Abstract
Background
Paclitaxel is a taxane-based chemotherapeutic agent used as a treatment in breast cancer. There is no effective prevention or treatment strategy for the most common side effect of peripheral neuropathy. In this manuscript, we reviewed the molecular mechanisms that contribute to paclitaxel-induced peripheral neuropathy (PIPN) with an emphasis on immune-related processes.
Methods
A systematic search of the literature was conducted in PubMed, EMBASE and Cochrane Library. The SYRCLE’s risk of bias tool was used to assess internal validity.
Results
156 studies conducted with rodent models were included. The risk of bias was high due to unclear methodology. Paclitaxel induces changes in myelinated axons, mitochondrial dysfunction, and mechanical hypersensitivity by affecting ion channels expression and function and facilitating spinal transmission. Paclitaxel-induced inflammatory responses are important contributors to PIPN.
Conclusion
Immune-related processes are an important mechanism contributing to PIPN. Studies in humans that validate these mechanistic data are highly needed to facilitate the development of therapeutic strategies.
Introduction
Breast cancer is the most common malignancy in women, affecting one in eight to ten women during their lifetime [Citation1]. Paclitaxel is a taxane-based chemotherapeutic agent used as a treatment in both early-stage and metastatic breast cancer [Citation2,Citation3]. Taxanes are microtubule-stabilizing agents that disrupt microtubule-dependent processes that arrest the cell cycle and induce apoptosis of dividing cells [Citation3,Citation4]. Given the broad involvement of microtubules in various cellular processes, microtubule stabilization also affects non-mitotic cells, including peripheral neurons. As a consequence, paclitaxel causes various disabling side effects of which peripheral neuropathy is the most commonly reported neurotoxic side effect (11–87%) [Citation5]. The most common distressing symptom of peripheral neuropathy is sensory dysfunction that may manifest as numbness, tingling, hyperalgesia, allodynia, and spontaneous pain, which are typically symmetrical in a ‘glove and stocking’ distribution [Citation3,Citation6,Citation7]. These symptoms vary in intensity, but severity increases with escalating total doses of paclitaxel [Citation8]. Paclitaxel-induced peripheral neuropathy severely reduces the quality of life of patients [Citation2,Citation6], and limits treatment with high and substantial cumulative doses of paclitaxel, which may impair cancer outcomes (i.e., recurrence and survival) [Citation2].
Various studies have been conducted to assess the effect of neuroprotective agents in the prevention or treatment of peripheral neuropathy [Citation9]. Up to now, no agents have been officially approved for prevention or treatment. In order to develop strategies to counteract peripheral neuropathy, an understanding of the cellular and molecular basis of paclitaxel-induced damage to non-tumor cells is required. Different mechanisms may lead to paclitaxel-induced neurotoxicity, including the engagement of immune-related processed and neuro-inflammatory responses in the nervous system, axonal damage, mitochondrial dysfunction, and increased spinal transmission [Citation10–14].
Various approaches have been taken to investigate paclitaxel-induced peripheral neuropathy, including in vitro studies using cultures of primary sensory neurons or cell lines, in vivo studies using rat and mice models, and human genetic studies, but still limited [Citation14]. Although it is challenging to replicate all patient-reported symptoms in rodent models (e.g., numbness and tingling rely on the verbal report), they do provide insight into evoked pain-like behaviors such as hyperalgesia and allodynia [Citation7] and proxies of nerve damage, therefore resembling peripheral neuropathy in human.
Here we aim to review the molecular mechanisms that contribute to the development of paclitaxel-induced peripheral neuropathy with an emphasis on immune activation and neuro-inflammatory processes using a systematic review to reduce bias. Furthermore, the clinical relevance of these proposed molecular mechanisms is discussed in relation to the development of targeted therapeutic strategies.
Methods
Search strategy
A Population Intervention Comparison Outcome (PICO) framework was used to make a structured search strategy. This search strategy was used to conduct a search in PubMed, EMBASE and Cochrane Library. The search was performed on 19 October 2020. Only English articles reporting on animal and human studies were considered eligible. No additional filters were applied to ensure a search of the literature as comprehensively as possible. The full search strategy is provided in Additional file 1.
Study selection and inclusion and exclusion criteria
The citations and abstracts of all articles were uploaded into the reference database ‘Mendeley’ and checked for duplicates. After merging duplicates, the citations and abstracts were uploaded into the systematic review web app Rayyan [Citation15]. Remainder articles were checked for duplicates and merged. Two researchers (CJCV and AEH) independently screened all titles and abstracts. Subsequently, the full-texts of articles that were potentially eligible for inclusion were assessed. Discrepancies were resolved by discussion and if necessary a third reviewer (NE) was consulted. The inclusion criteria for studies were specified in advance and were as follows: 1) design: randomized controlled trials (RCTs) or controlled trials (CTs); 2) population: women (aged 18 years or older, diagnosed with breast cancer) or animals (with or without a tumor) in vivo; 3) intervention: paclitaxel administration; 4) control: an appropriate control comparison group not receiving paclitaxel at any time point during the study, and 5) outcome: underlying molecular mechanisms of paclitaxel-induced peripheral neuropathy.
Risk of bias assessment
Two researchers (CJCV and AEH) independently assessed the internal validity of included studies using the SYstematic Review Center for Laboratory animal Experimentation (SYRCLE) risk of bias tool [Citation16], a tool based on the Cochrane Collaboration risk of bias tool [Citation17] adapted to include aspects of bias in experimental animal studies. The tool comprises ten entries, which represent six types of bias: selection bias, performance bias, detection bias, attrition bias, reporting bias and ‘other’ biases. The score ‘yes’ indicates a low risk of bias, whereas the score ‘no’ indicates a high risk of bias and ‘unclear’ states that insufficient details have been reported to assess the risk of bias properly. To overcome the problem of judging too many items as unclear, six additional study quality indicators were extracted: 1) any measure of randomization; 2) any measure of blinding; 3) sample size calculation; 4) temperature regulation; 5) ethical approval, and 6) conflict of interest statement. These additional items were independently assessed by two researchers (CJCV and AEH), where the score ‘yes’ indicates reported and the score ‘no’ indicates unreported.
Data extraction
A qualitative summary of the available evidence was produced to elucidate the underlying molecular mechanisms of paclitaxel-induced peripheral neuropathy.
Results
Study selection process
See for details of the study selection process. The search strategy yielded 4059 articles. The selection process resulted in 156 eligible studies.
Study characteristics
Details of the study characteristics are shown in Additional file 2. No eligible studies conducted in humans were identified, indicating the lack of mechanistic work in humans with paclitaxel-induced peripheral neuropathy. As a result, all articles included in this systematic review involved studies conducted with rodents.
Since the pain cannot be directly measured in animals, it is inferred from pain-like behaviors, such as withdrawal from a sensory stimulus [Citation18]. The most commonly used behavioral method to quantify nociception was stimulus-evoked, which could be further subdivided by the stimulus modality – mechanical, heat, and cold.
Risk of bias and quality of reporting
The risk of bias and study quality assessment of individual studies is represented in Additional file 3. When assessing the individual items of the SYRCLE’s risk of bias tool, most often the risk of bias was unclear due to insufficient reporting of the methodology (). Random and blinded group allocation was most poorly reported or lacked details of methods used. Studies that matched intervention groups and vehicle control groups on important baseline characteristics (e.g., pain thresholds and body weight) were indicated as low risk of selection bias. Studies that were performed in a controlled environment (e.g., temperature regulation of the animal room) were indicated as low risk of performance bias.
Figure 2. Risk of bias graphs. Graph A depicts the assessment of the risk of selection, performance, detection, attrition and other biases using the SYRCLE’s risk of bias tool. Graph B depicts the reporting of six key quality indicators. Review author’s decision of individual items are represented as absolute numbers of included studies.
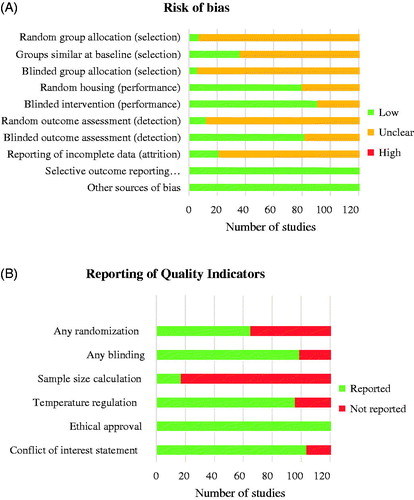
The overall reporting of quality indicators for the 156 included studies is shown in . In 65 (42%) studies, the intervention groups were randomized in some way. Blinding of the experimenter at any level was reported in 100 studies (64%). In 17 studies (11%), the chosen group sizes were supported by a sample size calculation. More than half of the studies (62%) reported that the temperature of the animal room was regulated within a physiological range. A statement of ethical approval was present in all studies. In the majority of studies (67%), a conflict of interest statement was present.
Molecular mechanisms underlying paclitaxel-induced peripheral neuropathy
Axonal damage
Two weeks of paclitaxel treatment causes a smaller mean axon diameter in sciatic nerves [Citation19] and a loss of axon area density [Citation20,Citation21], resulting in sustained (i.e., after three months of recovery) axonal damage [Citation22] . Paclitaxel treatment increases the G-ratio (ratio between the axon diameter and diameter of the axon with myelin sheet) [Citation23,Citation24], which may result from a reduced mean axon diameter or thinning of the myelin sheet [Citation19,Citation25,Citation26]. Compared to control, sensory nerve action potential amplitudes are reduced in paclitaxel-treated mice [Citation19,Citation26]. Degenerated nerve fibers are most often present at distal nerve segments compared to proximal nerve segments of paclitaxel-treated mice, indicating that axonal damage is length-dependent [Citation21]. Nevertheless, abnormalities such as decreased density of myelinated axons are also found in the axons of the dorsal roots [Citation24].
Figure 3. Schematic overview of dorsal root ganglion and spinal cord to indicate the various molecular mechanisms that contribute to paclitaxel-induced peripheral neuropathy. Paclitaxel administration to rodents induces damage to distal nerves observed as a loss of peripheral nerve fibers most pronounced at the terminal endings, thinning of myelin sheet surrounding mainly large nerve fibers, leading to reduced nerve conduction velocity and damage to neurons, as observed by an increase in ATF3, caspase-3 and TUNEL expression in the somatas of sensory neurons. Nerve damage may be caused by mitochondrial dysfunction: paclitaxel treatment results in swollen and vacuolated mitochondria with reduced ATP production. Faulty mitochondrial function and inadequate expression of several antioxidant enzymes (SOD, GPx, GSH, MDA) lead to increased intracellular ROS levels. ROS may activate the NLRP3 inflammasome in peripheral nerves and induce apoptosis. Additionally, expression and activity changes of TRP channels (e.g., TRPV1) and ion channels (e.g., Nav1.7) are observed, resulting in changes in excitability. Activation of increased translation controls may pertain to these changes in the excitability of sensory neurons. These changes are (partially) induced by mast cell degranulation and release of mediators (e.g., tryptase) that activate TLR4, further stimulating inflammatory responses, including the release of pro-inflammatory cytokines and chemokines that may affect transduction and excitability. Paclitaxel is capable of directly activating TLR4, directly stimulating an inflammatory response. Furthermore, other TLR receptors, such as TLR9, contribute to pain development in paclitaxel-treated rodents. Damaged neurons release damage-associated molecular patterns (DAMPs), which further activate TLR4 on various immune cells. Furthermore, various chemotactic cytokines secreted by damaged neurons recruit immune cells, leading to immune cell recruitment and activation in the DRG and the spinal cord. Macrophages, predominantly skewed to the pro-inflammatory M1 type, infiltrate the DRG, where they release pro-inflammatory cytokines that activate and sensitize sensory neurons. Macrophages also release HMGB1, which activates TLR4 on sensory neurons to contribute to pain development. Some reports indicate T cell recruitment to the DRG and spinal cord. T cells can produce the anti-inflammatory IL-10, which may aid in resolution of paclitaxel-induced transient neuropathy. Activated immune cells and also neurons secrete a plethora of inflammatory mediators, such as TNF, IL-17, IL-20 and CCL2, activating a variety of downstream inflammatory pathways. Within the spinal cord, paclitaxel treatment causes glial cell activation of predominantly spinal cord astrocytes, downregulation of glutamate uptake transporters on astrocytes and induction of cytokine release by astrocytes. Microglia activation is less clear. Finally, paclitaxel affects synaptic transmission regulation through upregulation of 5-HT1a receptors and neuropeptide substance P expression. Spinal excitatory synaptic transmission is also increased through σ1R and LPA receptors and dysregulated through activation of presynaptic NMDARs.
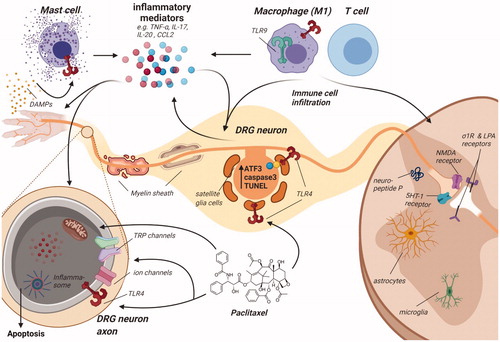
Various studies report loss of intra-epidermal nerve fibers (IENFs) in the hind paw skin after paclitaxel treatment, which indicates damage to peripheral nerve endings and is associated with neuropathic pain behaviors [Citation26–32]. Mitochondrial dysfunction likely contributes to this loss of peripheral nerve endings, since paclitaxel-induced mitochondrial damage is most pronounced in distal nerve segments compared to more proximal nerve segments [Citation33].
Signs of nerve damage after paclitaxel treatment are also found in the somatas of sensory neurons in the dorsal root ganglion (DRG) [Citation25]. ATF3, a marker of sensory cell injury, is increased in a subpopulation of predominantly large and medium, but to a lesser extent small-diameter sensory neurons [Citation25,Citation28,Citation34,Citation35]. Increased expression of ATF3 is most abundant in lumbar DRG neurons of paclitaxel-treated rats compared to trigeminal and thoracic DRG neurons, which might be due to the difference in axon length (i.e., lumbar DRG neurons have longer axons) [Citation34]. Additional evidence of damage to DRG neurons comes from studies reporting an increase in caspase-3 immunoreactivity in large diameter sensory neurons [Citation27,Citation36] or TUNEL-positive sensory neurons [Citation27].
Although the majority of studies show that paclitaxel-induced neuropathic pain is associated with axonal damage, some studies did not observe changes in the total number and density of myelinated axons, ATF3-positive DRG neurons, and microtubule numbers after paclitaxel treatment in rats, whilst pain-like behaviors were present [Citation23,Citation37]. In these studies, the cumulative dosage of paclitaxel was lower than in studies where axonal damage was observed, arguing that nerve damage may be limited to higher concentrations, whilst the development of neuropathic-like pain behaviors is not.
Sensory neurons employ cellular processes to maintain their integrity and protect themselves against neurotoxic agents. For example, nicotinamide mononucleotide adenylyl transferases (NMNATs) are indispensable in axonal and neuronal maintenance and protect against axonal degeneration in various neurodegenerative disease states [Citation38]. Nevertheless, a 50% knockdown of NMNAT2 does not affect the development or maintenance of paclitaxel-induced peripheral neuropathy [Citation38]. Similarly, neuronal function and survival are controlled by the neurotrophic factor nerve growth factor (NGF) by binding to tyrosine kinase receptor A (TrkA) and NGF-trkA signaling is involved in mechanical allodynia in paclitaxel-induced neuropathy [Citation39]. Decreased NGF levels are found in sciatic nerves of paclitaxel-treated rats, which is associated with the severity of neuropathy [Citation40,Citation41].
Mitochondrial dysfunction
Paclitaxel treatment increases the prevalence of atypical, swollen, and vacuolated mitochondria in the axons of myelinated as well as unmyelinated nerve fibers in a time course consistent with observed pain-like behavior [Citation23,Citation33,Citation37,Citation42,Citation43]. Furthermore, it causes deficits in mitochondrial respiration and ATP production possibly through reducing complex I and II activity [Citation44,Citation45]. Mitochondrial dysfunction produces a bioenergetic deficit and nicotinamide riboside, a vitamin B3 precursor of NAD+, prevents paclitaxel-induced peripheral neuropathy by ameliorating the decreased levels of NAD + in the sciatic nerve of paclitaxel-treated rats [Citation31]. The B vitamins nicotinamide, thiamin, and riboflavin exhibit antinociceptive activity in paclitaxel-induced neuropathic pain and reduce inflammatory cytokines such as TNF-α in DRG [Citation46]. Furthermore, paclitaxel reduces peroxisome proliferator-activated receptor gamma coactivator 1-alpha (PGC1α), a master regulator of mitochondrial biogenesis in sensory neurons [Citation32,Citation47] and expression of MFN2, a protein regulating mitochondrial transport and fusion [Citation48].
Mitochondria are a major source of reactive oxygen species (ROS) [Citation49]. Paclitaxel increases levels of mitochondrial superoxides in DRG neurons [Citation50], and ROS in non-peptidergic DRG neurons and the spinal dorsal horn (SDH) prior to and during development and maintenance of paclitaxel-induced hypersensitivity [Citation49]. Blocking oxidative NADPH oxidase (NOX)-4, a subunit of an enzyme family that produces ROS diminishes paclitaxel-induced pain in rats [Citation51]. In addition, administration of a nonspecific ROS scavenger inhibits paclitaxel-induced Nod-like receptor protein 3 (NLRP3) inflammasome activation and simultaneously diminishes paclitaxel-induced neuropathic pain [Citation42]. Moreover, anti-oxidant treatment reverses mitochondrial dysfunction and protects neurons from paclitaxel-induced peripheral neuropathy through activation of phosphoinositide-3-kinase (PI3K)/protein kinase B (AKT) and PGC1α pathways [Citation47]. Paclitaxel weakens survival pathways of PI3K/AKT cells through activating tumor suppressor gene p53 and duloxetine alleviates paclitaxel-induced p53 levels to reverse paclitaxel-induced oxidative stress and apoptosis [Citation52].
In addition to the dysfunction of mitochondria, inadequate endogenous antioxidant responses may contribute to enhanced ROS levels [Citation49]. Indeed, expression of proteins of the endogenous antioxidant system such as superoxide dismutase (SOD), glutathione peroxidase (GPx), glutathione (GSH) and malondialdehyde (MDA) [Citation32,Citation51,Citation53–55], and erythroid 2-related factor 2 (Nrf2) [Citation56,Citation57] in the DRG and/or peripheral sensory nerves of paclitaxel-treated rats is decreased. Duloxetine, a serotonin-norepinephrine reuptake inhibitor, and gallic acid, a natural phenolic compound, reduce paclitaxel-induced neuropathic pain via promotion of the anti-oxidant cascade [Citation54,Citation58].
Transduction, excitability, and synaptic transmission
Transduction: Transient receptor potential cation channels
Transient receptor potential (TRP) cation channels are widely expressed in the nervous system and can function as noxious stimuli detectors [Citation59]. Paclitaxel treatment increases the expression of several TRP channels () in DRG neurons, resulting in mechanical, cold or heat hypersensitivity.
Table 1. Increased expression of TRP channels following paclitaxel treatment.
Various studies have tried to identify the endogenous mediators involved in paclitaxel-dependent activation or sensitization of TRP channels. Paclitaxel increases the cytochrome-P450 epoxygenases metabolite of linoleic acid, 9,10-EpOME (9,10-epoxy-12Z-octadecenoic acid) in DRGs of paclitaxel-treated mice [Citation72]. This lipid sensitizes TRPV1 via a cAMP–PKA-dependent mechanism, causing the enhanced frequency of spontaneous excitatory postsynaptic currents in lamina II neurons of spinal cord cultures.
Additionally, paclitaxel is a ligand for toll-like receptor 4 (TLR4) [Citation73]. Paclitaxel increases the expression of TRPV1 specifically in TLR4-expressing DRG neurons [Citation63]. Moreover, paclitaxel activates TLR4 on satellite glial cells to release TNF that increases TRPA1 and TRPV4 expression in surrounding DRG neurons [Citation73]. TRP channels may also be regulated through proteinase-activated receptor 2 (PAR2) signaling. TLR4-dependent activation of mast cells and the release of tryptase engages PAR2 signaling in neurons [Citation65]. PAR2 is co-expressed with TRPV1, TRPV4 and TRPA1 and blocking PAR2 or its downstream signaling pathways attenuates paclitaxel-induced neuropathic pain [Citation65,Citation74–76]. In support of the role of mast cells, stabilizing mast cells prevents paclitaxel-induced neuropathic pain and blocks sensitization of TRPV1 upon paclitaxel treatment [Citation77]. Finally, TRPV4 may be sensitized through activation of kinin receptors that play a role in the development of paclitaxel-induced mechanical hypersensitivity, although the pathways that lead to kinin receptor activation are unknown [Citation78,Citation79].
Excitability
Voltage-gated ion channels are key regulators of DRG neuronal excitability. DRG neurons exhibit ectopic spontaneous activity after paclitaxel treatment [Citation80]. Many studies have described a change in expression and function of these channels associated with paclitaxel-induced neuropathic pain () [Citation63,Citation81,Citation82,Citation86–88,Citation91,Citation92]. Although the precise mechanisms that govern changes in the excitability of DRG neurons after paclitaxel are not well understood, these changes in paclitaxel-induced neuropathic pain are possibly driven through enhanced protein translation, exemplified by increased MNK-eIF4E phosphorylation [Citation93].
Table 2. Increased expression of voltage-gated channels following paclitaxel treatment.
Spinal transmission
Paclitaxel treatment induces glutamate release from primary afferent nerves through tonic activation of presynaptic N-methyl-D-aspartate receptors (NMDAs) [Citation94–96], which depends on activation of CaVα2δ-1 [Citation94] or metabotropic glutamate receptor 5 (mGLluR5) and protein kinase C (PKC) [Citation95,Citation96]. Paclitaxel-induced pain corresponds with a decreased expression of the spinal glutamate transporter, EAAT2, and enhanced expression of VGLUT2 and synapthophysin (i.e., a marker for identifying presynaptic axon terminal) [Citation97]. This loss of balance between glutamate release and uptake in the dorsal horn of the spinal cord contributes to paclitaxel-induced neuropathic pain. Furthermore, immature spine formation in the spinal cord may drive the persistence of neuropathic pain. Paclitaxel increases expression of slit-robo GTPase activating protein 3 (SrGAP3), a synaptogenic protein, in the spinal dorsal horn and facilitates the formation of newly immature dendritic spines, and triggers the initiation of neuropathic pain [Citation98]. SrGAP3 deactivates Rho GTPase (Rac1).
Paclitaxel increases expression of 5-HT1a and β2-adrenergic receptors (β2-AR) in the DRG [Citation99,Citation100], whereas serotonin and noradrenaline reuptake inhibitors relieve paclitaxel-induced pain via spinal 5-HT1, 5-HT2, and 5HT-3 receptor subtypes and spinal α2A-adrenergic receptors (α2A-AR), respectively [Citation100,Citation101]. These results indicate that serotonergic and noradrenergic modulation of spinal nociceptive transmission is altered in paclitaxel-induced neuropathic pain.
Paclitaxel increases the expression of the neuropeptide substance P in the dorsal horn of the spinal cord [Citation60,Citation102]. Paclitaxel also induces mechanical hypersensitivity by increasing spinal excitatory synaptic transmission through activating sigma-1 receptors (σ1R) and lysophosphatidic acid (LPA) receptors in the dorsal horn of the spinal cord [Citation103,Citation104]. However, these findings were contrasted by findings that antagonizing σ1R does not inhibit paclitaxel-induced neuropathy in rats [Citation105]. Furthermore, increased activity of the cation-chloride cotransporter Na+-K+-2Cl- cotransporter-1 (NKCC1) in the spinal cord may contribute to diminished spinal synaptic inhibition in paclitaxel-induced neuropathic pain [Citation106]. Finally, paclitaxel induces changes in endogenous kappa opioid receptor (KOR) agonist levels and opioid receptor signaling that are time-dependent, indicating that the initial aversive effects of paclitaxel treatment in mice, rather than hypersensitivity, is mediated by dysregulated KOR signaling [Citation107].
In addition, cannabinoid type 1 (CB1) receptor, δ-opioid receptor (DOR) protein levels, and their CB1R-DOR heteromers are increased in the spinal cord and basolateral amygdala of paclitaxel-treated mice. Mice exhibiting this increase show pain inhibition, suggesting that dysfunction of these receptors may contribute to paclitaxel-induced pain [Citation108]. Other studies indicate that CB2-agonists inhibit microglia and paclitaxel-induced neuropathic pain, suggesting that microglia contribute to the development of peripheral neuropathy in paclitaxel-treated mice [Citation109–112]. Further evidence shows that endogenous cannabinoids, including N-arachidonoylethanolamine (AEA, anandamide), produce antinociceptive effects via activation of CB1- and CB2 receptors. Paclitaxel significantly reduces levels of the endogenous cannabinoid 2-arachidonoylglycerol (2-AG) in the paw skin, suggesting that paclitaxel interferes with the endocannabinoid system, contributing to pain [Citation113].
Synaptic transmission in the brain
Paclitaxel administration impacts brain areas involved in the emotional and motivational response to pain, including areas involved in the mesocorticolimbic dopaminergic system [Citation114]. Paclitaxel affects the reorganization of pain neural circuitry, particularly the connections to the periaqueductal gray, to modulate pain perception [Citation114].
Immune activation
Activation of toll-like receptor pathways
TLR4 is a transmembrane protein, member of the toll-like receptor family that belongs to the pattern recognition receptor (PRR) and engages the same signaling pathway as the pro-inflammatory agent lipopolysaccharide (LPS) [Citation10]. TLR4 is expressed by immune cells but also sensory neurons. Importantly, TLR4 signaling has been implicated in the development of paclitaxel-induced peripheral neuropathy, because TLR4 antagonists block the development of paclitaxel-induced pain behaviors [Citation10,Citation11,Citation115].
Expression of components required for the TLR4 signal machinery (e.g., myeloid-differentiation response gene 88 (MyD88) and TIR-domain-containing adapter-inducing interferon-β (TRIF)) is increased in DRGs of paclitaxel-treated rodents [Citation11,Citation61]. In further support of the involvement of TLR4 signaling, inhibitors of MyD88 or mitogen-activated protein kinases (MAPK), two downstream signaling molecules of TLR4, prevent or reverse paclitaxel-induced behavioral hypersensitivity [Citation10,Citation11].
Besides TLR4, other TLR signaling pathways may be involved in paclitaxel-induced peripheral neuropathy. For example, a sex-dimorphic role of TLR9 is indicated in driving neuropathic pain [Citation116]. Male TLR9 mutant mice exhibit the delayed onset of paclitaxel-induced peripheral neuropathy and the administration of TLR9 inhibitors alleviates mechanical allodynia in male mice. Interestingly, TLR9 inhibition does not affect DRG infiltration of macrophages, suggesting that the receptor is not required for this process.
Immune cells
Macrophages
Various immune cells contribute to paclitaxel-induced peripheral neuropathy. For example, paclitaxel promotes macrophage accumulation in the DRG [Citation24,Citation28,Citation117], a process that requires expression of matrix metalloproteinase-3 (MMP-3) [Citation118] or the release of chemotactic factors (i.e., CCL2 and CX3CL1) by sensory neurons [Citation12,Citation117,Citation119]. Macrophages infiltrating the DRG during paclitaxel treatment have a pro-inflammatory M1 phenotype [Citation117]. Treatment of rats with losartan, an angiotensin II receptor type 1 (AT1R) blocker, alleviates mechanical allodynia, decreases the production of inflammatory mediators including CCL2 and reduces expression of M1 macrophage markers CD68, CCR2, iNOS and Nox2 in the DRG [Citation117,Citation120]. Simultaneously, losartan treatment results in an increase in PPARγ that is involved in M2 skewing [Citation117].
The exact mechanism of DRG macrophages contributing to the development and maintenance of paclitaxel-induced peripheral neuropathy remains unknown. Since macrophages express TLR4, paclitaxel may, directly and indirectly, activate TLR4. TLR4 activation could trigger cytoplasmic translocation and extracellular release of nuclear HMGB1 [Citation121]. HMGB1 in turn can further activate TLR4 signaling. Inhibiting HMGB1 release from macrophages prevents and reverses paclitaxel-induced neuropathy in mice [Citation121]. However, although direct TLR4 activation has been shown in rodents, it remains unknown whether paclitaxel also directly activates TLR4 in humans [Citation122].
T cells
T cells, in particular CD8+ T cells, are essential for recovery from paclitaxel-induced mechanical allodynia and are dependent on endogenous IL-10 signaling. Paclitaxel treatment increases the number of CD8+ T cells in DRG and inhibition of endogenous IL-10 signaling reconstituted with CD8+ T cells delayed recovery from paclitaxel-induced mechanical allodynia [Citation123]. On the contrary, administration of exogenous IL-10 attenuates paclitaxel-induced allodynia [Citation123].
Glial cells
Activation of spinal astrocytes also contributes to paclitaxel-induced peripheral neuropathy [Citation13,Citation124–129]. Spinal astrocytes regulate spinal synaptic transmission by modulating the uptake of the excitatory neurotransmitter glutamate from the synaptic cleft, with a reduction in transporters leading to increased glutamate levels at active synapses [Citation13,Citation122]. The majority of studies have demonstrated that spinal glutamate transporters, including GLAST and GLT-1, are rapidly downregulated by paclitaxel, possibly through activation of glycogen synthase kinase 3beta (GSK3 β) in the dorsal horn of the spinal cord [Citation13,Citation115,Citation122,Citation130–132]. Astrocyte activation following paclitaxel treatment may also occur in the anterior cingulate cortex (ACC), a brain region involved in pain perception and its modulation [Citation133]. Despite signs of astrocyte activation in the ACC, no changes were observed in glutamate transporter expression [Citation133].
Astrocytes treated with paclitaxel in vitro that are injected intrathecally into naïve mice induce mechanical allodynia [Citation129]. Inhibitors of astrocyte activation (L-α-AA), JNK or ERK, or neutralizing antibodies against TNF or SDF-1, alleviate paclitaxel-induced mechanical allodynia [Citation129], indicating a role of astrocytes and possible cytokines released by these cells.
Some evidence indicates that microglia may also be involved in the initiation of paclitaxel-induced peripheral neuropathy [Citation134], yet the majority of data points to astrocytes. Paclitaxel treatment induces signs of activation of microglia in the dorsal horn of the spinal cord including increased expression of CD86 [Citation135] and microglia-specific marker TMEM119 [Citation128]. Increased numbers of microglia in the spinal cord of paclitaxel-treated mice are accompanied by an increase in CCL3 [Citation136].
Other glial cells, such as satellite glial cells that support and surround DRG neuronal cell bodies, affect paclitaxel-induced peripheral neuropathy. For example, in paclitaxel-treated rats, satellite glial cells become hypertrophic and express ATF3 [Citation34,Citation35]. However, the exact contribution of these cells to paclitaxel-induced neuropathy is poorly studied.
Inflammatory mediators
Activation of the pro-inflammatory transcription factor NF-κB is reported in multiple studies, which further leads to the production of pro-inflammatory mediators [Citation41,Citation50,Citation126,Citation128,Citation137–140]. Many studies have found increased expression of various inflammatory mediators in the spinal cord which could be the result of astrocyte activation or other glia cell or infiltrating immune cells, including TNF [Citation32,Citation41,Citation120,Citation124–126,Citation128,Citation129,Citation135,Citation139–143], IFN-γ [Citation124,Citation135,Citation142], IL-1β [Citation32,Citation50,Citation55,Citation120,Citation125,Citation126,Citation128,Citation135,Citation139–144], TGF-β [Citation135] and stromal-derived cell factor 1 (SDF-1) [Citation129]. Two studies, however, did not observe any changes in pro-inflammatory cytokines [Citation13,Citation145].
Several other cytokines have recently been identified as contributors to paclitaxel-induced peripheral neuropathy. IL-17 is elevated in the spinal cord and cerebrospinal fluid of mice following paclitaxel treatment and IL-17 increases NMDAR activity and suppresses GABA-induced currents in the spinal cord [Citation146]. Blockade of IL-17R antibodies or knockdown of IL-17R reduces paclitaxel-induced pain [Citation146] and the same goes for blockade of IL-20 using monoclonal antibodies [Citation147]. IL-20 activates astrocytes, triggering the production of pro-inflammatory mediators in the DRG, including IL-8 and MCP-1. IL-20 also recruits macrophages to the DRG, partly through the MAPK pathway and modulates neurite outgrowth by disturbing intracellular Ca2+ balance [Citation147]. Furthermore, serum levels of IL-6 are elevated in paclitaxel-treated mice and administration of an IL-6 blocking antibody prevents mechanical allodynia and nerve damage [Citation117,Citation148]. Paclitaxel activates the PI3K/Akt pathway and inhibition of PI3K/Akt alleviates mechanical allodynia in paclitaxel-treated rats [Citation149].
The downstream production of MMP-9 is elevated in the DRG of paclitaxel-treated mice and inhibiting MMP-9 prevents and reverses mechanical allodynia. In particular, neutralizing antibodies against MMP-9 reduce oxidative stress and TNF-α and IL-6 levels in the DRG, indicating a key role for MMP-9 in paclitaxel-induced inflammation [Citation150].
Chemokines that have been studied for their contribution to paclitaxel-induced peripheral neuropathy include CCL3 (50,118,133), CCL2 (143), CX3CL1 (113,144,145), CXCL12 (146) and CXCL1 (147). CCL2/CCR2 signaling is involved in both the induction and maintenance of paclitaxel-induced behavioral hypersensitivity, since blockade of CCL2/CCR2 signaling prevents and reverses paclitaxel-induced hypersensitivity [Citation151]. Similarly, CX3CL1 expression is upregulated in the DRG in a time course consistent with the behavioral phenotype of paclitaxel-induced pain and blocking CX3CL1 prevents paclitaxel-induced DRG macrophage infiltration and cytokine release and attenuates paclitaxel-induced pain [Citation119,Citation152]. The receptor for CX3CL1, CX3CR1 increases in DRG and spinal cord after paclitaxel treatment [Citation153]. CX3CL1-CX3CR1 signaling contributes to paclitaxel-induced peripheral neuropathy through activating Akt2 in spinal dorsal horn microglia [Citation153] and p38 MAPK [Citation119] in microglia and DRG macrophages. Furthermore, paclitaxel treatment increases CXCL12 in the spinal cord [Citation154] and CXCL1 in the DRG and spinal cord [Citation155]. CXCL12 and CXCL1 signal through CXCR4 and CXCR2 and blocking these receptors reduces paclitaxel-induced mechanical hypersensitivity [Citation154,Citation155].
Finally, bradykinin, an inflammatory mediator, has been linked with paclitaxel-induced pain due to the analgesic effect of NSAIDs [Citation156]. Indeed, the expression of B2 mRNA (Bdkrb2) in the sciatic nerve, but not B1 mRNA (Bdkrb1) is increased after paclitaxel administration [Citation156].
Other neuro-inflammatory processes
Paclitaxel activates the sphingolipid pathway, resulting in the production of ceramide, a lipid that induces apoptosis in a variety of neoplasms. Increased ceramide production results from increased activity of ceramide hydrolyzing enzymes, which is associated with the development of neuropathic pain after paclitaxel treatment [Citation137].
The Wnt/β catenin pathway is also upregulated in the sciatic nerves of paclitaxel-treated rats. Wnt signaling inhibitors reduce the loss of IENF, improve nerve dysfunction and alleviate mechanical allodynia, suggesting a role of this pathway in paclitaxel-induced peripheral neuropathy [Citation157].
Discussion
This systematic review highlights various underlying mechanisms of paclitaxel-induced peripheral neuropathy. The most recent literature shows that inflammatory responses in neuronal tissue, including immune cell accumulation and cytokine/chemokine release, are important contributors to paclitaxel-induced peripheral neuropathy. Indeed, paclitaxel may directly activate TLR4 and its downstream pathways, causing macrophage and T cell accumulation in nervous tissue, and activate glial cells with numerous pro-inflammatory cytokines released from either DRG neurons or associated cells. Furthermore, paclitaxel induces mechanical hypersensitivity by increasing sensory neuron excitability and spinal transmission. As described in earlier reviews, axonal damage and mitochondrial dysfunction associated with oxidative stress seem central mechanisms in paclitaxel-induced peripheral neuropathy. It is possible that these mitochondrial-related mechanisms affect neuronal excitability but also drive neuro-inflammatory processes. However, how these processes may engage neuro-inflammatory processes remains elusive.
Despite a large number of identified molecular mechanisms, it is not well known how these various pathways interact and occur in time. Nevertheless, we hypothesize that central to the initiation of peripheral neuropathy is the effect of paclitaxel on mitochondrial function in nerve and supporting cells leading to nerve damage and thinning of myelin sheets surrounding peripheral nerve fibers. As a result, such damage may lead to infiltration of immune cells, as is observed in other models of neuropathy. Since paclitaxel also induces activation of TLR, this may affect the phenotype and number of immune cells in nervous tissue. Subsequently, the release of inflammatory pro-algesic mediators (e.g., TNF, IL-17, IL-20, CCL2) is promoted, affecting neuronal function through modulating expression and activity of ion channels in sensory neurons and receptors in the spinal cord. Importantly, future studies should also be aimed at understanding these dependencies and sequences in events to aid in determining at what point of these cascades therapeutic intervention would be most promising.
In this systematic review, we identified some discrepancies between findings on molecular mechanisms of paclitaxel-induced peripheral neuropathy. This might be due to the sex of animals, the dose and dosing schedule of paclitaxel administration, the mode of administration (intravenous or intraperitoneal), or the timing of measurement. For example, whilst recent findings have shown intriguing sex differences in the various models of chronic pain [Citation158], only a handful of studies using both male and female mice found disparate results depending on sex [Citation116,Citation134]. Furthermore, paclitaxel does not consistently induce signs of microglia activation in the spinal cord and most studies that showed involvement of microglia in paclitaxel-induced peripheral neuropathy used a cumulative dose of paclitaxel up to 36 mg/kg [Citation35,Citation134,Citation136] instead of 8 mg/kg as almost half of the included studies used. Although in the lower range, the latter does is clinically relevant, because it is equal to 56.24 mg/m2 and the dose of paclitaxel used in cancer patients ranges from 15 mg/m2 to 825 mg/m2 [Citation122]. We hypothesize that the use of higher doses of paclitaxel would be useful to more closely approach the threshold dose for the development of paclitaxel-induced peripheral neuropathy in humans, which lies approximately at 300 mg/m2 [Citation6].
Limitations
The reporting of the methodology of the experimental animal studies included in this systematic review is typically poor as highlighted by the results of the risk of bias assessment since many items were indicated as ‘unclear’ risk of bias. This issue has been previously reported [Citation159] and future studies, using animals, should improve both the experimental design and the quality of reporting by using the Design and Execution of Protocols for Animal Research and Treatment (DEPART) and Animals in Research: Reporting In Vivo Experiments (ARRIVE) guidelines to increase scientific validity of the results.
Second, the preclinical studies included in this systematic review do not fully match the clinical scenario, since paclitaxel is administered in the absence of a tumor, thus not taking the complex interaction between the tumor and chemotherapeutic agent into consideration. However, for example, nicotinamide riboside prevents paclitaxel-induced hypersensitivity and this effect was similar in both tumor-naïve and tumor-bearing rats [Citation31]. In addition, cancer patients often undergo chemotherapy after surgical removal of the tumor [Citation7] and therefore modeling chemotherapy-induced peripheral neuropathy through chemotherapy administration alone is a valid approach.
Third, pain outcome measurement in rodent models encompasses mostly stimulus-evoked (responses to e.g., mechanical stimuli) and non-stimulus evoked (spontaneous) behaviors [Citation18]. Rodent models of paclitaxel-induced peripheral neuropathy have elucidated pathophysiological mechanisms and identified potential drug targets. However, the question of translatability to paclitaxel-induced peripheral neuropathy in humans remains a topic of continued scrutiny.
Clinical relevance
A recent review of the pharmacological treatment of neuropathic pain provides evidence-based recommendations for its pharmacological treatment [Citation160]. First-line drugs currently recommended in peripheral neuropathic pain include gabapentin and pregabalin. Their analgesic effects are mainly related to the normalization of the paclitaxel-induced increased expression of voltage-dependent calcium channels that contain the alpha-2-delta type 1 subunit [Citation87,Citation88]. Although results from preclinical studies provide a valuable model to examine molecular pathways and test potential preventive and therapeutic targets, future well-designed clinical trials are needed to confirm these proposed pathways in humans to develop effective treatment strategies.
Exercise may have a promising role to prevent or mitigate pain as emerging data is indicating that exercise favorably alter the inflammatory environment. Data on inflammatory responses to exercise in patients with cancer during adjuvant chemotherapy tend to show that exercise decreases pro-inflammatory markers such as TNF-α, IL-1β and IFN-γ and increases anti-inflammatory markers such as IL-10 [Citation161–163]. It might be suggested that the anti-inflammatory effects of exercise partially explain the beneficial effect of exercise on pain in patients with cancer. Indeed, results from several randomized controlled trials point out a promising role for aerobic and resistance exercise, either separately or in combination, to improve pain in patients with breast cancer receiving adjuvant chemotherapy [Citation164–167]. For example, Mijwel et al. (2018) found that sixteen weeks of resistance with high-intensity interval training (RT-HIIT) significantly improved pain sensitivity in these patients [Citation167]. It will be of interest to see how these interventions affect neuro-immune processes or mitochondrial health during chemotherapy.
Conclusion
Many groups have begun to uncover potential molecular mechanisms by which paclitaxel causes peripheral neuropathy and this systematic review provides an overview of these mechanisms, including paclitaxel-induced inflammatory responses with numerous pro-inflammatory cytokines released from either DRG neurons or associated cells. Future studies are needed to investigate whether the same molecular mechanisms underlying paclitaxel-induced peripheral neuropathy are involved in humans in order to develop targeted preventive and therapeutic strategies.
Supplemental Material
Download MS Word (54.1 KB)Supplemental Material
Download MS Word (65.9 KB)Supplemental Material
Download MS Word (15.4 KB)Disclosure statement
No potential conflict of interest was reported by the author(s).
References
- Harbeck N, Gnant M. Breast cancer. Lancet. 2017;389(10074):1134–1150.
- Velasco R, Bruna J. Taxane-induced peripheral neurotoxicity. Toxics. 2015;3(2):152–169.
- Kerckhove N, CA, Condé S, Chaleteix C, et al. Long-term effects, pathophysiological mechanisms, and risk factors of chemotherapy-induced peripheral neuropathies: a comprehensive literature review. Front Pharmacol. 2017;8:86.
- Argyriou AA, Koltzenburg M, Polychronopoulos P, et al. Peripheral nerve damage associated with administration of taxanes in patients with cancer. Crit Rev Oncol Hematol. 2008;66(3):218–228.
- Zajaczkowska R, Kocot-Kepska M, Leppert W, et al. Mechanisms of chemotherapy-induced peripheral neuropathy. Int J Mol Sci. 2019;20(6):1451.
- Starobova H, Vetter I. Pathophysiology of chemotherapy-induced peripheral neuropathy. Front Mol Neurosci. 2017;10:174.
- Flatters SJL, Dougherty PM, Colvin LA. Clinical and preclinical perspectives on chemotherapy-induced peripheral neuropathy (CIPN): a narrative review. Br J Anaesth. 2017;119(4):737–749.
- Reeves BN, Dakhil SR, Sloan JA, et al. Further data supporting that paclitaxel-associated acute pain syndrome is associated with development of peripheral neuropathy: North Central Cancer Treatment Group trial N08C1. Cancer. 2012;118(20):5171–5178.
- De Iuliis F, Taglieri L, Salerno G, et al. Taxane induced neuropathy in patients affected by breast cancer: literature review. Crit Rev Oncol Hematol. 2015;96(1):34–45.
- Li Y, Zhang H, Zhang H, et al. Toll-like receptor 4 signaling contributes to paclitaxel-induced peripheral neuropathy. J Pain. 2014;15(7):712–725.
- Li Y, Zhang H, Kosturakis AK, et al. MAPK signaling downstream to TLR4 contributes to paclitaxel-induced peripheral neuropathy. Brain Behav Immun. 2015;49:255–266.
- Zhang H, Li Y, de Carvalho-Barbosa M, et al. dorsal root ganglion infiltration by macrophages contributes to paclitaxel chemotherapy-induced peripheral neuropathy. J Pain. 2016;17(7):775–786.
- Zhang H, Yoon S-Y, Zhang H, et al. Evidence that spinal astrocytes but not microglia contribute to the pathogenesis of paclitaxel-induced painful neuropathy. J Pain. 2012;13(3):293–303.
- Staff NP, Fehrenbacher JC, Caillaud M, et al. Pathogenesis of paclitaxel-induced peripheral neuropathy: a current review of in vitro and in vivo findings using rodent and human model systems. Exp Neurol. 2020;324:113121.
- Ouzzani M, Hammady H, Fedorowicz Z, et al. Rayyan—a web and mobile app for systemic reviews. Syst Rev. 2016;5(1)210.
- Hooijmans CR, Rovers MM, De Vries RBM, et al. SYRCLE’s risk of bias tool for animal studies. BMC Med Res Methodol. 2014;14(1):1–9.
- Higgins JPT, Altman DG, Gøtzsche PC, et al. The Cochrane collaboration’s tool for assessing risk of bias in randomised trials. Br Med J. 2011;343:889–893.
- Deuis JR, Dvorakova LS, Vetter I. Methods used to evaluate pain behaviors in rodents. Front Mol Neurosci. 2017;10:284.
- Boehmerle W, Huehnchen P, Peruzzaro S, et al. Electrophysiological, behavioral and histological characterization of paclitaxel, cisplatin, vincristine and bortezomib-induced neuropathy in C57Bl/6 mice. Sci Rep. 2014;4:6370.
- Benbow SJ, Cook BM, Reifert J, et al. Effects of paclitaxel and eribulin in mouse sciatic nerve: a microtubule-based rationale for the differential induction of chemotherapy-induced peripheral neuropathy. Neurotox Res. 2016;29(2):299–313.
- Tasnim A, Rammelkamp Z, Slusher AB, et al. Paclitaxel causes degeneration of both central and peripheral axon branches of dorsal root ganglia in mice. BMC Neurosci. 2016;17(1):47.
- Cook BM, Wozniak KM, Proctor DA, et al. Differential morphological and biochemical recovery from chemotherapy-induced peripheral neuropathy following paclitaxel, ixabepilone, or eribulin treatment in mouse sciatic nerves. Neurotox Res. 2018;34(3):677–692.
- Wu Y, Li J, Zhou J, et al. Dynamic long-term microstructural and ultrastructural alterations in sensory nerves of rats of paclitaxel-induced neuropathic pain. Chin Med J. 2014;127(16):2945–2952.
- Beh ST, Kuo YM, Chang WSW, et al. Preventive hypothermia as a neuroprotective strategy for paclitaxel-induced peripheral neuropathy. Pain. 2019;160(7):1505–1521.
- Jamieson SMF, Liu JJ, Connor B, et al. Nucleolar enlargement, nuclear eccentricity and altered cell body immunostaining characteristics of large-sized sensory neurons following treatment of rats with paclitaxel. Neurotoxicology. 2007;28(6):1092–1098.
- Wozniak KM, Vornov JJ, Wu Y, et al. Peripheral neuropathy induced by microtubule-targeted chemotherapies: insights into acute injury and long-term recovery. Cancer Res. 2018;78(3):817–829.
- Chine VB, Au NPB, Kumar G, et al. Targeting axon integrity to prevent chemotherapy-induced peripheral neuropathy. Mol Neurobiol. 2019;56(5):3244–3259.
- Liu C-C, Lu N, Cui Y, et al. Prevention of paclitaxel-induced allodynia by minocycline: effect on loss of peripheral nerve fibers and infiltration of macrophages in rats. Mol Pain. 2010;6:76.
- Ko M-H, Hu M-E, Hsieh Y-L, et al. Peptidergic intraepidermal nerve fibers in the skin contribute to the neuropathic pain in paclitaxel-induced peripheral neuropathy. Neuropeptides. 2014;48(3):109–117.
- Siau C, Xiao W, Bennett GJ. Paclitaxel- and vincristine-evoked painful peripheral neuropathies: loss of epidermal innervation and activation of Langerhans cells. Exp Neurol. 2006;201(2):507–514.
- Hamity MV, White SR, Blum C, et al. Nicotinamide riboside relieves paclitaxel-induced peripheral neuropathy and enhances suppression of tumor growth in tumor-bearing rats. Pain. 2020;161(10):2364–2375.
- Wu P, Chen Y. Evodiamine ameliorates paclitaxel-induced neuropathic pain by inhibiting inflammation and maintaining mitochondrial anti-oxidant functions. Hum Cell. 2019;32(3):251–259.
- Bobylev I, Joshi AR, Barham M, et al. Paclitaxel inhibits mRNA transport in axons. Neurobiol Dis. 2015;82:321–331.
- Jimenez-Andrade JM, Peters CM, Mejia NA, et al. Sensory neurons and their supporting cells located in the trigeminal, thoracic and lumbar ganglia differentially express markers of injury following intravenous administration of paclitaxel in the rat. Neurosci Lett. 2006;405(1–2):62–67.
- Peters CM, Jimenez-Andrade JM, Jonas BM, et al. Intravenous paclitaxel administration in the rat induces a peripheral sensory neuropathy characterized by macrophage infiltration and injury to sensory neurons and their supporting cells. Exp Neurol. 2007;203(1):42–54.
- Choi SS, Koh WU, Nam JS, et al. Effect of ethyl pyruvate on paclitaxel-induced neuropathic pain in rats. Korean J Pain. 2013;26(2):135–141.
- Flatters SJL, Bennett GJ. Studies of peripheral sensory nerves in paclitaxel-induced painful peripheral neuropathy: evidence for mitochondrial dysfunction. Pain. 2006;122(3):245–257.
- Slivicki RA, Ali YO, Lu H-C, et al. Impact of genetic reduction of NMNAT2 on chemotherapy-induced losses in cell viability in vitro and peripheral neuropathy in vivo. PLoS One. 2016;11(1):e0147620.
- Nakahashi Y, Kamiya Y, Funakoshi K, et al. Role of nerve growth factor-tyrosine kinase receptor A signaling in paclitaxel-induced peripheral neuropathy in rats. Biochem Biophys Res Commun. 2014;444(3):415–419.
- Arrieta Ó, Hernández-Pedro N, Fernández-González-Aragón MC, et al. Retinoic acid reduces chemotherapy-induced neuropathy in an animal model and patients with lung cancer. Neurology. 2011;77(10):987–995.
- Al-Massri KF, Ahmed LA, El-Abhar HS, et al. Pregabalin and lacosamide ameliorate paclitaxel-induced peripheral neuropathy via inhibition of JAK/STAT signaling pathway and Notch-1 receptor. Neurochem Int. 2018;120:164–171.
- Jia M, Wu C, Gao F, et al. Activation of NLRP3 inflammasome in peripheral nerve contributes to paclitaxel-induced neuropathic pain. Mol Pain. 2017;13:1744806917719804.
- Nieto FR, Cendan CM, Canizares FJ, et al. Genetic inactivation and pharmacological blockade of sigma-1 receptors prevent paclitaxel-induced sensory-nerve mitochondrial abnormalities and neuropathic pain in mice. Mol Pain. 2014;10:11.
- Zheng H, Xiao WH, Bennett GJ. Functional deficits in peripheral nerve mitochondria in rats with paclitaxel- and oxaliplatin-evoked painful peripheral neuropathy. Exp Neurol. 2011;232(2):154–161.
- Janes K, Doyle T, Bryant L, et al. Bioenergetic deficits in peripheral nerve sensory axons during chemotherapy-induced neuropathic pain resulting from peroxynitrite-mediated post-translational nitration of mitochondrial superoxide dismutase. Pain. 2013;154(11):2432–2440.
- Braga AV, Costa SOAM, Rodrigues FF, et al. Thiamine, riboflavin, and nicotinamide inhibit paclitaxel-induced allodynia by reducing TNF-α and CXCL-1 in dorsal root ganglia and thalamus and activating ATP-sensitive potassium channels. Inflammopharmacology. 2020;28(1):201–213.
- Li X, Yang S, Wang L, et al. Resveratrol inhibits paclitaxel-induced neuropathic pain by the activation of PI3K/Akt and SIRT1/PGC1alpha pathway. JPR. 2019;12:879–890.
- Yamashita Y, Irie K, Kochi A, et al. Involvement of Charcot-Marie-Tooth disease gene mitofusin 2 expression in paclitaxel-induced mechanical allodynia in rats. Neurosci Lett. 2017;653:337–340.
- Duggett NA, Griffiths LA, McKenna OE, et al. Oxidative stress in the development, maintenance and resolution of paclitaxel-induced painful neuropathy. Neuroscience. 2016;333:13–26.
- Kim HK, Hwang S-H, Abdi S. Tempol ameliorates and prevents mechanical hyperalgesia in a rat model of chemotherapy-induced neuropathic pain. Front Pharmacol. 2016;7:532.
- Miao H, Xu J, Xu D, et al. Nociceptive behavior induced by chemotherapeutic paclitaxel and beneficial role of antioxidative pathways. Physiol Res. 2019;68(3);491–500.
- Lu Y, Zhang P, Zhang Q, et al. duloxetine attenuates paclitaxel-induced peripheral nerve injury by inhibiting p53-related pathways. J Pharmacol Exp Ther. 2020;373(3):453–462.
- Zhao X, Liu L, Wang Y, et al. Electroacupuncture enhances antioxidative signal pathway and attenuates neuropathic pain induced by chemotherapeutic paclitaxel. Physiol Res. 2019;68(3):501–510.
- Chahal SK, Sodhi RK, Madan J. Duloxetine hydrochloride loaded film forming dermal gel enriched with methylcobalamin and geranium oil attenuates paclitaxel-induced peripheral neuropathy in rats. IBRO Rep. 2020;9:85–95.
- Yasar H, Ersoy A, Yazici GN, et al. The effect of lutein on paclitaxel-induced neuropathy and neuropathic pain in rats. Int J Clin Exp Med. 2020;13(6):4316–4323.
- Sun H, Guo X, Wang Z, et al. Alphalipoic acid prevents oxidative stress and peripheral neuropathy in nab-paclitaxel-treated rats through the Nrf2 signalling pathway. Oxid Med Cell Longev. 2019;2019:1–11.
- Singh J, Saha L, Singh N, et al. Study of nuclear factor-2 erythroid related factor-2 activator, berberine, in paclitaxel induced peripheral neuropathy pain model in rats. J Pharm Pharmacol. 2019;71(5):797–805.
- Kaur S, Muthuraman A. Ameliorative effect of gallic acid in paclitaxel-induced neuropathic pain in mice. Toxicol Rep. 2019;6:505–513.
- Hara T, Chiba T, Abe K, et al. Effect of paclitaxel on transient receptor potential vanilloid 1 in rat dorsal root ganglion. Pain. 2013;154(6):882–889.
- Wu Y, Chen J, Wang R. Puerarin suppresses TRPV1, calcitonin gene-related peptide and substance P to prevent paclitaxel-induced peripheral neuropathic pain in rats. Neuroreport. 2019;30(4):288–294.
- Li Y, Yin C, Li X, et al. Electroacupuncture alleviates paclitaxel-induced peripheral neuropathic pain in rats via suppressing TLR4 signaling and TRPV1 upregulation in sensory neurons. Int J Mol Sci. 2019;20(23):5917.
- Kamata Y, Kambe T, Chiba T, et al. Paclitaxel induces upregulation of transient receptor potential vanilloid 1 expression in the rat spinal cord. Int J Mol Sci. 2020;21(12):4341.
- Li Y, Adamek P, Zhang H, et al. The cancer chemotherapeutic paclitaxel increases human and rodent sensory neuron responses to TRPV1 by activation of TLR4. J Neurosci. 2015;35(39):13487–13500.
- Rossato MF, Rigo FK, Oliveira SM, et al. Participation of transient receptor potential vanilloid 1 in paclitaxel-induced acute visceral and peripheral nociception in rodents. Eur J Pharmacol. 2018;828:42–51.
- Chen Y, Yang C, Wang ZJ. Proteinase-activated receptor 2 sensitizes transient receptor potential vanilloid 1, transient receptor potential vanilloid 4, and transient receptor potential ankyrin 1 in paclitaxel-induced neuropathic pain. Neuroscience. 2011;193:440–451.
- Alessandri-Haber N, Dina OA, Joseph EK, et al. Interaction of transient receptor potential vanilloid 4, integrin, and SRC tyrosine kinase in mechanical hyperalgesia. J Neurosci. 2008;28(5):1046–1057.
- Alessandri-Haber N, Dina OA, Yeh JJ, et al. Transient receptor potential vanilloid 4 is essential in chemotherapy-induced neuropathic pain in the rat. J Neurosci. 2004;24(18):4444–4452.
- Boehmerle W, Huehnchen P, Lee SLL, et al. TRPV4 inhibition prevents paclitaxel-induced neurotoxicity in preclinical models. Exp Neurol. 2018;306:64–75.
- Materazzi S, Fusi C, Benemei S, et al. TRPA1 and TRPV4 mediate paclitaxel-induced peripheral neuropathy in mice via a glutathione-sensitive mechanism. Pflugers Arch. 2012;463(4):561–569.
- Sałat K, Filipek B. Antinociceptive activity of transient receptor potential channel TRPV1, TRPA1, and TRPM8 antagonists in neurogenic and neuropathic pain models in mice. J Zhejiang Univ Sci B. 2015;16(3):167–178.
- Zhang X-Z, Luo D-X, Bai X-H, et al. Upregulation of TRPC6 Mediated by PAX6 Hypomethylation Is Involved in the Mechanical allodynia induced by chemotherapeutics in dorsal root ganglion. Int J Neuropsychopharmacol. 2020;23(4):257–267.
- Sisignano M, Angioni C, Park C-K, et al. Targeting CYP2J to reduce paclitaxel-induced peripheral neuropathic pain. Proc Natl Acad Sci USA. 2016;113(44):12544–12549.
- Wu Z, Wang S, Wu I, et al. Activation of TLR-4 to produce tumour necrosis factor-α in neuropathic pain caused by paclitaxel. Eur J Pain. 2015;19(7):889–898.
- Alvarez P, Ferrari LF, Levine JD. Muscle pain in models of chemotherapy-induced and alcohol-induced peripheral neuropathy. Ann Neurol. 2011;70(1):101–109.
- Dina OA, Chen X, Reichling D, et al. Role of protein kinase cepsilon and protein kinase A in a model of paclitaxel-induced painful peripheral neuropathy in the rat. Neuroscience. 2001;108(3):507–515.
- Nie B, Liu C, Bai X, et al. AKAP150 involved in paclitaxel-induced neuropathic pain via inhibiting CN/NFAT2 pathway and downregulating IL-4. Brain Behav Immun. 2018;68:158–168.
- Gao W, Zan Y, Wang Z-JJ, et al. Quercetin ameliorates paclitaxel-induced neuropathic pain by stabilizing mast cells, and subsequently blocking PKCε-dependent activation of TRPV1. Acta Pharmacol Sin. 2016;37(9):1166–1177.
- Costa R, Motta EM, Dutra RC, et al. Anti-nociceptive effect of kinin B(1) and B(2) receptor antagonists on peripheral neuropathy induced by paclitaxel in mice. Br J Pharmacol. 2011;164(2b):681–693.
- Costa R, Bicca MA, Manjavachi MN, et al. Kinin receptors sensitize TRPV4 channel and induce mechanical hyperalgesia: relevance to paclitaxel-induced peripheral neuropathy in mice. Mol Neurobiol. 2018;55(3):2150–2161.
- Li Y, Tatsui CE, Rhines LD, et al. Dorsal root ganglion neurons become hyperexcitable and increase expression of voltage-gated T-type calcium channels (Cav3.2) in paclitaxel-induced peripheral neuropathy. Pain. 2017;158(3):417–429.
- Li Y, North RY, Rhines LD, et al. DRG voltage-gated sodium channel 1.7 Is upregulated in paclitaxel-induced neuropathy in rats and in humans with neuropathic pain. J Neurosci. 2018;38(5):1124–1136.
- Xiao Y, Xia Z, Wu Y, et al. Sodium channel Nav1.7 expression is upregulated in the dorsal root ganglia in a rat model of paclitaxel-induced peripheral neuropathy. Springerplus. 2016;5(1):1738.
- Chandra S, Wang Z, Tao X, et al. Computer-aided discovery of a new Nav1.7 inhibitor for treatment of pain and itch. Anesthesiology. 2020;133(3):611–627.
- Liang X, Yu G, Su R. Effects of ralfinamide in models of nerve injury and chemotherapy-induced neuropathic pain. Eur J Pharmacol. 2018;823:27–34.
- Okubo K, Takahashi T, Sekiguchi F, et al. Inhibition of T-type calcium channels and hydrogen sulfide-forming enzyme reverses paclitaxel-evoked neuropathic hyperalgesia in rats. Neuroscience. 2011;188:148–156.
- Kawakami K, Chiba T, Katagiri N, et al. Paclitaxel increases high voltage-dependent calcium channel current in dorsal root ganglion neurons of the rat. J Pharmacol Sci. 2012;120(3):187–195.
- Gauchan P, Andoh T, Ikeda K, et al. Mechanical allodynia induced by paclitaxel, oxaliplatin and vincristine: different effectiveness of gabapentin and different expression of voltage-dependent calcium channel alpha(2)delta-1 subunit. Biol Pharm Bull. 2009;32(4):732–734.
- Xiao W, Boroujerdi A, Bennett GJ, et al. Chemotherapy-evoked painful peripheral neuropathy: analgesic effects of gabapentin and effects on expression of the alpha-2-delta type-1 calcium channel subunit. Neuroscience. 2007;144(2):714–720.
- Matsumoto M, Inoue M, Hald A, et al. Inhibition of paclitaxel-induced A-fiber hypersensitization by gabapentin. J Pharmacol Exp Ther. 2006;318(2):735–740.
- Mao Q, Wu S, Gu X, et al. DNMT3a-triggered downregulation of K2p 1.1 gene in primary sensory neurons contributes to paclitaxel-induced neuropathic pain. Int J Cancer. 2019;145(8):2122–2134.
- Li L, Li J, Zuo Y, et al. Activation of KCNQ channels prevents paclitaxel-induced peripheral neuropathy and associated neuropathic pain. J Pain. 2019;20(5):528–539.
- Masocha W. Gene expression profile of sodium channel subunits in the anterior cingulate cortex during experimental paclitaxel-induced neuropathic pain in mice. PeerJ. 2016;4:e2702.
- Megat S, Ray PR, Moy JK, et al. Nociceptor translational profiling reveals the ragulator-rag GTPase complex as a critical generator of neuropathic pain. J Neurosci. 2019;39(3):393–411.
- Chen Y, Chen S-R, Chen H, et al. Increased α2δ-1-NMDA receptor coupling potentiates glutamatergic input to spinal dorsal horn neurons in chemotherapy-induced neuropathic pain. J Neurochem. 2019;148(2):252–274.
- Xie J-D, Chen S-R, Chen H, et al. Presynaptic N-methyl-D-aspartate (NMDA) receptor activity is increased through protein kinase C in paclitaxel-induced neuropathic pain. J Biol Chem. 2016;291(37):19364–19373.
- Xie J-D, Chen S-R, Pan H-L. Presynaptic mGluR5 receptor controls glutamatergic input through protein kinase C-NMDA receptors in paclitaxel-induced neuropathic pain. J Biol Chem. 2017;292(50):20644–20654.
- Wang X-M, Gu P, Saligan L, et al. Dysregulation of EAAT2 and VGLUT2 spinal glutamate transports via histone deacetylase 2 (HDAC2) contributes to paclitaxel-induced painful neuropathy. Mol Cancer Ther. 2020;19(10):2196–2209.
- Chen Z, Zhang S, Nie B, et al. Distinct roles of srGAP3-Rac1 in the initiation and maintenance phases of neuropathic pain induced by paclitaxel. J Physiol. 2020;598(12):2415–2430.
- Andoh T, Sakamoto A, Kuraishi Y. Effects of xaliproden, a 5-HT(1)A agonist, on mechanical allodynia caused by chemotherapeutic agents in mice. Eur J Pharmacol. 2013;721(1–3):231–236.
- Costa-Pereira JT, Ribeiro J, Martins I, et al. Role of spinal cord α2-adrenoreceptors in noradrenergic inhibition of nociceptive transmission during chemotherapy-induced peripheral neuropathy. Front Neurosci. 2020;13:1413. [Internet] Available from: https://www.embase.com/search/results?subaction=viewrecord&id=L630727665&from=export
- Li D, Yoo JH, Kim SK. Long-lasting and additive analgesic effects of combined treatment of bee venom acupuncture and venlafaxine on paclitaxel-induced allodynia in mice. Toxins. 2020;12(10):620.
- Chiba T, Oka Y, Kambe T, et al. Paclitaxel-induced peripheral neuropathy increases substance P release in rat spinal cord. Eur J Pharmacol. 2016;770:46–51.
- Nieto FR, Cendan CM, Sanchez-Fernandez C, et al. Role of sigma-1 receptors in paclitaxel-induced neuropathic pain in mice. J Pain. 2012;13(11):1107–1121.
- Uchida H, Nagai J, Ueda H. Lysophosphatidic acid and its receptors LPA1 and LPA3 mediate paclitaxel-induced neuropathic pain in mice. Mol Pain. 2014;10:71.
- Tomohisa M, Junpei O, Aki M, et al. Possible involvement of the sigma-1 receptor chaperone in chemotherapeutic-induced neuropathic pain. Synapse. 2015;69(11):526–532.
- Chen S-R, Zhu L, Chen H, et al. Increased spinal cord Na(+)-K(+)-2Cl(−) cotransporter-1 (NKCC1) activity contributes to impairment of synaptic inhibition in paclitaxel-induced neuropathic pain. J Biol Chem. 2014;289(45):31111–31120.
- Meade JA, Alkhlaif Y, Contreras KM, et al. Kappa opioid receptors mediate an initial aversive component of paclitaxel-induced neuropathy. Psychopharmacology. 2020;237(9):2777–2793.
- Sierra S, Gupta A, Gomes I, et al. Targeting cannabinoid 1 and delta opioid receptor heteromers alleviates chemotherapy-induced neuropathic pain. ACS Pharmacol Transl Sci. 2019;2(4):219–229.
- Segat GC, Manjavachi MN, Matias DO, et al. Antiallodynic effect of beta-caryophyllene on paclitaxel-induced peripheral neuropathy in mice. Neuropharmacology. 2017;125:207–219.
- Deng L, Guindon J, Vemuri VK, et al. The maintenance of cisplatin- and paclitaxel-induced mechanical and cold allodynia is suppressed by cannabinoid CB(2) receptor activation and independent of CXCR4 signaling in models of chemotherapy-induced peripheral neuropathy. Mol Pain. 2012;8:71.
- Wu J, Hocevar M, Bie B, et al. Cannabinoid type 2 receptor system modulates paclitaxel-induced microglial dysregulation and central sensitization in rats. J Pain. 2019;20(5):501–514.
- Naguib M, Xu JJ, Diaz P, et al. Prevention of paclitaxel-induced neuropathy through activation of the central cannabinoid type 2 receptor system. Anesth Analg. 2012;114(5):1104–1120.
- Thomas A, Okine BN, Finn DP, et al. Peripheral deficiency and antiallodynic effects of 2-arachidonoyl glycerol in a mouse model of paclitaxel-induced neuropathic pain. Biomed Pharmacother. 2020;129:110456.
- Ferris CF, Nodine S, Pottala T, et al. Alterations in brain neurocircuitry following treatment with the chemotherapeutic agent paclitaxel in rats. Neurobiol Pain. 2019;6:100034.
- Xu Y, Cheng G, Zhu Y, et al. Anti-nociceptive roles of the glia-specific metabolic inhibitor fluorocitrate in paclitaxel-evoked neuropathic pain. Acta Biochim Biophys Sin. 2016;48(10):902–908.
- Luo X, Huh Y, Bang S, et al. Macrophage toll-like receptor 9 contributes to chemotherapy-induced neuropathic pain in male mice. J Neurosci. 2019;39(35):6848–6864.
- Kalynovska N, Diallo M, Sotakova-Kasparova D, et al. Losartan attenuates neuroinflammation and neuropathic pain in paclitaxel-induced peripheral neuropathy. J Cell Mol Med. 2020;24(14):7949–7958.
- Nishida K, Kuchiiwa S, Oiso S, et al. Up-regulation of matrix metalloproteinase-3 in the dorsal root ganglion of rats with paclitaxel-induced neuropathy. Cancer Sci. 2008;99(8):1618–1625.
- Huang Z-Z, Li D, Liu C-C, et al. CX3CL1-mediated macrophage activation contributed to paclitaxel-induced DRG neuronal apoptosis and painful peripheral neuropathy. Brain Behav Immun. 2014;40:155–165.
- Kim E, Hwang S-H, Kim H-K, et al. Losartan, an angiotensin II type 1 receptor antagonist, alleviates mechanical hyperalgesia in a rat model of chemotherapy-induced neuropathic pain by inhibiting inflammatory cytokines in the dorsal root ganglia. Mol Neurobiol. 2019;56(11):7408–7419.
- Sekiguchi F, Domoto R, Nakashima K, et al. Paclitaxel-induced HMGB1 release from macrophages and its implication for peripheral neuropathy in mice: evidence for a neuroimmune crosstalk. Neuropharmacology. 2018;141:201–213.
- Yan X, Maixner DW, Yadav R, et al. Paclitaxel induces acute pain via directly activating toll like receptor 4. Mol Pain. 2015;11:10.
- Krukowski K, Eijkelkamp N, Laumet G, et al. CD8+ t cells and endogenous IL-10 are required for resolution of chemotherapy-induced neuropathic pain. J Neurosci. 2016;36(43):11074–11083. Available from: https://pubmed.ncbi.nlm.nih.gov/27798187
- Makker PGS, Duffy SS, Lees JG, et al. Characterisation of immune and neuroinflammatory changes associated with chemotherapy-induced peripheral neuropathy. PLOS One. 2017;12(1):e0170814.
- Burgos E, Gomez-Nicola D, Pascual D, et al. Cannabinoid agonist WIN 55,212-2 prevents the development of paclitaxel-induced peripheral neuropathy in rats. Possible involvement of spinal glial cells. Eur J Pharmacol. 2012;682(1–3):62–72.
- Gui Y, Zhang J, Chen L, et al. Icariin, a flavonoid with anti-cancer effects, alleviated paclitaxel-induced neuropathic pain in a SIRT1-dependent manner. Mol Pain. 2018;14:1744806918768970.
- Ruiz-Medina J, Baulies A, Bura SA, et al. Paclitaxel-induced neuropathic pain is age dependent and devolves on glial response. Eur J Pain. 2013;17(1):75–85.
- Zhao Y-X, Yao M-J, Liu Q, et al. Electroacupuncture treatment attenuates paclitaxel-induced neuropathic pain in rats via inhibiting spinal glia and the TLR4/NF-κB pathway. J Pain Res. 2020;13:239–250.
- Liu X, Tonello R, Ling Y, et al. Paclitaxel-activated astrocytes produce mechanical allodynia in mice by releasing tumor necrosis factor-α and stromal-derived cell factor 1. J Neuroinflammation. 2019;16(1):209.
- Gao M, Yan X, Weng H-R. Inhibition of glycogen synthase kinase 3β activity with lithium prevents and attenuates paclitaxel-induced neuropathic pain. Neuroscience. 2013;254:301–311.
- Cata JP, Weng H-R, Chen J-H, et al. Altered discharges of spinal wide dynamic range neurons and down-regulation of glutamate transporter expression in rats with paclitaxel-induced hyperalgesia. Neuroscience. 2006;138(1):329–338.
- Weng H-R, Aravindan N, Cata JP, et al. Spinal glial glutamate transporters downregulate in rats with taxol-induced hyperalgesia. Neurosci Lett. 2005;386(1):18–22.
- Masocha W. Astrocyte activation in the anterior cingulate cortex and altered glutamatergic gene expression during paclitaxel-induced neuropathic pain in mice. PeerJ. 2015;3:e1350.
- Saika F, Matsuzaki S, Kobayashi D, et al. Chemogenetic regulation of CX3CR1-expressing microglia using Gi-DREADD exerts sex-dependent anti-allodynic effects in mouse models of neuropathic pain. Front Pharmacol. 2020;11:925.
- Ha J-W, You M-J, Park H-S, et al. Differential effect of LPS and paclitaxel on microglial functional phenotypes and circulating cytokines: the possible role of CX3CR1 and IL-4/10 in blocking persistent inflammation. Arch Pharm Res. 2019;42(4):359–368.
- Ochi-Ishi R, Nagata K, Inoue T, et al. Involvement of the chemokine CCL3 and the purinoceptor P2X7 in the spinal cord in paclitaxel-induced mechanical allodynia. Mol Pain. 2014;10(1):53.
- Janes K, Little JW, Li C, et al. The development and maintenance of paclitaxel-induced neuropathic pain require activation of the sphingosine 1-phosphate receptor subtype 1. J Biol Chem. 2014;289(30):21082–21097.
- Kamei J, Hayashi S, Sakai A, et al. Rikkunshito prevents paclitaxel-induced peripheral neuropathy through the suppression of the nuclear factor kappa B (NFκB) phosphorylation in spinal cord of mice. PLoS One. 2017;12(2):e0171819.
- Kim HK, Hwang S-H, Lee S-O, et al. Pentoxifylline ameliorates mechanical hyperalgesia in a rat model of chemotherapy-induced neuropathic pain. Pain Phys. 2016;19(4):E589–600.
- Janes K, Esposito E, Doyle T, et al. A3 adenosine receptor agonist prevents the development of paclitaxel-induced neuropathic pain by modulating spinal glial-restricted redox-dependent signaling pathways. Pain. 2014;155(12):2560–2567.
- Ledeboer A, Jekich BM, Sloane EM, et al. Intrathecal interleukin-10 gene therapy attenuates paclitaxel-induced mechanical allodynia and proinflammatory cytokine expression in dorsal root ganglia in rats. Brain Behav Immun. 2007;21(5):686–698.
- Al-Mazidi S, Alotaibi M, Nedjadi T, et al. Blocking of cytokines signalling attenuates evoked and spontaneous neuropathic pain behaviours in the paclitaxel rat model of chemotherapy-induced neuropathy. Eur J Pain. 2018;22(4):810–821.
- Kim HK, Hwang S-H, Oh E, et al. Rolipram, a selective phosphodiesterase 4 inhibitor, ameliorates mechanical hyperalgesia in a rat model of chemotherapy-induced neuropathic pain through inhibition of inflammatory cytokines in the dorsal root ganglion. Front Pharmacol. 2017;8:885.
- Miranda HF, Sierralta F, Aranda N, et al. Antinociception induced by rosuvastatin in murine neuropathic pain. Pharmacol Rep. 2018;70(3):503–508.
- Parvathy SS, Masocha W. Matrix metalloproteinase inhibitor COL-3 prevents the development of paclitaxel-induced hyperalgesia in mice. Med Princ Pract. 2013;22(1):35–41.
- Luo H, Liu HZ, Zhang WW, et al. Interleukin-17 regulates neuron-glial communications, synaptic transmission, and neuropathic pain after chemotherapy. Cell Rep. 2019;29(8):2384–2397.
- Chen LH, Yeh YM, Chen YF, et al. Targeting interleukin-20 alleviates paclitaxel-induced peripheral neuropathy. Pain. 2020;161(6):1237–1254.
- Huehnchen P, Muenzfeld H, Boehmerle W, et al. Blockade of IL-6 signaling prevents paclitaxel-induced neuropathy in C57Bl/6 mice. Cell Death Dis. 2020;11(1):45.
- Huang J, Chen D, Yan F, et al. JTC-801 alleviates mechanical allodynia in paclitaxel-induced neuropathic pain through the PI3K/Akt pathway. Eur J Pharmacol. 2020;883:173306.
- Tonello R, Lee SH, Berta T. Monoclonal antibody targeting the matrix metalloproteinase 9 prevents and reverses paclitaxel-induced peripheral neuropathy in mice. J Pain. 2019;20(5):515–527.
- Zhang H, Boyette-Davis JA, Kosturakis AK, Li Y, et al. Induction of monocyte chemoattractant protein-1 (MCP-1) and its receptor CCR2 in primary sensory neurons contributes to paclitaxel-induced peripheral neuropathy. J Pain. 2013;14(10):1031–1044.
- Li D, Huang Z-Z, Ling Y-Z, et al. Up-regulation of CX3CL1 via Nuclear factor-κB-dependent histone acetylation is involved in paclitaxel-induced peripheral neuropathy. Anesthesiology. 2015;122(5):1142–1151.
- Li D, Chen H, Luo X-H, et al. CX3CR1-mediated Akt1 activation contributes to the paclitaxel-induced painful peripheral neuropathy in rats. Neurochem Res. 2016;41(6):1305–1314.
- Zhang X-L, Ou-Yang H-D, Li Z-Y, et al. Epigenetic upregulation of CXCL12 expression mediates antitubulin chemotherapeutics-induced neuropathic pain. Pain. 2017;158(4):637–648.
- Manjavachi MN, Passos GF, Trevisan G, et al. Spinal blockage of CXCL1 and its receptor CXCR2 inhibits paclitaxel-induced peripheral neuropathy in mice. Neuropharmacology. 2019;151:136–143.
- Zanata GC, Pinto LG, da Silva NR, et al. Blockade of bradykinin receptors or angiotensin II type 2 receptor prevents paclitaxel-associated acute pain syndrome in mice. Eur J Pain. 2020;25(1):189–198.
- Resham K, Sharma SS. Pharmacological interventions targeting Wnt/β-catenin signaling pathway attenuate paclitaxel-induced peripheral neuropathy. Eur J Pharmacol. 2019;864:172714.
- Mogil JS. Qualitative sex differences in pain processing: emerging evidence of a biased literature. Nat Rev Neurosci. 2020;21(7):353–365.
- Kilkenny C, Parsons N, Kadyszewski E, et al. Survey of the quality of experimental design, statistical analysis and reporting of research using animals. PLoS One. 2009;4(11):e7824.
- Attal N. Pharmacological treatments of neuropathic pain: the latest recommendations. Rev Neurol. 2018;175(1–2):46–50.
- Jones LW, Fels DR, West M, et al. Modulation of circulating angiogenic factors and tumor biology by aerobic training in breast cancer patients receiving neoadjuvant chemotherapy. Cancer Prev Res. 2013;6(9):925–937.
- Glass OK, Inman BA, Broadwater G, et al. Effect of aerobic training on the host systemic milieu in patients with solid tumours: an exploratory correlative study. Br J Cancer. 2015;112(5):825–831.
- Kleckner IR, Kamen C, Cole C, et al. Effects of exercise on inflammation in patients receiving chemotherapy: a nationwide NCORP randomized clinical trial. Support Care Cancer. 2019;27(12):4615–4625.
- Courneya KS, McKenzie DC, Mackey JR, et al. Effects of exercise dose and type during breast cancer chemotherapy: multicenter randomized trial. JNCI J Natl Cancer Inst. 2013;105(23):1821–1832.
- Travier N, Velthuis MJ, Steins Bisschop CN, et al. Effects of an 18-week exercise programme started early during breast cancer treatment: a randomised controlled trial. BMC Med. 2015;13:121.
- van Waart H, Stuiver MM, van Harten WH, et al. Effect of low-intensity physical activity and moderate- to high-intensity physical exercise during adjuvant chemotherapy on physical fitness, fatigue, and chemotherapy completion rates: results of the PACES randomized clinical trial. J Clin Oncol. 2015;33(17):1918–1927.
- Mijwel S, Backman M, Bolam KA, et al. Highly favorable physiological responses to concurrent resistance and high-intensity interval training during chemotherapy: the OptiTrain breast cancer trial. Breast Cancer Res Treat. 2018;169(1):93–103.