Abstract
Purpose/objectives
Proton beam therapy (PBT) may provide a dosimetric advantage in sparing soft tissue and bone for selected patients with extremity soft sarcoma (eSTS). We compared PBT with photons plans generated using intensity-modulated radiotherapy (IMRT) and three-dimensional conformal radiotherapy (3D-CRT).
Materials/methods
Seventeen patients previously treated with pencil beam scanning PBT were included in this study. Of these patients, 14 treated with pre-operative 50 Gy in 25 fractions were analyzed. IMRT and 3D-CRT plans were created to compare against the original PBT plans. Dose-volume histogram (DVH) indices were evaluated amongst PBT, IMRT, and 3D plans. Kruskal–Wallis rank sum tests were used to get the statistical significance. A p value smaller than .05 was considered to be statistically significant.
Results
For the clinical target volume (CTV), D2%, D95%, D98%, Dmin, Dmax, and V50Gy, were assessed. Dmin, D1%, Dmax, Dmean, V1Gy, V5Gy, and V50Gy were evaluated for the adjacent soft tissue. D1%, Dmax, Dmean, and V35-50% were evaluated for bone. All plans met CTV target coverage. The PBT plans delivered less dose to soft tissue and bone. The mean dose to the soft tissue was 2 Gy, 11 Gy, and 13 Gy for PBT, IMRT, and 3D, respectively (p < .001). The mean dose to adjacent bone was 15 Gy, 26 Gy, and 28 Gy for PBT, IMRT, and 3D, respectively (p = .022).
Conclusion
PBT plans for selected patients with eSTS demonstrated improved sparing of circumferential soft tissue and adjacent bone compared to IMRT and 3D-CRT. Further evaluation will determine if this improved dosimetry correlates with reduced toxicity and improved quality of life.
Introduction
Limb salvage with surgical excision and pre-operative or post-operative radiation is the preferred treatment strategy for extremity soft tissue sarcoma (eSTS) [Citation1,Citation2]. Radiation improves local control rates but may negatively impact function and quality of life [Citation3–5]. Timing of radiotherapy, dose, and field size correlate to specific toxicities such as decreased range of motion, fibrosis, and lymphedema. Developments in photon radiation planning such as three-dimensional conformal radiotherapy (3D-CRT), intensity-modulated radiotherapy (IMRT), and volumetric modulated arc therapy (VMAT) may lead to improved tissue sparing and thus reduced treatment toxicity compared to conventionally planned radiotherapy [Citation6–8]. In addition to more sophisticated treatment planning, image-guided radiotherapy (IGRT) allows for decreased target margins and smaller target volumes resulting in a significant decrease in late toxicity [Citation8].
Proton beam therapy (PBT) may also be advantageous for eSTS patients with anatomically challenging presentations such as proximal medial thigh location or semi-circumferential tumor. Protons are heavy charged particles that undergo small angle scattering and deposit maximum energy per length close to the end of the range, termed the ‘Bragg’ peak [Citation9]. PBT may be advantageous given the improved target conformality and reduction in integral dose [Citation10–12]. In particular, PBT may lead to a reduction in dose to soft tissue and bone, allowing for a decreased risk of chronic lymphedema or late bone fracture. In this study, we performed a dosimetric comparison of pencil beam scanning PBT, IMRT, and 3D-CRT for patients with eSTS. We hypothesize that PBT will lead to better sparing of soft tissue and bone compared to IMRT and 3D-CRT.
Materials and methods
After approval by the institutional review board (IRB), 17 eSTS patients previously treated with PBT were identified, and 14 of these patients treated with pre-operative intent were the subjects for this study. Patient positioning was determined during the simulation, and proper immobilization was used to ensure daily reproducibility. A treatment planning CT was performed without and with intravenous contrast.
Gross tumor volume (GTV) was delineated based on the CT simulation and fusion of magnetic resonance imaging (MRI) and positron emission tomography (PET) imaging when available. The clinical target volume (CTV) was an expansion of the GTV to include adjacent tissue at risk for microscopic extension using consensus guidelines [Citation13]. The CTV was constrained by anatomic barriers, including fascia, bone, or compartment. The adjacent circumferential soft tissue and bone were contoured as organs at risk (OARs). The soft tissue was delineated by setting a region of interest (ROI) box 2 cm above and below the superior and inferior edge of the CTV or PTV. Then, the target volume and the bone avoidance structure are cropped away to create the soft tissue avoidance structure. The bone was contoured similarly by using an ROI 2 cm above and below the superior and inferior edges of the target volume.
Planning technique
Proton plans were generated using pencil beam spot scanning using single-field (SFO) or multi-field optimization (MFO) [Citation14,Citation15]. With SFO, each proton field is optimized to cover the target uniformly [Citation14,Citation15]. With MFO, spot intensities from multiple fields are optimized concurrently to create a dose fluence generating a highly conformal dose distribution [Citation14,Citation15]. Treatment plans typically included two to three fields, with all fields treated daily. Beam-specific optimization target volumes (OTV) and scanning target volumes (STV) were created during the planning process to account for uncertainties in proton range and daily positioning. Beam angles and field design were selected to have optimal radiologic path length, normal tissue sparing, and control the potential high radiobiologic dose at each beam’s distal end of the proton range due to the high linear energy transfer in proton therapy [Citation16]. Bolus was never applied for PBT (but range shifter was used instead). The plans were evaluated with 3 mm patient setup errors and 3% proton range uncertainty scenarios to ensure robust target coverage [Citation14]. The dose prescription was described in centigray radiobiologic equivalent (cGy RBE) assuming a fixed relative biological effectiveness (RBE) of 1.1. Proton plans were evaluated using dose-volume histogram (DVH) indices for both target volume coverage and OAR sparing.
In IMRT or 3D-CRT planning, a 5 mm PTV was applied as a uniform expansion from the CTV, adhering to our clinical practice. Beam angle selection for 3D-CRT plans consisted of angles that would provide optimal coverage considering sparing normal tissue and OARs. IMRT planning with volumetric modulated arc therapy (VMAT) utilized two to three half arcs depending on the complexity and location of the tumor. Bolus was utilized for cases where the CTV extended to the skin surface, and coverage was not adequate without it. All cases were optimized to meet the plan objectives for each case best.
Dose prescription and dose-volume constraints
The prescription dose was 5000 cGy RBE in 25 fractions for all patients. Plans were evaluated using DVH indices, including the mean and median doses to the CTV, adjacent soft tissue, and bone. For the CTV, dose to 2 cc (D2cc), dose to 95% (D95%), dose to 98% (D98%), volume receiving 50 Gy (V50Gy), minimum dose (Dmin), and maximum point dose (Dmax) were assessed. For the adjacent soft tissue, Dmin, dose to 1% (D1%), Dmax, Dmean, volume receiving 1 Gy (V1Gy), volume receiving 5 Gy (V5Gy), and volume receiving 50 Gy (V50Gy) were evaluated. D1%, Dmax, Dmean, and V35-50Gy were evaluated for adjacent bone. For all plans, the following dose constraints were followed: 1) CTV V100% ≥ 99% (at least 99% of the clinical target volume receives 100% of the dose). 2) Soft tissue Dmean <5000 cGy (mean dose to soft tissue is less than 5000 cGy). 3) Bone Dmean <5000 cGy (mean dose to adjacent bone is less than 5000 cGy). 4) Bone Avoidance V4000 cGy < 50% (less than 50% of bone volume receives 4000 cGy). 5.) Bone Avoidance V5000 cGy <50%. CTV conformality was evaluated using the RTOG conformity index (CI), the ratio of the volume of 95% isodose line to the clinical target volume. CTV heterogeneity was compared using the heterogeneity index (HI), defined as the ratio of the maximum point dose and the prescription dose.
For important OAR DVH indices, the means and medians were calculated. Kruskal–Wallis rank sum tests were used to compare distributions of continuous variables.
Results
Tumor location was as follows: 9 (64%) proximal lower extremity, 1 (7%) distal lower extremity (knee), 3 (22%) proximal upper extremity, and 1 (7%) distal upper extremity (hand). Of the 14 patients receiving proton beam therapy, five proton patient plans were planned with SFO, and nine patients were planned with MFO. A representative case comparing dose distributions for PBT, IMRT, and 3D-CRT is shown in . Target coverage was within acceptability criteria for all PBT, IMRT, and 3D-CRT plans. Mean CTV coverage, soft tissue, and bone dose are summarized in and . The mean dose to the soft tissue was 2 Gy (range: 0 − 4.5 Gy), 11 Gy (range: 8–17 Gy), and 13 Gy (range: 5–32 Gy) for PBT, IMRT, and 3D-CRT plans, respectively. PBT demonstrated a statistically significantly lower mean dose to the soft tissue than either IMRT or 3D-CRT (p < .001). The mean dose to adjacent bone was 15 Gy (range: 1–35 Gy), 26 Gy (range: 10–40 Gy), and 28 Gy (range: 5–48 Gy) for PBT, IMRT, and 3D, respectively, which also was significantly lower for PBT (p = .022). summarizes specific dose-volume parameters for CTV, soft tissue, and bone.
Figure 1. Axial and coronal CT dosimetry demonstrating a PBT (A,B), IMRT/VMAT (C,D), and 3D-CRT (E,F) treatment plan.
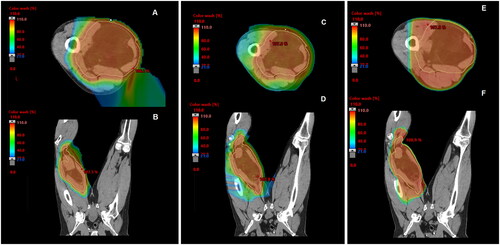
Figure 2. Mean DVH indices of PBT, IMRT/VMAT, and 3D treatment plans for CTV, bone, and soft tissue.
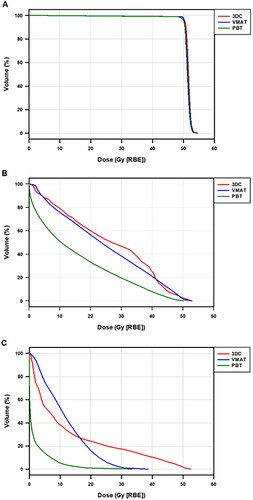
Table 1. Mean and median dose-volume parameters for clinical target volume, soft tissue, and bone.
Table 2. Specific mean dose volume parameters for clinical target volume, soft tissue, and bone dose-volume parameters.
The mean CI was 1.5, 1.9, and 3.4 for PBT, IMRT/VMAT, and 3D-CRT, respectively (). Conformity was significantly improved in the PBT plans compared with IMRT and 3DCRT. (p < .001). The mean HI was 1.092, 1.093, and 1.068 for PBT, IMRT, and 3D-CRT, respectively. There was a significant difference in HI for PBT, IMRT, and 3D plans (p < .001).
Discussion
While maintaining equivalent target coverage, this study suggests that pencil beam scanning PBT can produce a more conformal and heterogeneous plan while reducing the dose to the adjacent bone and non-target soft tissue compared to IMRT and 3D-CRT in selected patients with eSTS. There is minimal data regarding PBT's potential benefits for this patient population. In an early experience, PBT as pre-operative treatment of extremity sarcoma was shown to have a wound complication rate and local control similar to historical controls [Citation17]. In their study, Fogliata et al. reported a dosimetric comparison between VMAT and PBT on ten eSTS patients treated with post-operative radiation to 66 Gy in 25 fractions [Citation18]. The target coverage receiving 95% of the prescription dose was 4% higher for PBT versus VMAT-IMRT [Citation18]. They also noted statistically significant higher dose homogeneity evaluating standard deviation inside the PTV (2.3 Gy for protons and 3.0 Gy for IMRT) [Citation18]. Furthermore, they noted no significant difference in bone maximum dose of 50 Gy to 1 cm3 for protons versus photons [Citation18]. The most considerable difference between proton and photon plans was demonstrated for medium to low doses (V35Gy and V40Gy).
The current study evaluates treatment techniques in the pre-operative radiotherapy setting. Radiotherapy dose, the timing of radiotherapy, and field size have been shown to correlate with specific toxicity following radiotherapy. For instance, pre-operative radiotherapy followed by wide local excision has been shown to lead to a higher rate of wound complications but lower rates of fibrosis and lymphedema [Citation4]. On the other hand, post-operative RT, which traditionally employs doses up to 66 Gy and large field sizes, is associated with higher rates of fibrosis and lymphedema [Citation4]. PBT may be advantageous by limiting the volume treated and sparing the dose to the bone and lymphatic strip. Karasek et al. demonstrated that patients with eSTS receiving doses higher than 55 Gy to large treatment volumes suffered from increased fibrosis, skin changes, and impaired function and strength [Citation19]. Although a rare late toxicity, bonefracture can occur if 50 Gy is delivered to the entire bone circumference, bone exposure is present, periosteal stripping is performed during surgery, or perioperative chemotherapy is used [Citation20]. In a small cohort of patients who developed late bone fracture following radiation therapy for eSTS, it was demonstrated that radiation induced osteitis or bone fracture is associated with bone V40 > 64% [Citation21]. A reduction in bone fracture incidence was shown if mean bone dose was less than 37 Gy [Citation21]. We demonstrated a median bone dose of 14.1 Gy with PBT, which could indicate there is a low risk of bone fracture when protons are used. One caveat is that the uncertainty over possible higher radiobiologic effective dose due to the high LET at the end of proton range in bone, and therefore clinical data is warranted.
It is a common understanding of extremity sarcoma radiotherapy that sparing a segment of the circumferential limb from the total dose is critical to reducing the risk of lymphedema. In their evaluation of toxicity following adjuvant radiotherapy, Stinson et al. demonstrated that a larger length of radiation therapy field (greater than 35 cm) correlated with higher rates of edema. However, field sizes are now much smaller with image guidance and IMRT; therefore, the risk of lymphedema should theoretically be reduced. The specific relationship between soft tissue circumferential dose and the potential risk of lymphedema is less defined. There is no data in the literature that provides specific constraints for preventing lymphedema in patients with eSTS. There is data, however, which demonstrates that the dose to soft tissue and skin can be reduced. In their comparative study evaluating IMRT and 3DCRT as pre-operative treatment, Griffin et al. demonstrated that IMRT decreased the dose to the skin and subcutaneous tissues overlying the tumor that would be part of the future surgical flap [Citation22]. In their comparative study of post-operative IMRT and 3DCRT, Hong et al. demonstrated that soft tissue volume receiving prescription dose could be reduced by 78%. The D5 to soft tissue could be reduced by 13% with IMRT versus 3D techniques [Citation23]. The use of IMRT was associated with an increased volume of soft tissue receiving low dose bath, but mean doses were similar compared to 3DCRT [Citation23]. Additionally, IMRT significantly reduced the skin volume receiving the prescription dose and the dose encompassing 5% or 95% of the skin. Clinical follow-up and toxicity evaluation is warranted to determine whether dosimetric constraints could be defined in the pre-operative and post-operative setting. Although the soft tissue dose-volume constraints to limit the risk of edema remain poorly defined, this ultimately may prove to be the more significant dosimetric and clinical advantage of proton therapy over IMRT/VMAT.
The advantages of IMRT to 3D-CRT have been demonstrated in the post-operative setting. Stewart et al. compared 3D conformal therapy and IMRT for ten patients with eSTS undergoing post-operative therapy [Citation7]. Simple IMRT plans using two to three fields, as well as standard IMRT plans using four to five fields, were created. This study demonstrated that IMRT plans provided greater conformity and homogeneity, with significantly lower femur V45 using 2/3 field IMRT and 4/5 field IMRT than 3D-CRT. Additionally, 4/5 field IMRT resulted in significantly lower normal tissue V55 and normal tissue maximum dose than 3DCRT. As previously discussed, Hong et al. demonstrated that IMRT was superior in reducing the bone dose while minimizing hot spots in the soft tissue and skin [Citation23].
The delivery of proton beam therapy for extremity sarcoma requires robustness given setup and range uncertainties [Citation24–27]. The sensitivity of scanning beam PBT plans to uncertainties is defined as ‘plan robustness’. Range uncertainties arise from multiple sources, such as computer tomographic (CT) number uncertainty, tumor shrinkage, patient weight changes, and uncertainties associated with the conversion of CT numbers to stopping powers [Citation28]. Appreciable degradation of the delivered dose distributions may also occur from setup uncertainties that are due to misalignment of incident beams with the external patient anatomy and due to the realignment of the internal patient anatomy with respect to the target volumes. The factors mentioned impact dose distributions distally and proximally but also within it as well, impacting the overall robustness. For example, patients with proximal thigh sarcomas may be repositioned in the frog leg position daily, which may be quite challenging to reproduce daily. Setup errors often produce the greatest uncertainty when delivering a single fraction [Citation24].
In contrast to proton therapy, conventional photon radiation therapy relies on an additional margin around the clinical target volume (CTV) to form a planning target volume to handle set-up uncertainties [Citation29]. However, different strategies have been used to mitigate the impact of uncertainties in scanning beam PBT treatment planning. For SFO, the impact of uncertainties may be reduced with the use of appropriate beam specific distal and proximal margins. For MFO, the practice has been to use robust optimization to generate a robust scanning beam PBT plan [Citation29]. Image guidance is performed with daily kV-kV image matching to bone. The development of local edema is another common occurrence during the radiation for extremity sarcoma tumors that may impact the proton plan robustness more significantly than an IMRT/VMAT plan. This is monitored clinically as well as with verification scanning to assess changes in target coverage and to adapt the plan as needed.
Given the importance of maintaining robustness, immobilization is of high importance when delivering PBT for eSTS [Citation17]. At the time of simulation, custom fabricated cradles are used for proximal thigh sarcomas and thermoplastic molds and mask combinations over the foot and ankle for distal lower extremity sarcomas [Citation17]. With the use of customizable cradle, a frog leg position can be used daily with accurate daily set-up. The reproducibility is verified by radiation therapists, proton dosimetrists and physicists, and physician [Citation17]. Another benefit of proton beam therapy is the ability to maintain the contralateral extremity in a normal position, as there is less concern for low dose bath compared to photons [Citation17].
Our findings of improved dose distribution with PBT require further study to confirm a clinical benefit. However, advanced technology has been demonstrated to improve outcomes in eSTS. In the post-operative setting, IMRT has been associated with improved local control compared to conventional techniques [Citation30]. Image-guided radiation therapy can reduce late toxicities after pre-operative radiation by allowing for margin reduction [Citation8]. Recent guidelines from the American Society for Radiation Oncology (ASTRO) recommend using IMRT for eSTS to reduce the dose to OARS and reduce toxicity [Citation31].
Radiation is a well-known risk factor for the development of secondary malignancies [Citation32,Citation33]. In a report of childhood cancer survivors, secondary sarcomas occurred a median of 11.8 years from original diagnosis [Citation32]. After adjusting for treatment, children with primary sarcomas were more likely to develop a secondary sarcoma [Citation32]. In another study, a total of 159 of 7079 (2.2%) pediatric and young adult patients were found to have a secondary malignant neoplasm following treatment for sarcoma. Of the 3654 pediatric patients treated for a tumor involving the extremity sarcoma (bone and soft tissue), 76 (2.1%) developed a secondary malignant neoplasm. Protons are often considered in children and young adults with eSTS as the objective is to spare as much normal tissue as possible. However, adults with good prognosis should also be considered for proton beam therapy. A review of the SEER database of patients treated between 1973 and 2008 demonstrated increased risk of secondary sarcoma in patients treated with radiation therapy. Stratifying by age, standardized incidence ratios (SIR) of secondary sarcoma to be 5.32, 2.41, and 1.83 for ages 20–39, 40–59, and 60–79 years old, respectively [Citation33]. A caveat is that patients with primary sarcomas were excluded due to difficulty distinguished between recurrent primary sarcomas or development of secondary sarcomas. Proton therapy has demonstrated have reduced risk of secondary malignancy, with a relative risk reduction of 48% in comparison to photons [Citation34]. Therefore, it is not unreasonable to consider proton beam therapy in adults, especially those with larger tumors and sparing of circumferential tissue is more achievable with proton beam therapy.
Several factors limit this study. Although we demonstrate improved soft tissue and bone sparing with PBT, correlation with toxicity outcomes and patient-reported quality of life will be needed to demonstrate clinical benefit. Also, we acknowledge the need for careful patient selection in this study. Although the ASTRO guidelines currently recommend IMRT as the preferred technique, many patients with small to medium-sized tumors that are superficial or lateralized may still be treated well with 3D-CRT techniques.
Conclusion
In patients with eSTS, sophisticated radiotherapy techniques such as PBT and IMRT/VMAT are advantageous in reducing the dose to the adjacent bone and limb circumference, potentially leading to reduced late toxicity and improved function over time. For patients with anatomically challenging tumors that are large, central, and semi-circumferential around the bone, pencil beam scanning PBT may be a promising technique when available.
Ethics approval
This study was approved from the Institutional Review Board.
Acknowledgements
Data were presented in part at the PT-COG North America Seventh Annual Conference, Virtual, 18–20 September 2021.
Disclosure statement
No potential conflict of interest was reported by the author(s).
Data availability statement
Research data are stored in an institutional repository and will be shared upon request to the corresponding author.
Additional information
Funding
References
- Mundt AJ, Awan A, Sibley GS, et al. Conservative surgery and adjuvant radiation therapy in the management of adult soft tissue sarcoma of the extremities: clinical and radiobiological results. Int J Radiat Oncol Biol Phys. 1995;32(4):977–985.
- Rosenberg SA, Tepper J, Glatstein E, et al. The treatment of soft-tissue sarcomas of the extremities: prospective randomized evaluations of (1) limb-sparing surgery plus radiation therapy compared with amputation and (2) the role of adjuvant chemotherapy. Ann Surg. 1982;196(3):305–315.
- O'Sullivan B, Davis AM, Turcotte R, et al. Preoperative versus postoperative radiotherapy in soft-tissue sarcoma of the limbs: a randomised trial. Lancet. 2002;359(9325):2235–2241.
- Davis AM, O'Sullivan B, Turcotte R, et al. Late radiation morbidity following randomization to preoperative versus postoperative radiotherapy in extremity soft tissue sarcoma. Radiother Oncol. 2005;75(1):48–53.
- Davis AM, O'Sullivan B, Bell RS, et al. Function and health status outcomes in a randomized trial comparing preoperative and postoperative radiotherapy in extremity soft tissue sarcoma. J Clin Oncol. 2002;20(22):4472–4477.
- Alektiar KM, Brennan MF, Healey JH, et al. Impact of intensity-modulated radiation therapy on local control in primary soft-tissue sarcoma of the extremity. J Clin Oncol. 2008;26(20):3440–3444.
- Stewart AJ, Lee YK, Saran FH. Comparison of conventional radiotherapy and intensity-modulated radiotherapy for post-operative radiotherapy for primary extremity soft tissue sarcoma. Radiother Oncol. 2009;93(1):125–130.
- Wang D, Zhang Q, Eisenberg BL, et al. Significant reduction of late toxicities in patients with extremity sarcoma treated with image-guided radiation therapy to a reduced target volume: results of radiation therapy oncology group RTOG-0630 trial. J Clin Oncol. 2015;33(20):2231–2238.
- Paganetti H. Proton beam therapy. Bristol (UK): IOP Publishing; 2017.
- DeLaney TF, Haas RL. Innovative radiotherapy of sarcoma: proton beam radiation. Eur J Cancer. 2016;62:112–123.
- Keole S, Ashman JB, Daniels TB. Proton therapy for sarcomas. Cancer J. 2014;20(6):409–414.
- Frisch S, Timmermann B. The evolving role of proton beam therapy for sarcomas. Clin Oncol (R Coll Radiol). 2017;29(8):500–506.
- Wang D, Bosch W, Roberge D, et al. RTOG sarcoma radiation oncologists reach consensus on gross tumor volume and clinical target volume on computed tomographic images for preoperative radiotherapy of primary soft tissue sarcoma of extremity in radiation therapy oncology group studies. Int J Radiat Oncol Biol Phys. 2011;81(4):e525–e528.
- Quan EM, Liu W, Wu R, et al. Preliminary evaluation of multifield and single-field optimization for the treatment planning of spot-scanning proton therapy of head and neck cancer. Med Phys. 2013;40(8):081709.
- Paganetti H. Proton therapy physics - series in medical physics and biomedical engineering. Boca Raton (FL): CRC Press; 2011.
- Deng W, Yang Y, Liu C, et al. A critical review of LET-based intensity-modulated proton therapy plan evaluation and optimization for head and neck cancer management. Int J Part Ther. 2021;8(1):36–49.
- Laughlin BS, Golafshar MA, Ahmed S, et al. Early experience using proton beam therapy for extremity soft tissue sarcoma: a multicenter study. Int J Part Ther. 2022;9(1):1–11.
- Fogliata A, Scorsetti M, Navarria P, et al. Dosimetric comparison between VMAT with different dose calculation algorithms and protons for soft-tissue sarcoma radiotherapy. Acta Oncol. 2013;52(3):545–552.
- Karasek K, Constine LS, Rosier R. Sarcoma therapy: functional outcome and relationship to treatment parameters. Int J Radiat Oncol Biol Phys. 1992;24(4):651–656.
- Bishop AJ, Zagars GK, Allen PK, et al. Treatment-related fractures after combined modality therapy for soft tissue sarcomas of the proximal lower extremity: can the risk be mitigated? Pract Radiat Oncol. 2016;6(3):194–200.
- Dickie CI, Parent AL, Griffin AM, et al. Bone fractures following external beam radiotherapy and limb-preservation surgery for lower extremity soft tissue sarcoma: relationship to irradiated bone length, volume, tumor location and dose. Int J Radiat Oncol Biol Phys. 2009;75(4):1119–1124.
- Griffin AM, Euler CI, Sharpe MB, et al. Radiation planning comparison for superficial tissue avoidance in radiotherapy for soft tissue sarcoma of the lower extremity. Int J Radiat Oncol Biol Phys. 2007;67(3):847–856.
- Hong L, Alektiar KM, Hunt M, et al. Intensity-modulated radiotherapy for soft tissue sarcoma of the thigh. Int J Radiat Oncol Biol Phys. 2004;59(3):752–759.
- Malyapa R, Lowe M, Bolsi A, et al. Evaluation of robustness to setup and range uncertainties for head and neck patients treated with pencil beam scanning proton therapy. Int J Radiat Oncol Biol Phys. 2016;95(1):154–162.
- Unkelbach J, Bortfeld T, Martin BC, et al. Reducing the sensitivity of IMPT treatment plans to setup errors and range uncertainties via probabilistic treatment planning. Med Phys. 2009;36(1):149–163.
- Schaffner B, Pedroni E. The precision of proton range calculations in proton radiotherapy treatment planning: experimental verification of the relation between CT-HU and proton stopping power. Phys Med Biol. 1998;43(6):1579–1592.
- Schneider U, Pedroni E, Lomax A. The calibration of CT hounsfield units for radiotherapy treatment planning. Phys Med Biol. 1996;41(1):111–124.
- Liu C, Patel SH, Shan J, et al. Robust optimization for intensity modulated proton therapy to redistribute high linear energy transfer from nearby critical organs to tumors in head and neck cancer. Int J Radiat Oncol Biol Phys. 2020;107(1):181–193.
- Liu W, Zhang X, Li Y, et al. Robust optimization of intensity modulated proton therapy. Med Phys. 2012;39(2):1079–1091.
- Folkert MR, Singer S, Brennan MF, et al. Comparison of local recurrence with conventional and intensity-modulated radiation therapy for primary soft-tissue sarcomas of the extremity. J Clin Oncol. 2014;32(29):3236–3241.
- Salerno KE, Alektiar KM, Baldini EH, et al. Radiation therapy for treatment of soft tissue sarcoma in adults: executive summary of an ASTRO clinical practice guideline. Pract Radiat Oncol. 2021;11(5):339–351.
- Henderson TO, Rajaraman P, Stovall M, et al. Risk factors associated with secondary sarcomas in childhood cancer survivors: a report from the childhood cancer survivor study. Int J Radiat Oncol Biol Phys. 2012;84(1):224–230.
- Berrington de Gonzalez A, Kutsenko A, Rajaraman P. Sarcoma risk after radiation exposure. Clin Sarcoma Res. 2012;2(1):18.
- Chung CS, Yock TI, Nelson K, et al. Incidence of second malignancies among patients treated with proton versus photon radiation. Int J Radiat Oncol Biol Phys. 2013;87(1):46–52.