10 801 women in 17 randomized trials. Lancet. 2011;378(9804):1707–1716.
- Nilsson G, Holmberg L, Garmo H, Duvernoy O, Sjögren I, Lagerqvist B, et al. Distribution of coronary artery stenosis after radiation for breast cancer. J Clin Oncol. 2012;30(4):380–386.
- Darby S, Ewertz M, McGale P, Bennet A, Blom-Goldman U, Brønnum D, et al. Risk of ischemic heart disease in women after radiotherapy for breast cancer. N Engl J Med. 2013;368(11):987–998.
- Drost L, Yee C, Lam H, Zhang L, Wronski M, McCann C, et al. A systematic review of heart dose in breast radiotherapy. Clinical Breast Cancer. 2018;18(5):e819–e824.
- Mast M, Vredeveld E, Credoe H, van Egmond J, Heijenbrok M, Hug E, et al. Whole breast proton irradiation for maximal reduction of heart dose in breast cancer patients. Breast Cancer Res Treat. 2014;148(1):33–39.
- Ares C, Khan S, MacArtain A, Heuberger J, Goitein G, Gruber G, et al. Postoperative proton radiotherapy for localized and locoregional breast cancer: potential for clinically relevant improvements? Int J Radiat Oncol Biol Phys. 2010;76(3):685–697.
- Lin L, Vennarini S, Dimofte A, Ravanelli D, Shillington K, Batra S, et al. Proton beam versus photon beam dose to the heart and left anterior descending artery for left-sided breast cancer. Acta Oncologica. 2015;54(7):1032–1039.
- Flejmer A, Edvardsson A, Dohlmar F, Josefsson D, Nilsson M, Witt Nyström P, et al. Respiratory gating for proton beam scanning versus photon 3D-CRT for breast cancer radiotherapy. Acta Oncologica. 2016;55(5):577–583.
- Stick L, Yu J, Maraldo M, Aznar M, Pedersen A, Bentzen S, et al. Joint estimation of cardiac toxicity and recurrence risks after comprehensive nodal photon versus proton therapy for breast cancer. Int J Radiat Oncol Biol Phys. 2017;97(4):754–761.
- Seco J, Robertson D, Trofimov A, Paganetti H. Breathing interplay effects during proton beam scanning: simulation and statistical analysis. Phys Med Biol. 2009;54(14):N283–N294.
- Liu W, Zhang X, Li Y, Mohan R. Robust optimization of intensity modulated proton therapy. Med Phys. 2012;39(2):1079–1091.
- Boda-Heggeman J, Knopf A, Simeonova-Chergou A, Wertz H, Stieler F, Jahnke A, et al. Deep inspiration breath hold-based radiation therapy: a clinical review. Int J Radiat Oncol Biol Phys. 2016;94(3):478–492.
- Ödén J, Toma-Dasu I, Eriksson K, Flejmer A, Dasu A. The influence of breathing motion and a variable relative biological effectiveness in proton therapy of left-sided breast cancer. Acta Oncologica. 2017;56(11):1428–1436.
- Patel S, Lu H, Nyamwanda J, Jimenez R, Taghian A, MacDonald S, et al. Postmastectomy radiation therapy technique and cardiopulmonary sparing: a dosimetric comparative analysis between photons and protons with free breathing versus deep inspiration breath hold. Pract Radiat Oncol. 2017;7(6):e377–e384.
- Ranger A, Dunlop A, Hutchinson K, Convery H, Maclennan M, Chantler H, et al. A dosimetric comparison of breast radiotherapy techniques to treat locoregional lymph nodes including the internal mammary chain. Clinical Oncology. 2018;30(6):346–353.
- Edvardsson A, Nilsson M, Amptoulach S, Ceberg S. Comparison of doses and NTCP to risk organs with enhanced inspiration gating and free breathing for left-sided breast cancer radiotherapy using the AAA algorithm. Radiat Oncol. 2015;10(1):84.
- Yu J, Park S, Herman M, Langen K, Mehta M. Feigenberg S. Free breathing versus breath-hold scanning beam proton therapy and cardiac sparing in breast cancer. Int J Particle Ther. 2016;3(3):407–413.
- Korevaar E, Habraken S, Scandurra D, Kierkels R, Unipan M, Eenink M, et al. Practical robustness evaluation in radiotherapy – a photon and proton-proof alternative to PTV-based plan evaluation. Radiother Oncol. 2019;141:267–274.
- Flejmer A, Chehrazi B, Josefsson D, Toma-Dasu I, Dasu A. Impact of physiological breathing motion for breast cancer radiotherapy with proton beam scanning - an in silico study. Phys Med. 2017;39:88–94.
- Langendijk J, Lambin P, De Ruysscher D, Widder J, Bos M, Verheij M. Selection of patients for radiotherapy with protons aiming at reduction of side effects: the model-based approach. Radiother Oncol. 2013;107(3):267–273.
- Offersen B, Boersma L, Kirkove C, Hol S, Aznar M, Biete Sola A, et al. ESTRO consensus guideline on target volume delineation for elective radiation therapy of early stage breast cancer. Radiother Oncol. 2015;114(1):3–10.
- Offersen B, Boersma L, Kirkove C, Hol S, Aznar M, Sola A, et al. ESTRO consensus guideline on target volume delineation for elective radiation therapy of early stage breast cancer, version 1.1. Radiother Oncol. 2016;118(1):205–208.
- Feng M, Moran JM, Koelling T, et al. Development and validation of a heart atlas to study cardiac exposure to radiation following treatment for breast cancer. Int J Radiat Oncol Biol Phys. 2011;79(1):10–18.
- Richter A, Sweeney R, Baier K, Flentje M, Guckenberger M. Effect of breathing motion in radiotherapy of breast cancer. Strahlenther Onkol. 2009;185(7):425–430.
- Wang W, Li J, Hu H, Li F, Xu M, Sun T, et al. Correlation between target motion and the dosimetric variance of breast and organ at risk during whole breast radiotherapy using 4DCT. Radiat Oncol. 2013;8(1):111.
- Tong Y, Yin Y, Lu J, Liu T, Chen J, Cheng P, et al. Quantification of heart. pericardium. and left ventricular myocardium movements during the cardiac cycle for thoracic tumor radiotherapy. OTT. 2018;11:547–554.
- Landelijk Platform Radiotherapie Mammacarcinoom en Landelijk Platform Protonentherapie. Landelijk Indicatie Protocol Protonen Therapie; 30 October 2018. [Accessed 2019 Jan 9]. Available from: http://www.nvro.nl/images/LIPP_mamma_06112018_definitief_opgestuurd_naar_ZiN.pdf.
- Protik A, van Herk M, Witte M, et al. The impact of breathing amplitude on dose homogeneity in intensity modulated proton therapy. Phys Imag Radiat Oncol. 2017;3:11–16.
- Knopf A, Hong T, Lomax A. Scanned proton radiotherapy for mobile targets-the effectiveness of re-scanning in the context of different treatment planning approaches and for different motion characteristics. Phys Med Biol. 2011;56(22):7257–7271.