ABSTRACT
Background: Tendon disorders increasingly afflict our aging society but we lack the scientific understanding to clinically address them. Clinically relevant models of tendon disease are urgently needed as established small animal models of tendinopathy fail to capture essential aspects of the disease. Two-dimensional and three-dimensional cell and tissue culture models are similarly limited, lacking many physiological extracellular matrix cues required to maintain tissue homeostasis or guide matrix remodeling. These cues reflect the biochemical and biomechanical status of the tissue, and encode information regarding the mechanical and metabolic competence of the tissue. Tendon explants overcome some of these limitations and have thus emerged as a valuable tool for the discovery and study of mechanisms associated with tendon homeostasis and pathophysiology. Tendon explants retain native cell-cell and cell-matrix connections, while allowing highly reproducible experimental control over extrinsic factors like mechanical loading and nutritional availability. In this sense tendon explant models can deliver insights that are otherwise impossible to obtain from in vivo animal or in vitro cell culture models. Purpose: In this review, we aimed to provide an overview of tissue explant models used in tendon research, with a specific focus on the value of explant culture systems for the controlled study of the tendon core tissue. We discuss their advantages, limitations and potential future utility. We include suggestions and technical recommendations for the successful use of tendon explant cultures and conclude with an outlook on how explant models may be leveraged with state-of-the-art biotechnologies to propel our understanding of tendon physiology and pathology.
1. Introduction
1.1. Clinical need and role of tendon explant models
Musculoskeletal diseases accounts for over ⅓ of human years lived with disability, with tendon disorders representing 30–50% of all musculoskeletal-related clinical visits. Tendon disorders are associated with pain, swelling and restricted ranges of motion, are usually exacerbated by activity, and affect individuals across the demographic spectrumCitation1–Citation3. The most common tendon disorders involve the Achilles, patellar, rotator cuff, digital flexor, and elbow extensor tendons, often leading to despair for patients and frustration for the clinicians trying to treat themCitation4–Citation6. Unfortunately, the societal prevalence of tendon disorders is only increasing, in line with major risk factors including age, obesity, diabetes, mechanically demanding work and sport activitiesCitation7–Citation13. Yet despite ever-growing rates of incidence, substantial individual suffering, and an enormous collective socioeconomic burden, tendon disorders remain under-researched and poorly understoodCitation5. Current clinical approaches to treating tendon ailments involve pain management and physiotherapy over a course of several months, sometimes extending into years. The ability to introduce more effective therapies against tendon disease has been slow due to lacking knowledge on the molecular and cellular basis of tendon pathology and physiologyCitation14–Citation16.
A major obstacle to scientific and clinical advancement has been the persistent lack of valid models of human tendon disease. Such models are essential to clarify basic cellular mechanisms and to test novel therapeutic treatments. In this review, we discuss the potential of tendon explant models to fill this gap. They are uniquely powerful because they (1) preserve the cell’s native extracellular matrix (ECM) niche, (2) they can be viably maintained and longitudinally monitored for extended periods of time in ex vivo culture, (3) their physiology can be probed using available tools from the fields of cellular and molecular biology, and critically (4) the mechanics and structure of the (dys)functional matrix can be quantitatively phenotyped in a multi-scale fashion, providing a clear functional context in which to interpret and explain observed cellular behaviors.
1.2. Structure and function of the tendon extracellular matrix niche
While the cellular underpinnings of tendon physiology still remain mostly obscure, the manner by which the tendon ECM delivers its mechanical function is well understood. Tendon serves to transfer muscle force to bone, enabling movement and/or musculoskeletal stability. Healthy tendon tissue features a hierarchically structured collagen matrix that is anatomically optimized to sustain extraordinary mechanical demands. The tendon ECM houses tendon cells within a complex niche that can be very roughly divided into an electron-dense load-bearing collagen network embedded within a mostly non-collagen matrix ().
Figure 1. The tendon cell’s extracellular niche at a glance. (a) Tendon tissue comprises aligned collagen-rich fascicles interspersed with tendon stromal cells that reside in a highly complex/structured ECM niche. The interstitial matrix is mainly formed by collagen type I fibers, which constitute the most abundant ECM component in tendon and embrace the cells longitudinally. The non-collagenous matrix is rich in glycoproteins, in tendons mainly represented by the small leucin-rich proteoglycans (SLRPs). The pericellular matrix is a thin, continuous layer surrounding the cells and that contains collagen types V and VI, elastin and fibril associated collagens with interrupted triple helices (FACITs); (b) In healthy adult tendon tissue, the cells are closely connected to one another and remain in a quiescent state. In diseased tendon tissue, cell and ECM homeostasis are disturbed leading to cell activation and abnormal ECM production and turnover.
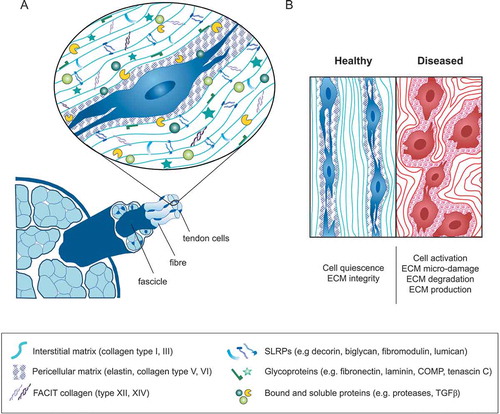
The dense tendon ECM comprises tightly packed fibrillar collagen molecules (thought to be dominantly type-I collagen amongst a potentially wide range of other collagen types) packed into so-called collagen fibrils with diameters ranging from 10s to 100s of nanometers and with lengths reaching to the centimeter scaleCitation17,Citation18. The fibrils are typically bundled along their load-bearing axis into cellular size scale structures called collagen fibers that are visible under a light microscope. These fiber bundles, and the cells that maintain them, in turn, constitute the tendon fascicle—considered to be the basic functional unit of tendon tissue. The tendon fascicle is also the smallest unit of tendon tissue useable as a tissue explantCitation17.
Aside from type I collagen structures, the peri-cellular matrix surrounding a tendon stromal fibroblast comprises a complex but still poorly understood milieu. The fibrillar collagens type III, V and XI are typically only present in small quantities in healthy tendonCitation19. While the full biological and biophysical role of many of these collagens is still unclear, collagen type III fraction within the ECM increases during tendon healing, and is considered to serve as a provisional matrixCitation20–Citation22. Collagens types V and XI are known to regulate collagen fibril formation in the processes known as fibrillogenesisCitation23–Citation27. Several non-fibrillar collagens are also present in tendon in low amounts, for instance, type VI is often localized to the pericellular matrix and is increased in abundance in injured tendonsCitation28. The fibril-associated collagens (FACITs) types XII and XIV are described as providing a molecular bridge between type I collagen and other matrix molecules, and play important roles during tendon developmentCitation29.
The non-collagen components of the matrix intersperses the collagen-dense structures at each hierarchical level. This hydrated interfibrillar matrix spans single collagen fibrils, fibers, and fascicles, encouraging fluid flow and nutrient transport, as well as accommodating interspersion of nerves and vesselsCitation30,Citation31. These wrapping layers are rich in proteoglycans (a distinct subgroup of glycoproteins), in tendon mainly represented by the small leucine-rich proteoglycan (SLRP, e.g. decorin, biglycan, fibromodulin, and lumican), versican and aggrecan, and contain a small fraction of elastin and other glycoproteins including fibronectin, laminins, lubricin, tenascin C and members of the thrombospondin family (e.g. cartilage oligomeric protein, also referred to as thrombospondin-5)Citation19,Citation32. This diversity of structural matrix proteins broadens the functional range of the tissue beyond tensile load-bearing, contributing to fiber and fascicle sliding, providing resistance to compression, supporting collagen matrix assembly and regulating cellular processes during tendon development and healingCitation33–Citation38. The quantitative and spatial distribution of matrix constituents varies between different tendon regions (myotendinous junction—mid-substance—enthesis) and tendon types (load-bearing and positional), with the roles of the various matrix component being an active area of researchCitation39,Citation40.
The tendon ECM is synthesized, maintained and remodeled by a still poorly defined population of fibroblast-like stromal cellsCitation19,Citation41,Citation42. Tendon stromal cells are traditionally characterized by an expression of mechanically regulated transcription factors such as scleraxis and mohawk that are essential to normal tendon developmentCitation43, as well as the expression of the glycoprotein tenomodulinCitation44. While scleraxis gene expression is perhaps most widely used as a tendon cell marker, it has a largely unknown function and is expressed in several tissues, such as the skin, heart and the corneaCitation42,Citation45. Recent studies, however, show that the tendon stroma comprises a heterogeneous cell population, including resident fibroblasts, macrophages, and stem/progenitor cells, where a clear picture on the individual cellular phenotypes, their origin and function is still emerging and urgently neededCitation42,Citation46–Citation48.
Tendon stromal cells are found interspersed between collagen fibers where they align longitudinally allowing effective cell-cell and cell-ECM communication. Together with their local collagen fiber network, tendon stromal cells form the fascicles defined as the load-bearing tendon core.
1.3. The extracellular matrix niche in tendon health and pathology
Considering the largely mechanical function of tendon and the extreme mechanical demands on tendon tissues, cellular sensing of mechanical signals is an essential aspect of tissue homeostasis. Mechanical loads are transmitted to tendon cells via their local matrix, with these cues guiding cell interactions with the matrix in a feedback loopCitation49–Citation54. Within the cell, mechanically regulated gene expression patterns and cell metabolism orchestrate protein synthesis (or post-translational modification) including ECM proteins, growth factors, cytokines, and proteasesCitation55. This process shapes the cellular microenvironment, tuning cell-cell and cell-matrix interactions with an impact on homeostatic balance. When these processes go awry, tendon tissue may shift (in whole, or in part) from a healthy to a dysfunctional stateCitation56. Indeed, histopathological studies have clearly shown that tendon disease states are characterized by cell activation and substantial changes in ECM components and organization ()). Alterations include hypercellularity, neovascularization (presence of a fibrovascular ECM), inflammation and a dysregulation of the critical balance between ECM remodeling proteases (MMPs, ADAMTs, cathepsins) and their inhibitors. When sustained these changes most probably reflect failed cellular efforts to re-establish tissue homeostasis, including an adequate restructuring of the tendon matrix with sustainably embedded resident cells. Hence, tendon tissue behavior is tightly dependent on specialized biophysical and biochemical niches defined by the ECM architecture, ECM biophysics, and context-dependent cell-matrix interactions. These aspects of the extracellular niche are essential to consider in any research model that is aimed at studying tendon homeostasis and mechanisms of pathology.
It is extremely difficult, if not impossible, to adequately recapitulate original ECM characteristics in vitro using engineered two-dimensional (2D) or three-dimensional (3D) culture systems. Very importantly, tendon explant models include these key features of the native tendon microenvironment. As such they are exceptionally well-suited for in vitro study of cell-cell and cell-matrix signaling—allowing insight into cellular communication that cannot be reasonably studied on cells in a simulated ECM niche. In the following sections, we make the case for increased use of explant models to uncover basic mechanisms of tendon physiology.
2. The utility and power of tendon explant culture models
2.1. Limitations of tendon in vivo and in vitro models
Studies on basic mechanisms of tissue physiology largely derive from either animal models or from in vitro experiments using isolated tendon cellsCitation44,Citation55. Although animal models are commonly viewed as a gold standard for the study of tendon repairCitation57, these models are limited in their ability to capture features of human tendon disease, for instance, they fail to mimic the limited intrinsic repair capacity of adult human tendon tissueCitation58. Tendon tissue response to an acute injury or mechanical overuse is species-specific, involving systemic healing processes with an often-unclear homology to humans. This seems to be particularly problematic in small animal models of tissue repair that can deviate from human system behaviors in centrally important ways, for instance in immune system involvement in healingCitation59,Citation60. On the other hand, large animal models, such as horses, dogs and sheep, naturally develop tendon disease, thus better resembling the human conditionCitation61–Citation63. Moreover, the large size of these animals facilitates the implementation of initiation defects and the mimicking of human tissue response to an implanted biomaterial. Yet, high housing and animal care costs, long breeding times and late skeletal maturity translate to low throughput and limit the practical use of large animals in tendon research.
Apart from the above-mentioned limitations, the use of animal models also introduces considerable ethical and time constraints. And although in vivo models are attractive for investigating tissue development and tissue repairCitation64, complex systemic regulation of tendon physiology and pathology presents many unknown yet potentially decisive confounding factors that cannot easily be controlled.
At the other end of the spectrum, 2D and 3D cell culture systems are substantially less complex than in vivo models and are widely used due to their practicality. Cell-based models are powerful and attractive in the sense that they accommodate the use of human cells, potentially allowing investigators to capture clinically and biologically relevant effects of human individuality (donor-to-donor variability). However, cell culture models come with many limitations. One major drawback is the loss of proportional heterogeneity from the isolated cell population during in vitro cell expansionCitation65. Such imbalanced conditions hamper drawing conclusions on whole tendon biology if only individual, more adhesive/proliferative cell sub-populations are selected in vitro. How to prevent or compensate for this loss is an active area of research, particularly how culture conditions influence the selection and growth of individual cell phenotypes. Nevertheless, cell-based models are particularly useful for high-throughput screening of response to experimental conditions and are in fact well suited for studies of robust cellular mechanisms that are relatively insensitive to physiological context. The physiological contexts that in vitro 3D models can adequately capture arguably includes neo-tendon formation after an acute injury, the study of initial interactions between recruited tendon cells and an implanted biomaterial or the crosstalk between tendon fibroblasts and other cell types (e.g. immune and stem cells). On one hand, cell models have been widely used to study cellular sensitivity to biochemical and biophysical cues from the cellular niche (model ECM ligands, mechanical substrate compliance, drugs). On the other hand, speculating on the biological relevance of the behaviors that emerge within cell models presents risks that often requires verification using other model systems and validation against clinical evidence.
2.2. Advantages of tendon explant models
When used appropriately, ex vivo explant models enable insights that cannot be gained using other in vitro and in vivo models of tendon physiology (). Whole tendon tissues maintained in viable culture conditions comprise original cell sub-populations with physiological cell organization, native ECM composition and native ECM architecture. In tendon explants, cells reside within a physiological ECM niche that can transfer mechanical stimuli and biological signals in a manner that largely mimics the in vivo complexity of cell-cell and cell-matrix communication. This allows highly controlled yet biologically relevant studies on cell-driven ECM turnover processes within the tissue stromal compartment. It also opens the possibility to directly investigate tissue functionality by measuring mechanical properties and initial aspects of crosstalk between tendon stromal tissues and other tissue compartments that encompass the immune, vascular, and nervous systems.
Figure 2. Advantages and limitations of tendon research models. In vivo models are attractive systems to investigate the development and repair of tendon tissue under physiological conditions. Tendon explant (ex vivo) models capture key features of native tendon tissue including a preserved ECM niche and can be applied to study ECM mechanobiology and cell-cell/matrix communication under near-physiological conditions. In vitro cell culture of tendon-derived cells enables the high-throughput and cost-effective investigation of complex, molecular research questions under fully controlled conditions.
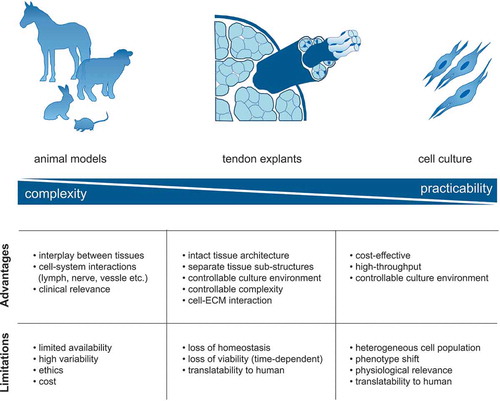
In comparison to in vivo models, explant models permit the deep study of the biological response of stromal tissues to external factors in a manner that the factors can be better isolated and controlled. In models that provide an abundance of explantable tissues (e.g. rodent tail tendon explant models), a range of experimental conditions can be investigated with minimal pain and distress to the animal. In being both scalable and highly reproducible, tissue explant models adhere to the advancement of the “3Rs” principle for animal experimentation (Replace, Reduce, Refine)Citation66. In this sense, explant model systems can mitigate some ethical limitations of in vivo models, while simultaneously alleviating economic and time-related burdens.
Another major technical advantage of explant models is that molecular tools and techniques that have been developed for standard cell culture experiments can be readily applied. This allows deep probing of molecular pathways, as well as high-throughput functional screening on both the tissue and molecular levels—a highly powerful combination. First, of course, the physiological relevance of the explant culture system must be established by convincing agreement with clinical experience and insights derived from the study of human tissue. After relevance is established there is immense potential to exploit these models for the identification of novel mechanisms that may translate to clinical strategies for disease prevention and/or the corresponding development and testing of new therapeutics.
2.3. Scientific advancements enabled by tendon explant models
2.3.1. Systems to study tissue crosstalk
Tendon explants have long been productively used for the ex vivo characterization of collagen tissue structure–function relationships attributed to the ECMCitation67–Citation76. Concomitant with ever-improving understanding of tendon cell homeostasis and our ability to physiologically maintain it in vitro, tendon explant models have been increasingly used to study cell-mediated processes. These processes necessarily include crosstalk between cells, their ECM and their nutritional environment, aspects that all closely interact to regulate tissue turnover in health, disease, and healingCitation77,Citation78. For instance, explanted canine flexor tendons have been harnessed to verify clinical impressions that retention of the tendon sheath is beneficial to healing after surgical repair of lacerated tendonsCitation79. This work demonstrates both the clinical value of explant models, and highlights the possibility to employ them for the discovery of basic mechanisms. Other examples demonstrating the potential of explant models include indirect co-culture approaches using human adipose-derived stem cells and human tendon explants that point to bi-directional crosstalk with a significant influence on resulting tissue architecture and secretion of ECM remodeling enzymesCitation80. Such studies using ex vivo tendon models demonstrate their value in isolating tissue- and cell-specific contributions to tendon physiology.
Perhaps the real power of explant model systems is that they enable investigation of tissue crosstalk. Tendon explants can be exposed to soluble factors to reveal cellular response within their native 3D matrix structure. As a recent example, a rat rotator cuff injury model for investigating bone-muscle-tendon crosstalk was introduced to investigate the role of inflammatory mediators on cell viability and ECM structureCitation81. Our own group has exploited equine tendon explants to investigate cellular sensitivity to multiscale changes in tendon matrix mechanics induced by exogenously applied cross-linking agentsCitation82. Others have investigated the effect of growth factors and/or hormones (e.g. platelet-derived growth factor, dexamethasone) on the metabolism of cells within their 3D matrix using rabbit flexor tendons, equine superficial digital flexor tendons and human explantsCitation78,Citation83–Citation85. While most studies to date have focused on fairly narrow functional readouts of cells (e.g. viability, gene expression) or their matrix (e.g. elastic modulus), explant models offer a large untapped potential for deeper biological investigation. We believe that ex vivo systems represent a powerful approach for the study of multiscale tissue properties and the characteristics of cells in their native 3D environment.
2.3.2. Mechano-culture systems
Given the mechano-sensitive nature of tendon in general and the fact that mechanical overuse is considered to be a driving factor in many tendon disorders, the inclusion of (cyclic) mechanical stresses is likely to be an essential requirement for faithful replication of the in vivo tendon nicheCitation86–Citation89. Appropriate experimental strategies are needed for this endeavor, as studying (healthy) human tendon tissue ex vivo is generally impossible. Mechanical stimulation of tendon models via controlled mechanical stretching systems are well-suited methods to simulate the effects of physiological or pathological loading on cells and ECM. Mechanical stretching systems may also be coupled to environmental conditions to mimic specific in vivo cellular and tissue niches in a standardized and controlled fashion. While mechanoculture systems for cell culture are widely used and well established, tissue-specific mechanoculture of 3D tissues is more challengingCitation90. In the tendon field, both commercially available and custom-designed bioreactor systemsCitation87,Citation91–Citation93 have been implemented over the past three decades. Our laboratory is among those pursuing this work, having recently described a tendon specific bioreactor system for long-term mechano-cultureCitation94 that allows individual specimen culture conditions and including tissue pre-tension. We see a great potential in exploring explant mechano-culture within specific biochemical niches as important to establish a baseline for physiologically relevant studies on tendon homeostasis, disease, and recovery.
However, a major challenge of tendon explant research is that many details regarding how different loading conditions (magnitude, frequency, rest) affect tendon development, homeostasis, damage, and repair remain unknown. Disparities in models, methods, and reporting of results have added fuel to the debate on exactly what constitutes physiological mechanical stimulus, and what constitutes pathological mechanical overload. Although it is widely accepted that chronic tendon problems arise from the excessive tensile load, excessive compressive forces (such as due to bony impingement) have been proposed as one specific cause of tendon disordersCitation95,Citation96. Further, tissue shear strains can yield various combinations of tensile and compressive loading at the cellular level, and may result in aberrant stimulus or even damage at the cellular levelCitation97–Citation99. Yet, past research has generally attributed tendon pathology to tensile overload because isolating a cell-level mechanical stimulus is difficult and so remains a major challenge in the field. However, explant models used in combination with functional microscopy are already revealing the relationships between tissue-level mechanical stresses, cell-level deformations, and central cellular mechanisms of mechanotransductionCitation72,Citation73,Citation97,Citation100–Citation102.
Despite opaque underlying cellular mechanisms, explant models have been productively employed to explore the strong link between tissues loads and biological response. For instance, experiments using avian flexor digitorum profundus tendon have indicated that aggressive mechanical loading results in a drastic reduction of tendon mechanical properties that are associated with higher secretion of collagenase and inflammatory mediators, such as prostaglandin E2 and nitric oxideCitation103,Citation104. Likewise, supra-physiological loading introduced areas of ECM damage in equine superficial digital flexor tendon and an increase of factors associated with matrix turnover, such as matrix metalloproteinase 13 (MMP-13) and C1,2CCitation105. Additionally, cyclooxygenase-2 (COX-2) and interleukin-6 (IL-6), both inflammatory markers, have been reported to be elevated in overloaded tendon ex vivo. Up-regulation of inflammatory (IL-6) and matrix degradation markers (MMP-3, MMP-13, C1,2C) in explants of bovine deep digital flexor tendons has confirmed these findingsCitation106. Similarly, by correlating ECM properties to such molecular markers, it was shown that increased IL-6 gene expression in explanted bovine extensor tendon fascicles occurs after application of a minimal damage loading protocol, indicating that this behavior is robustly modeled in explants even across speciesCitation107. Remarkably, the increase of mediators associated with inflammation and matrix remodeling is supported by observations of pathological human-derived tissues, implying that certain proinflammatory features of tendon disease are captured by ex vivo tendon overloadingCitation108,Citation109.
Complementing explant studies of supra-physiological loading, many studies have investigated sub-physiological loading. Removal of a mechanical stimulus upon explantation has been reported to dramatically increase MMP expression and secretion followed by a decrease of biomechanical properties in explanted canine, rabbit and rat tendonsCitation86,Citation110–Citation112. However, the response to changes in mechanical loading may be tendon-type specific as shown by comparing mechanically modulated gene expression in Achilles and supraspinatus tendon of ratsCitation112. Our own studies using mouse tail tendon explants have revealed that this mechanically gated proteolytic response to unloading is strongly dependent on the culture conditionsCitation94,Citation113, a context-dependency we speculate may be related to relative tissue vascularity. We believe these studies highlight how explant culture systems can be productively and powerfully utilized for investigation of tendon mechanobiology, allowing controlled application (or deprivation) of mechanical loads and the longitudinal functional tracking of mechanical properties.
2.4. Tendon fascicles—a unique tool to study the tendon stromal core
Within a given tendon, fascicles represent “base functional units” that are hierarchically ordered into core structures in a manner that provides a wide potential range of tissue level biomechanical propertiesCitation77. For the study of these functional units, rodent tail tendon fascicles are ideally suited. While few groups have mastered the art of extracting and exploiting highly cross-linked load-bearing tendon fasciclesCitation106,Citation114, tail tendon fascicles can be readily extracted with minimal mechanical or biological damage. Rat and mouse tail tendons have thus played an important role in studies of tendon structure–function relationships (e.g.Citation100,Citation115,Citation116), mostly because of their ease of isolation, a high yield of fascicles per animal, and a high degree of mechanical and biological reproducibilityCitation117. Furthermore, rodent tail tendon explants represent a powerful model to study mechanically regulated matrix remodelingCitation89,Citation118. The tail fascicle has well-aligned collagen structures with clearly defined tissue boundaries. This structure minimizes the chances of preparation artifacts and is favorable for the application of controlled loading regimes (tensile over- or underloading) to examine the effects of mechanobiological stimuliCitation111,Citation119. It is, however, important to note that cells within explanted fascicles can be damaged by supra-physiological but sub-rupture mechanical loads, and that micro-damage to the extracellular matrix of rat tail tendon fascicles is not recovered after several days of in vitro cultureCitation120. We speculate that the limited healing capacity of isolated tendon fascicles may be due to a lack of progenitor cells that are present within the interfascicular matrix. We view this as a major advantage that makes the fascicle an ideal model to isolate and investigate tendon core response to extrinsic influences. Straightforward stress-deprivation and/or static tension of tail tendon fascicles have already provided valuable understanding of mechanisms that are implicated in the development of pathology, healing and tissue homeostasisCitation50,Citation94,Citation111,Citation118,Citation119,Citation121–Citation125. We thus view rodent tail tendon explants as representing the ideal combination of experimental reproducibility and cross-species relevance for use as an in vitro model of “core tendon” biology.
Nonetheless, potentially important concerns have been raised regarding the physiological and clinical relevance of rodent tail tendons as a model for human disease and repairCitation77,Citation117,Citation126. The most cited shortcoming is the fact that human pathologies typically occur in load-bearing tendons while rodent tail tendons are positional tendons. Load-bearing and positional tendons have functionally related differences at both the cellular and compositional levels, for instance, major differences in the nature and extent of collagen cross-linking. However, the relevance of these differences is debatable, as highlighted by studies demonstrating that load-transfer and damage mechanisms are comparable in load-bearing and positional tendonsCitation99. Among other important similarities, the tail fascicle has mechanical properties that are comparable to mature load-bearing human tendon, with elastic moduli ranging from several hundred MPa to over 1 GPaCitation72,Citation127,Citation128. Additionally, the failure properties of isolated tail tendon fascicles reflect those of whole human tendon, with failure stresses on the order of 80 MPa and failure strains of approximately 10%Citation72,Citation128. Aside from the molecular differences in collagen cross-links (divalent aldimine cross-links in murine tail tendon tissue versus combined divalent aldimine and trivalent histidine cross-links in human), the ECM biochemical composition and architectural structure of rodent tendon is similar to healthy human tendonCitation50,Citation73. Similarly, the genetic regulation and protein composition involved in native tissues and in response to a tendon injury in certain mouse strains is remarkably close to that of human tendon—suggesting that many of the basic biological mechanisms of the tendon are conserved across species and anatomical originCitation129. In view of available evidence, we conclude that the tail tendon model is well suited for investigations within non-damaging physiological ranges of mechanical loading.
2.5. The challenges of maintaining tendon homeostasis in explants
Despite its many advantages, work with tendon explants comes with challenges. A central challenge is to understand the conditions required for tissue homeostasis. Very little is known about the healthy tendon environment, including mechanical, biological and physico-chemical factors that should be included to or excluded from culture conditions. Importantly, the very definition of tissue homeostasis remains a crucial topic. Because healthy adult tendon tissue is metabolically calmCitation130–Citation132 and becomes activated in disease, we believe that culture conditions should enable a homeostatic baseline in which tendon cells remain quiescent. To potentially achieve tendon homeostasis ex vivo, we believe the community must first uncover the full range of cellular and molecular changes that occur in tendon upon explantation. This major effort requires unbiased screening experiments on genes, proteins, metabolites and kinase activation with respect to the ex vivo culture conditions. If quiescence is unreachable, the anabolic and catabolic processes should at least be in balance as to maintain a steady-state of mechanical (functional) tissue properties over time. Indeed, such homeostatic-like conditions can be achieved by loading tendon tissueCitation89,Citation110, paying special attention to choosing experimental culture conditions (media composition, culture gas composition, and the physical stimulation environment; see below) or by inhibiting catabolic processesCitation125,Citation133.
Our own efforts to coax tendon explants toward functional recovery from imposed tissue damage have been challengingCitation120. This difficulty is implicitly confirmed by studies in the literature that almost exclusively rely on proxy measures of anabolic tissue response (i.e. cellular gene and protein expression) but which do not demonstrate the formation of functional matrix (for exampleCitation78,Citation134–Citation136). We nonetheless hold hope that a functional repair response can be achieved in tendon explant models, as has been achieved in other explanted model systems including spinal cord, meniscus and cochlear tissuesCitation137–Citation139.
3. A perspective on the utility and power of tail tendon explants as a model system for investigating tendon biology
The shift from homeostatic cell behavior after explant extraction from the host is poorly understood. However, it is well known that gene expression dramatically changes immediately after extraction and that the process of matrix proteolysis begins. From our own experience, we have learned that factors such as tissue temperature and oxygen tension strongly determine the outcome of explant culturesCitation113. By finding an appropriate loading regime and culture conditions that mimic in vivo conditions we may be able to limit such molecular shifts and approach the critical task of better understanding tendon homeostasisCitation94. Along these lines, it may then also be possible to extend the currently limited viability of ex vivo culture models. Monitoring cell viability in explant culture is important, as the release of inflammatory cytokines from apoptotic cells may trigger cell death in adjacent cells, which finally distort the experimental outcomesCitation81,Citation140. Additionally, it is essential to examine whether it is valid to extrapolate results from explant model systems to draw conclusions on human tendon biology and disease.
While existing long-term explant culture studies on tail tendon mostly employ rat tissue, our work on murine tail tendon explants is the first to explore this modelCitation94,Citation113. This is likely because mouse tendons are relatively small and fragile, which poses challenges in their handling. However, murine tail tendons offer several advantages: (1) their small size allows consistent tissue gradients of oxygen and nutrients from the margins to the center of the explant, (2) working with murine tissue is highly cost efficient and perhaps most importantly, (3) mouse models offer obvious advantages in terms of the available genetic models and molecular tools.
In this regard, our work with murine tail tendon fascicles has demonstrated their utility for controlled parametric investigation of tendon physiology and pathologyCitation94,Citation113. These initial efforts have focused on the maintenance of tissue homeostasis. In absence of tension and in standard cell culture conditions (10% serum, 37°C, 20 kPa pO2), mechanical tissue degradation in unloaded murine tail tendon fascicles is readily detectable after 1 week of cultureCitation113. These results are consistent with earlier studies showing that mechanical unloading leads to functional tissue breakdown, a process that can be abrogated by application of mechanical tensionCitation50,Citation89,Citation110,Citation118,Citation141. While the number of parameters that may be varied in the culture environment to maintain tissue stability are numerous and depend on the research question, our investigations have shown that various combination of tissue loading, oxygen tension, temperature and presence of serum can alternately promote or inhibit matrix breakdown.
3.1. Mechanical loading
It is difficult to draw conclusions regarding best practices for mechanoculture intended to maintain tendon explant homeostasis. An ideal protocol will likely depend on the anatomical role of the tendonCitation142,Citation143, among other factors. However, for positional tendons, such as murine tail tendon, it appears that loads in the range of the toe region in the stress–strain curve (e.g. those that yield crimp disappearance) are sufficient to maintain tissue mechanics at levels close to native tendon fasciclesCitation50,Citation89,Citation94,Citation118,Citation141, and without inducing mechanical damage to the cells or matrixCitation120. Arnoczky and colleagues discovered that the proteolytic erosion of mechanical properties can be delayed by simply anchoring the tendon ends to promote tensional homeostasisCitation118. While it is seems that static anchoring can maintain functional properties of the tissueCitation122,Citation144, the protective effects of tissue tension are not only cellular, as tissue tension can obscure enzymatic cleavage sites and can protect the collagen matrix from breakdownCitation145–Citation147.
3.2. Oxygen partial pressure
Our most recent work has focused on the role of tissue vascularity in driving cells from homeostasis and activating them toward matrix turnoverCitation113. Healthy tendon cells reside in a niche with physiologically low oxygen availabilityCitation148,Citation149. However, standard cell and tissue culture is normally performed at atmospheric oxygen partial pressure, which considerably exceeds the physiological condition that cells normally encounter in vivo. On this basis, we suggest that maintaining tendon explants at oxygen partial pressures around 3 kPa better approximates a healthy tissue environment.
3.3. Temperature
We have observed that culture temperature significantly influences explant stabilityCitation113, and we view it as imperative that one considers the relative physiological temperature of the tissue origin (core or extremity) when choosing the appropriate culture temperature for maintaining explant homeostasis. While the culture temperature will affect biological processes ranging from enzymatic activity to mass transport, it can also affect the biophysical stability of the extracellular matrix. Denaturation temperature and mechanical properties of different tendons significantly depend on the number of cross-links present in a tissueCitation54. The type of cross-links only indirectly affects the denaturation temperature of a tendon, namely through their effect on the hydration of the tissue (with a negative correlation between hydration and stability)Citation150. The fact that cross-links vary between tendons of different origins and develop with maturationCitation151 may reflect the local thermal environment of the tissue, again highlighting that physiological explant culture conditions will vary depending on the source of the tendon.
3.4. Serum
Serum contains a wide range of nutrients, hormones and growth factors. It is a poorly defined culture medium component containing ingredients that may or may not be physiologically relevant for maintaining tendon homeostasis. Because the healthy tendon is generally nutrient poor due to limited blood flow within the tendon core, we believe that a serum-free culture conditions mimic a healthy tendon microenvironment more suitably than serum-supplemented conditions. We have recently shown that serum may contribute to tissue degradation depending on the other above-mentioned culture parametersCitation113. Additionally, serum promotes cell proliferation and migration out of the tissue, a hallmark generally encountered in pathological tendon tissue.
4. Conclusions and perspective
Tendon explant models are a potentially powerful tool for research on human tendon health, damage and repair (). Unlike all other in vitro model systems, explants retain important features of healthy tendon tissue including cell-cell and cell-matrix connections that are central to tissue regulation and essential for tissue homeostasis. Tendon explants incorporate a near-native microenvironment for tendon cells while allowing a reduction of the systemic complexity that generally clouds interpretation of in vivo model responses. Finally, the ability to track biological readouts (such as live imaging) in parallel to direct measurement of functional (mechanical) tissue properties represents a major advantage of explant models. We view tendon fascicle explant models to offer an ideal foundation for investigating tissue crosstalk. Here the fascicle can be modularly combined with cell models and/or other tissue models, gradually and controllably increasing model complexity. For instance, co-culture models of tendon explants with endothelial or immune cells eventually embedded in supporting hydrogel matrices can be used to study compartment crosstalk between the tendon core and its extrinsic environment. Further, gene-editing methods (e.g. CRISPR/Cas9) allow within targeted studies to focus on the role of altered cell-cell and cell-matrix communication in tendon damage and repair. We envision that, over time, successful model systems will emerge that are able to capture clinically relevant features of tendon health and disease. We believe that such models will find increasing use within pharmacological high-content screening approaches. Ultimately, we expect that tendon explant models will play an integral role in unraveling biological mechanisms of tendon disease and accelerate the clinical translation of new therapies.
Figure 3. Next-generation applications of tendon explant models. Ex vivo tendon models bear a huge potential for future discoveries and applications. Studying the response of healthy and diseased explant model systems to externally applied perturbations will enable the better understanding of tendon physiology. The model phenotype may be specifically influenced and controlled by modulating the tissue explant culture environments and mechanical loading regimes. Furthermore, live imaging, the study of molecular mechanisms by employing gene-editing tools and the use of co-cultures systems for investigation of cellular crosstalk are promising future applications. Finally, an interdisciplinary approach and the clever combination of single applications will allow for high-throughput screening of therapeutics in the future.
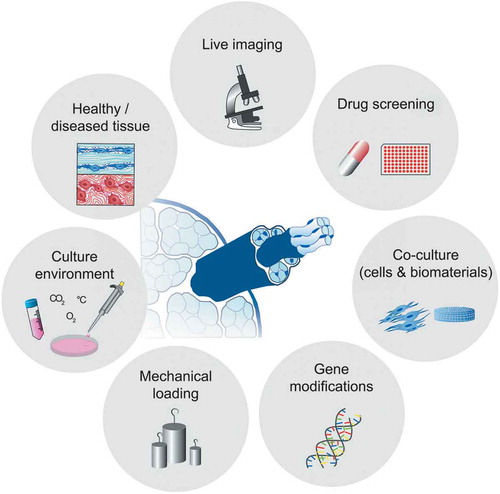
Disclosure statement
No potential conflict of interest was reported by the authors.
References
- Kaux JF, Forthomme B, Goff CL, Crielaard J-M, Croisier J-L. Current opinions on tendinopathy. J Sports Sci Med. 2011;10(2):238–253.
- Scott A, Backman LJ, Speed C. Tendinopathy: update on pathophysiology. J Orthop Sports Phys Ther. 2015;45(11):833–841. doi:10.2519/jospt.2015.5884.
- Maffulli N, Wong J, Almekinders LC. Types and epidemiology of tendinopathy. Clin Sports Med. 2003;22(4):675–692. doi:10.1016/S0278-5919(03)00004-8.
- McCreesh K, Lewis J. Continuum model of tendon pathology - where are we now? Int J Exp Pathol. 2013;94(4):242–247. doi:10.1111/iep.12029.
- Hopkins C, Fu S-C, Chua E, Hu X, Rolf C, Mattila VM, Qin L, Yung PS-H, Chan K-M. Critical review on the socio-economic impact of tendinopathy. Asia Pac J Sports Med Arthrosc Rehabil Technol. 2016;4:9–20. doi:10.1016/j.asmart.2016.01.002.
- Riley G. Tendinopathy–from basic science to treatment. Nat Clin Pract Rheumatol. 2008;4(2):82–89. doi:10.1038/ncprheum0700.
- Ranger TA, Wong AMY, Cook JL, Gaida JE. Is there an association between tendinopathy and diabetes mellitus? A systematic review with meta-analysis. Br J Sports Med. 2016;50(16):982–989. doi:10.1136/bjsports-2015-094735.
- Burner TW, Rosenthal AK. Diabetes and rheumatic diseases. Curr Opin Rheumatol. 2009;21(1):50–54. doi:10.1097/BOR.0b013e32831bc0c4.
- Gaida JE, Ashe MC, Bass SL, Cook JL. Is adiposity an under-recognized risk factor for tendinopathy? A systematic review. Arthritis Rheum. 2009;61(6):840–849. doi:10.1002/art.v61:6.
- Abate M, Schiavone C, Salini V, Andia I. Occurrence of tendon pathologies in metabolic disorders. Rheumatology (Oxford). 2013;52(4):599–608. doi:10.1093/rheumatology/kes395.
- Oliva F, Piccirilli E, Berardi AC, Frizziero A, Tarantino U, Maffulli N. Hormones and tendinopathies: the current evidence. Br Med Bull. 2016;117(1):39–58. doi:10.1093/bmb/ldv054.
- Xu Y, Murrell GA. The basic science of tendinopathy. Clin Orthop Relat Res. 2008;466(7):1528–1538. doi:10.1007/s11999-008-0286-4.
- Kaeding C, Best TM. Tendinosis: pathophysiology and nonoperative treatment. Sports Health. 2009;1(4):284–292. doi:10.1177/1941738109337778.
- Millar NL, Murrell GA, McInnes IB. Inflammatory mechanisms in tendinopathy - towards translation. Nat Rev Rheumatol. 2017;13(2):110–122. doi:10.1038/nrrheum.2016.213.
- Andarawis-Puri N, Flatow EL, Soslowsky LJ. Tendon basic science: development, repair, regeneration, and healing. J Orthop Res. 2015;33(6):780–784. doi:10.1002/jor.22869.
- Docheva D, Müller SA, Majewski M, Evans CH. Biologics for tendon repair. Adv Drug Deliv Rev. 2015;84:222–239. doi:10.1016/j.addr.2014.11.015.
- Handsfield GG, Slane LC, Screen HR. Nomenclature of the tendon hierarchy: an overview of inconsistent terminology and a proposed size-based naming scheme with terminology for multi-muscle tendons. J Biomech. 2016. doi:10.1016/j.jbiomech.2016.06.028.
- Svensson RB, Herchenhan A, Starborg T, Larsen M, Kadler KE, Qvortrup K, Magnusson SP. Evidence of structurally continuous collagen fibrils in tendon. Acta Biomater. 2017. doi:10.1016/j.actbio.2017.01.006.
- Thorpe CT, Birch HL, Clegg PD, Screen HRC. Tendon physiology and mechanical behavior: structure-function relationships. In: Gomes M, Reis R, Rodrigues, M, editors. Tendon regeneration understanding tissue physiology and development to engineer functional substitutes. London (UK): Elsevier; 2015. p. 3–39.
- Williams I, McCullagh K, Silver I. The distribution of types I and III collagen and fibronectin in the healing equine tendon. Connect Tissue Res. 1984;12(3–4):211–227. doi:10.3109/03008208409013684.
- Riley G, Harrall RL, Constant CR, Chard MD, Cawston TE, Hazleman BL. Tendon degeneration and chronic shoulder pain: changes in the collagen composition of the human rotator cuff tendons in rotator cuff tendinitis. Ann Rheum Dis. 1994;53(6):359–366. doi:10.1136/ard.53.6.359.
- Pingel J, Lu Y, Starborg T, Fredberg U, Langberg H, Nedergaard A, Weis M, Eyre D, Kjaer M, Kadler KE. 3-D ultrastructure and collagen composition of healthy and overloaded human tendon: evidence of tenocyte and matrix buckling. J Anat. 2014;224(5):548–555. doi:10.1111/joa.2014.224.issue-5.
- Birk DE, Fitch JM, Babiarz JP, Doane KJ, Linsenmayer TF. Collagen fibrillogenesis in vitro: interaction of types I and V collagen regulates fibril diameter. J Cell Sci. 1990;95(4):649–657.
- Fichard A, Kleman J-P, Ruggiero F. Another look at collagen V and XI molecules. Matrix Biol. 1995;14(7):515–531. doi:10.1016/S0945-053X(05)80001-0.
- Wenstrup RJ, Florer JB, Brunskill EW, Bell SM, Chervoneva I, Birk DE. Type V collagen controls the initiation of collagen fibril assembly. J Biol Chem. 2004;279(51):53331–53337. doi:10.1074/jbc.M409622200.
- Wenstrup RJ, Smith SM, Florer JB, Zhang G, Beason DP, Seegmiller RE, Soslowsky LJ, Birk DE. Regulation of collagen fibril nucleation and initial fibril assembly involves coordinate interactions with collagens V and XI in developing tendon. J Biol Chem. 2011;286(23):20455–20465. doi:10.1074/jbc.M111.223693.
- Sun M, Connizzo BK, Adams SM, Freedman BR, Wenstrup RJ, Soslowsky LJ, Birk DE. Targeted deletion of collagen V in tendons and ligaments results in a classic Ehlers-Danlos syndrome joint phenotype. Am J Pathol. 2015;185(5):1436–1447. doi:10.1016/j.ajpath.2015.01.031.
- Thakkar D, Grant TM, Hakimi O, Carr AJ. Distribution and expression of type VI collagen and elastic fibers in human rotator cuff tendon tears. Connect Tissue Res. 2014;55(5–6):397–402. doi:10.3109/03008207.2014.959119.
- Banos CC, Thomas AH, Kuo CK. Collagen fibrillogenesis in tendon development: current models and regulation of fibril assembly. Birth Defects Res Part C: Embryo Today: Rev. 2008;84(3):228–244. doi:10.1002/bdrc.v84:3.
- Thorpe CT, Birch HL, Clegg PD, Screen HRC. The role of the non-collagenous matrix in tendon function. Int J Exp Pathol. 2013;94(4):248–259. doi:10.1111/iep.12027.
- Taye N, Karoulias SZ, Hubmacher D. The “other” 15–40%: the role of non-collagenous extracellular matrix proteins and minor collagens in tendon. J Orthop Res. 2019. doi:10.1002/jor.24440.
- Kannus P. Structure of the tendon connective tissue. Scand J Med Sci Sports. 2000;10(6):312–320. doi:10.1034/j.1600-0838.2000.010006312.x.
- Banes AJ, Link GW, Bevin AG, Peterson HD, Gillespie Y, Bynum D, Watts S, Dahners L. Tendon synovial cells secrete fibronectin in vivo and in vitro. J Orthop Res. 1988;6(1):73–82. doi:10.1002/jor.1100060110.
- Jozsa L, Lehto M, Kannus P, Kvist M, Reffy A, Vieno T, Järvinen M, Demel S, Elek E. Fibronectin and laminin in Achilles tendon. Acta Orthop Scand. 1989;60(4):469–471. doi:10.3109/17453678909149322.
- Smith R, Gerard M, Dowling B, Dart AJ, Birch HL, Goodship AE. Correlation of cartilage oligomeric matrix protein (COMP) levels in equine tendon with mechanical properties: a proposed role for COMP in determining function‐specific mechanical characteristics of locomotor tendons. Equine Vet J. 2002;34(S34):241–244.
- Martin J, Mehr D, Pardubsky PD, Buckwalter JA. The role of tenascin‐C in adaptation of tendons to compressive loading. Biorheology. 2003;40(1, 2, 3):321–329.
- Kohrs RT, Zhao C, Sun YL, Jay GD, Zhang L, Warman ML, An KN, Amadio PC. Tendon fascicle gliding in wild type, heterozygous, and lubricin knockout mice. J Orthop Res. 2011;29(3):384–389. doi:10.1002/jor.21247.
- Frolova EG, Drazba J, Krukovets I, Kostenko V, Blech L, Harry C, Vasanji A, Drumm C, Sul P, Jenniskens GJ, Plow EF, Stenina-Adognravi O. Control of organization and function of muscle and tendon by thrombospondin-4. Matrix Biol. 2014;37:35–48. doi:10.1016/j.matbio.2014.02.003.
- Järvinen M, Kannus P, Kvist M, Isola J, Lehto M, Jozsa L. Macromolecular composition of the myotendinous junction. Exp Mol Pathol. 1991;55(3):230–237. doi:10.1016/0014-4800(91)90003-G.
- Merrilees MJ, Flint MH. Ultrastructural study of tension and pressure zones in a rabbit flexor tendon. Am J Anat. 1980;157(1):87–106.
- Birk DE, Trelstad RL. Extracellular compartments in tendon morphogenesis: collagen fibril, bundle, and macroaggregate formation. J Cell Biol. 1986;103(1):231–240. doi:10.1083/jcb.103.1.231.
- Best KT, Loiselle AE. Scleraxis lineage cells contribute to organized bridging tissue during tendon healing and identify a subpopulation of resident tendon cells. FASEB J. 2019;33(7):8578–8587. doi:10.1096/fj.201900130RR.
- Schweitzer R, Chyung JH, Murtaugh LC, Brent AE, Rosen V, Olson EN, Lassar A, Tabin CJ. Analysis of the tendon cell fate using scleraxis, a specific marker for tendons and ligaments. Development. 2001;128(19):3855–3866.
- Delgado Caceres M, Pfeifer CG, Docheva D. Understanding tendons: lessons from transgenic mouse models. Stem Cells Dev. 2018;27(17):1161–1174. doi:10.1089/scd.2018.0121.
- Eadie AL, Titus AJ, Brunt KR. Getting to the heart of myofibroblast differentiation: implications for scleraxis in ECM remodeling and therapeutic targeting. Am J Physiol Heart Circ Physiol. 2018;315(5):H1232–H1235. doi:10.1152/ajpheart.00381.2018.
- Yin Z, Hu JJ, Yang L, Zheng ZF, An CR, Wu BB, Zhang C, Shen WL, Liu HH, Chen JL, Heng BC. Single-cell analysis reveals a nestin+ tendon stem/progenitor cell population with strong tenogenic potentiality. Sci Adv. 2016;2(11):e1600874. doi:10.1126/sciadv.1600874.
- Dakin SG, Martinez FO, Yapp C, Wells G, Oppermann U, Dean BJF, Smith RDJ, Wheway K, Watkins B, Roche L, Carr AJ. Inflammation activation and resolution in human tendon disease. Sci Transl Med. 2015;7(311):311ra173–311ra173. doi:10.1126/scitranslmed.aac4269.
- Swanson JB, De Micheli AJ, Disser NP, Martinez LM, Walker NR, Cosgrove BD, Mendias CL. A single-cell transcriptional atlas identifies extensive heterogeneity in the cellular composition of tendons. BioRXiv. 2019.
- Eliasson P, Andersson T, Hammerman M, Aspenberg P. Primary gene response to mechanical loading in healing rat Achilles tendons. J Appl Physiol (1985). 2013;114(11):1519–1526. doi:10.1152/japplphysiol.01500.2012.
- Arnoczky SP, Lavagnino M, Whallon JH, Hoonjan A. In situ cell nucleus deformation in tendons under tensile load; a morphological analysis using confocal laser microscopy. J Orthop Res. 2002;20(1):29–35. doi:10.1016/S0736-0266(01)00080-8.
- Andersson T, Eliasson P, Hammerman M, Sandberg O, Aspenberg P. Low-level mechanical stimulation is sufficient to improve tendon healing in rats. J Appl Physiol (1985). 2012;113(9):1398–1402. doi:10.1152/japplphysiol.00491.2012.
- Freedman BR, Rodriguez AB, Leiphart RJ, Newton JB, Ban E, Sarver JJ, Mauck RL, Shenoy VB, Soslowsky LJ. Dynamic loading and tendon healing affect multiscale tendon properties and ECM stress transmission. Sci Rep. 2018;8(1):10854. doi:10.1038/s41598-018-29060-y.
- Arnoczky SP, Lavagnino M, Egerbacher M. The mechanobiological aetiopathogenesis of tendinopathy: is it the over-stimulation or the under-stimulation of tendon cells? Int J Exp Pathol. 2007;88(4):217–226. doi:10.1111/j.1365-2613.2007.00548.x.
- Snedeker JG, Gautieri A. The role of collagen crosslinks in ageing and diabetes - the good, the bad, and the ugly. Muscles Ligaments Tendons J. 2014;4(3):303–308. doi:10.32098/mltj.03.2014.07.
- Nourissat G, Berenbaum F, Duprez D. Tendon injury: from biology to tendon repair. Nat Rev Rheumatol. 2015;11(4):223. doi:10.1038/nrrheum.2015.26.
- Killian ML, Cavinatto L, Galatz LM, Thomopoulos S. The role of mechanobiology in tendon healing. J Shoulder Elbow Surg. 2012;21(2):228–237. doi:10.1016/j.jse.2011.11.002.
- Lebaschi A, Deng X-H, Zong J, Cong G-T, Carballo CB, Album ZM, Camp C, Rodeo SA. Animal models for rotator cuff repair. Ann N Y Acad Sci. 2016;1383(1):43–57. doi:10.1111/nyas.2016.1383.issue-1.
- Bottagisio M, Lovati AB. A review on animal models and treatments for the reconstruction of Achilles and flexor tendons. J Mater Sci Mater Med. 2017;28(3):45. doi:10.1007/s10856-017-5858-y.
- Brehm MA, Shultz LD, Luban J, Greiner DL. Overcoming current limitations in humanized mouse research. J Infect Dis. 2013;208(Suppl 2):S125–30. doi:10.1093/infdis/jit319.
- Nunan R, Harding KG, Martin P. Clinical challenges of chronic wounds: searching for an optimal animal model to recapitulate their complexity. Dis Model Mech. 2014;7(11):1205–1213. doi:10.1242/dmm.016782.
- Marr C, McMillan I, Boyd JS, Wright NG, Murray M. Ultrasonographic and histopathological findings in equine superficial digital flexor tendon injury. Equine Vet J. 1993;25(1):23–29. doi:10.1111/evj.1993.25.issue-1.
- Muir P, Johnson K. Supraspinatus and biceps brachii tendinopathy in dogs. J Small Anim Pract. 1994;35(5):239–243. doi:10.1111/j.1748-5827.1994.tb03268.x.
- Smith MM, Sakurai G, Smith SM, Young AA, Melrose J, Stewart CM, Appleyard RC, Peterson JL, Gillies RM, Dart AJ, Sonnabend DH, Little CB. Modulation of aggrecan and ADAMTS expression in ovine tendinopathy induced by altered strain. Arthritis Rheum. 2008;58(4):1055–1066. doi:10.1002/art.23388.
- Hast M, Zuskov A, Soslowsky L. The role of animal models in tendon research. Bone Joint Res. 2014;3(6):193–202. doi:10.1302/2046-3758.36.2000281.
- Vigano M, Perucca Orfei C, Colombini A, Stanco D, Randelli P, Sansone V, de Girolamo L. Different culture conditions affect the growth of human tendon stem/progenitor cells (TSPCs) within a mixed tendon cells (TCs) population. J Exp Orthop. 2017;4(1):8. doi:10.1186/s40634-017-0082-8.
- Russell WMS, Burch RL, Hume CW. The principles of humane experimental technique. London (UK): Methuen; 1959.
- Kastelic J, Galeski A, Baer E. The multicomposite structure of tendon. Connect Tissue Res. 1978;6(1):11–23. doi:10.3109/03008207809152283.
- Robinson PS, Huang T-F, Kazam E, Iozzo RV, Birk DE, Soslowsky LJ. Influence of decorin and biglycan on mechanical properties of multiple tendons in knockout mice. J Biomech Eng. 2005;127(1):181–185. doi:10.1115/1.1835363.
- Sasaki N, Odajima S. Elongation mechanism of collagen fibrils and force-strain relations of tendon at each level of structural hierarchy. J Biomech. 1996;29(9):1131–1136. doi:10.1016/0021-9290(96)00024-3.
- Fessel G, Snedeker JG. Evidence against proteoglycan mediated collagen fibril load transmission and dynamic viscoelasticity in tendon. Matrix Biol. 2009;28(8):503–510. doi:10.1016/j.matbio.2009.08.002.
- Rigozzi S, Mueller R, Snedeker JG. Collagen fibril morphology and mechanical properties of the Achilles tendon in two inbred mouse strains. J Anat. 2010;216(6):724–731. doi:10.1111/j.1469-7580.2010.01225.x.
- Li Y, Fessel G, Georgiadis M, Snedeker JG. Advanced glycation end-products diminish tendon collagen fiber sliding. Matrix Biol. 2013;32(3–4):169–177. doi:10.1016/j.matbio.2013.01.003.
- Gautieri A, Passini FS, Silván U, Guizar-Sicairos M, Carimati G, Volpi P, Moretti M, Schoenhuber H, Redaelli A, Berli M, Snedeker JG. Advanced glycation end-products: mechanics of aged collagen from molecule to tissue. Matrix Biol. 2017; 59:95–108. doi:10.1016/j.matbio.2016.09.001.
- Szczesny SE, Elliott DM. Interfibrillar shear stress is the loading mechanism of collagen fibrils in tendon. Acta Biomater. 2014;10(6):2582–2590. doi:10.1016/j.actbio.2014.01.032.
- Thorpe CT, Riley GP, Birch HL, Clegg PD, Screen HRC. Effect of fatigue loading on structure and functional behaviour of fascicles from energy-storing tendons. Acta Biomater. 2014;10(7):3217–3224. doi:10.1016/j.actbio.2014.04.008.
- Szczesny SE, Aeppli C, David A, Mauck RL. Fatigue loading of tendon results in collagen kinking and denaturation but does not change local tissue mechanics. J Biomech. 2018;71:251–256. doi:10.1016/j.jbiomech.2018.02.014.
- Snedeker JG, Foolen J. Tendon injury and repair - A perspective on the basic mechanisms of tendon disease and future clinical therapy. Acta Biomater. 2017;63:18–36. doi:10.1016/j.actbio.2017.08.032.
- Schnabel LV, Mohammed HO, Miller BJ, McDermott WG, Jacobson MS, Santangelo KS, Fortier LA. Platelet rich plasma (PRP) enhances anabolic gene expression patterns in flexor digitorum superficialis tendons. J Orthop Res. 2007;25(2):230–240. doi:10.1002/(ISSN)1554-527X.
- Ikeda J, Zhao C, Moran SL, An K-N, Amadio PC. Effects of synovial interposition on healing in a canine tendon explant culture model. J Hand Surg Am. 2010;35(7):1153–1159. doi:10.1016/j.jhsa.2010.03.023.
- Costa-Almeida R, Berdecka D, Rodrigues MT, Reis RL, Gomes ME. Tendon explant cultures to study the communication between adipose stem cells and native tendon niche. J Cell Biochem. 2018;119(4):3653–3662. doi:10.1002/jcb.v119.4.
- Connizzo BK, Grodzinsky AJ. Release of pro-inflammatory cytokines from muscle and bone causes tenocyte death in a novel rotator cuff in vitro explant culture model. Connect Tissue Res. 2018;59:423–436. doi:10.1080/03008207.2018.1439486.
- Fessel G, Cadby J, Wunderli S, van Weeren R, Snedeker JG. Dose and time dependent effects of genipin cross-linking on cell viability and tissue mechanics - toward clinical application for tendon repair. Acta Biomater. 2014;10(5):1897–1906. doi:10.1016/j.actbio.2013.12.048.
- Abrahamsson SO, Lundborg G, Lohmander LS. Recombinant human insulin‐like growth factor‐I stimulates in vitro matrix synthesis and cell proliferation in rabbit flexor tendon. J Orthop Res. 1991;9(4):495–502. doi:10.1002/jor.1100090405.
- Yoshikawa Y, Abrahamsson SO. Dose-related cellular effects of platelet-derived growth factor-BB differ in various types of rabbit tendons in vitro. Acta Orthop Scand. 2001;72(3):287–292. doi:10.1080/00016470152846646.
- Wong MW, Lui WT, Chuen Fu S, Man Lee K. The effect of glucocorticoids on tendon cell viability in human tendon explants. Acta Orthop. 2009;80(3):363–367. doi:10.3109/17453670902988386.
- Wang T, Lin Z, Ni M, Thien C, Day RE, Gardiner B, Rubenson J, Kirk TB, Smith DW, Wang A, Lloyd DG. Cyclic mechanical stimulation rescues Achilles tendon from degeneration in a bioreactor system. J Orthop Res. 2015;33(12):1888–1896.
- Wang T, Lin Z, Day RE, Gardiner B, Landao-Bassonga E, Rubenson J, Kirk TB, Smith DW, Lloyd DG, Hardisty G, Wang A, Zheng Q, Zheng MH. Programmable mechanical stimulation influences tendon homeostasis in a bioreactor system. Biotechnol Bioeng. 2013;110(5):1495–1507. doi:10.1002/bit.v110.5.
- Adekanmbi I, Franklin S, Thompson MS. A novel in vitro loading system for high frequency loading of cultured tendon fascicles. Med Eng Phys. 2013;35(2):205–210. doi:10.1016/j.medengphy.2012.08.015.
- Abreu EL, Leigh D, Derwin KA. Effect of altered mechanical load conditions on the structure and function of cultured tendon fascicles. J Orthop Res. 2008;26(3):364–373. doi:10.1002/(ISSN)1554-527X.
- Pörtner R, Nagel-Heyer S, Goepfert C, Adamietz P, Meenen NM. Bioreactor design for tissue engineering. J Biosci Bioeng. 2005;100(3):235–245. doi:10.1263/jbb.100.235.
- Youngstrom DW, Rajpar I, Kaplan DL, Barrett JG. A bioreactor system for in vitro tendon differentiation and tendon tissue engineering. J Orthop Res. 2015;33(6):911–918. doi:10.1002/jor.22848.
- Hannafin JA, Arnoczky SP. Effect of cyclic and static tensile loading on water content and solute diffusion in canine flexor tendons: an in vitro study. J Orthop Res. 1994;12(3):350–356. doi:10.1002/(ISSN)1554-527X.
- Abousleiman RI, Reyes Y, McFetridge P, Sikavitsas V. Tendon tissue engineering using cell-seeded umbilical veins cultured in a mechanical stimulator. Tissue Eng Part A. 2008;15(4):787–795. doi:10.1089/ten.tea.2008.0102.
- Wunderli SL, Widmer J, Amrein N, Foolen J, Silvan U, Leupin O, Snedeker JG. Minimal mechanical load and tissue culture conditions preserve native cell phenotype and morphology in tendon-a novel ex vivo mouse explant model. J Orthop Res. 2018;36(5):1383–1390. doi:10.1002/jorr.v36.5.
- Hamilton B, Purdam C. Patellar tendinosis as an adaptive process: a new hypothesis. Br J Sports Med. 2004;38(6):758–761. doi:10.1136/bjsm.2003.005157.
- Almekinders LC, Weinhold PS, Maffulli N. Compression etiology in tendinopathy. Clin Sports Med. 2003;22(4):703–710. doi:10.1016/S0278-5919(03)00067-X.
- Szczesny SE, Caplan JL, Pedersen P, Elliott DM. Quantification of interfibrillar shear stress in aligned soft collagenous tissues via notch tension testing. Sci Rep. 2015;5:14649. doi:10.1038/srep14649.
- Carpenter JE, Flanagan CL, Thomopoulos S, Yian EH, Soslowsky LJ. The effects of overuse combined with intrinsic or extrinsic alterations in an animal model of rotator cuff tendinosis. Am J Sports Med. 1998;26(6):801–807. doi:10.1177/03635465980260061101.
- Lee AH, Elliott DM. Multi-scale loading and damage mechanisms of plantaris and rat tail tendons. J Orthop Res. 2019;37:1827–1837. doi:10.1002/jorr.v37.8.
- Szczesny SE, Fetchko KL, Dodge GR, Elliott DM. Evidence that interfibrillar load transfer in tendon is supported by small diameter fibrils and not extrafibrillar tissue components. J Orthop Res. 2017;35:2127–2134. doi:10.1002/jor.v35.10.
- Screen HR, Shelton JC, Chhaya VH, Kayser MV, Bader DL, Lee DA. The influence of noncollagenous matrix components on the micromechanical environment of tendon fascicles. Ann Biomed Eng. 2005;33(8):1090–1099. doi:10.1007/s10439-005-5777-9.
- Screen HR, Lee DA, Bader DL, Shelton JC. An investigation into the effects of the hierarchical structure of tendon fascicles on micromechanical properties. Proc Inst Mech Eng H. 2004;218(2):109–119. doi:10.1243/095441104322984004.
- Flick J, Devkota A, Tsuzaki M, Almekinders L, Weinhold P. Cyclic loading alters biomechanical properties and secretion of PGE2 and NO from tendon explants. Clin Biomech (Bristol, Avon). 2006;21(1):99–106. doi:10.1016/j.clinbiomech.2005.08.008.
- Devkota AC, Tsuzaki M, Almekinders LC, Banes AJ, Weinhold PS. Distributing a fixed amount of cyclic loading to tendon explants over longer periods induces greater cellular and mechanical responses. J Orthop Res. 2007;25(8):1078–1086. doi:10.1002/(ISSN)1554-527X.
- Thorpe CT, Chaudhry S, Lei II, Varone A, Riley GP, Birch HL, Clegg PD, Screen HRC. Tendon overload results in alterations in cell shape and increased markers of inflammation and matrix degradation. Scand J Med Sci Sports. 2015;25(4):e381–391.
- Spiesz EM, Thorpe CT, Chaudhry S, Riley GP, Birch HL, Clegg PD, Screen HRC. Tendon extracellular matrix damage, degradation and inflammation in response to in vitro overload exercise. J Orthop Res. 2015;33(6):889–897. doi:10.1002/jor.22879.
- Legerlotz K, Jones GC, Screen HRC, Riley GP. Cyclic loading of tendon fascicles using a novel fatigue loading system increases interleukin-6 expression by tenocytes. Scand J Med Sci Sports. 2013;23(1):31–37. doi:10.1111/sms.2013.23.issue-1.
- Legerlotz K, Jones ER, Screen HRC, Riley GP. Increased expression of IL-6 family members in tendon pathology. Rheumatology (Oxford). 2012;51(7):1161–1165. doi:10.1093/rheumatology/kes002.
- Jones GC, Corps AN, Pennington CJ, Clark IM, Edwards DR, Bradley MM, Hazleman BL, Riley GP. Expression profiling of metalloproteinases and tissue inhibitors of metalloproteinases in normal and degenerate human achilles tendon. Arthritis Rheum. 2006;54(3):832–842. doi:10.1002/(ISSN)1529-0131.
- Hannafin JA, Arnoczky SP, Hoonjan A, Torzilli PA. Effect of stress deprivation and cyclic tensile loading on the material and morphologic properties of canine flexor digitorum profundus tendon: an in vitro study. J Orthop Res. 1995;13(6):907–914. doi:10.1002/(ISSN)1554-527X.
- Gardner K, Arnoczky SP, Caballero O, Lavagnino M. The effect of stress-deprivation and cyclic loading on the TIMP/MMP ratio in tendon cells: an in vitro experimental study. Disabil Rehabil. 2008;30(20–22):1523–1529. doi:10.1080/09638280701785395.
- Thornton GM, Shao X, Chung M, Sciore P, Boorman RS, Hart DA, Lo IKY. Changes in mechanical loading lead to tendonspecific alterations in MMP and TIMP expression: influence of stress deprivation and intermittent cyclic hydrostatic compression on rat supraspinatus and Achilles tendons. Br J Sports Med. 2010;44(10):698–703. doi:10.1136/bjsm.2008.050575.
- Wunderli S, Blache U, Piccoli AB, Niederoest B, Holenstein CN, Passini F, Silvan U, Bundgaard L, Auf Dem Keller U, Snedeker JG. Tendon response to matrix unloading is determined by the patho-physiological niche. Matrix Biol. 2019. doi:10.1016/j.matbio.2019.12.003.
- Shepherd JH, Riley GP, Screen HR. Early stage fatigue damage occurs in bovine tendon fascicles in the absence of changes in mechanics at either the gross or micro-structural level. J Mech Behav Biomed Mater. 2014;38:163–172. doi:10.1016/j.jmbbm.2014.06.005.
- Rigby BJ, Hirai N, Spikes JD, Eyring H. The mechanical properties of rat tail tendon. J Gen Physiol. 1959;43(2):265–283. doi:10.1085/jgp.43.2.265.
- Robinson PS, Lin TW, Jawad AF, Iozzo RV, Soslowsky LJ. Investigating tendon fascicle structure–function relationships in a transgenic-age mouse model using multiple regression models. Ann Biomed Eng. 2004;32(7):924–931. doi:10.1023/B:ABME.0000032455.78459.56.
- Fessel G, Snedeker JG. Equivalent stiffness after glycosaminoglycan depletion in tendon — an ultra-structural finite element model and corresponding experiments. J Theor Biol. 2011;268(1):77–83. doi:10.1016/j.jtbi.2010.10.007.
- Arnoczky S, Tian T, Lavagnino M, Gardner K. Ex vivo static tensile loading inhibits MMP-1 expression in rat tail tendon cells through a cytoskeletally based mechanotransduction mechanism. J Orthop Res. 2004;22(2):328–333. doi:10.1016/S0736-0266(03)00185-2.
- Lavagnino M, Arnoczky SP, Egerbacher M, Gardner KL, Burns ME. Isolated fibrillar damage in tendons stimulates local collagenase mRNA expression and protein synthesis. J Biomech. 2006;39(13):2355–2362. doi:10.1016/j.jbiomech.2005.08.008.
- Stauber T, Blache U, Snedeker JG. Tendon tissue microdamage and the limits of intrinsic repair. Matrix Biol. 2019. doi:10.1016/j.matbio.2019.07.008.
- Lavagnino M, Brooks AE, Oslapas AN, Gardner KL, Arnoczky SP. Crimp length decreases in lax tendons due to cytoskeletal tension, but is restored with tensional homeostasis. J Orthop Res. 2017;35(3):573–579. doi:10.1002/jor.v35.3.
- Gardner K, Lavagnino M, Egerbacher M, Arnoczky SP. Re-establishment of cytoskeletal tensional homeostasis in lax tendons occurs through an actin-mediated cellular contraction of the extracellular matrix. J Orthop Res. 2012;30(11):1695–1701. doi:10.1002/jor.v30.11.
- Lavagnino M, Arnoczky SP, Gardner K. In situ deflection of tendon cell-cilia in response to tensile loading: an in vitro study. J Orthop Res. 2011;29(6):925–930. doi:10.1002/jor.v29.6.
- Jafari L, Savard M, Gobeil F, Langelier E. Characterization of moderate tendinopathy in ex vivo stress-deprived rat tail tendons. Biomed Eng Online. 2019;18(1):54. doi:10.1186/s12938-019-0673-y.
- Cousineau-Pelletier P, Langelier E. Relative contributions of mechanical degradation, enzymatic degradation, and repair of the extracellular matrix on the response of tendons when subjected to under- and over- mechanical stimulations in vitro. J Orthop Res. 2010;28(2):204–210. doi:10.1002/jor.20982.
- Choi R, Smith M, Clarke E, Little C. Cellular, matrix, and mechano-biological differences in load-bearing versus positional tendons throughout development and aging: a narrative review. Connect Tissue Res. 2018;59(5):483–494. doi:10.1080/03008207.2018.1504929.
- Maganaris CN, Paul JP. In vivo human tendon mechanical properties. J Physiol. 1999;521(1):307–313. doi:10.1111/tjp.1999.521.issue-1.
- Wren TA, Yerby SA, BeauprÈ GS, Carter DR. Mechanical properties of the human achilles tendon. Clin Biomech. 2001;16(3):245–251. doi:10.1016/S0268-0033(00)00089-9.
- Arble JR, Lalley AL, Dyment NA, Joshi P, Shin D-G, Gooch C, Grawe B, Rowe D, Shearn JT. The LG/J murine strain exhibits near-normal tendon biomechanical properties following a full-length central patellar tendon defect. Connect Tissue Res. 2016;57(6):496–506. doi:10.1080/03008207.2016.1213247.
- Heinemeier KM, Schjerling P, Heinemeier J, Magnusson SP, Kjaer M. Lack of tissue renewal in human adult Achilles tendon is revealed by nuclear bomb (14)C. FASEB J. 2013;27(5):2074–2079. doi:10.1096/fj.12-225599.
- Heinemeier KM, Schjerling P, Øhlenschlæger TF, Eismark C, Olsen J, Kjær M. Carbon-14 bomb pulse dating shows that tendinopathy is preceded by years of abnormally high collagen turnover. FASEB J. 2018;32(9):4763–4775. doi:10.1096/fj.201701569R.
- Grinstein M, Dingwall HL, O’Connor LD, Zou K, Capellini TD, Galloway JL. A distinct transition from cell growth to physiological homeostasis in the tendon. eLife. 2019;8:e48689. doi:10.7554/eLife.48689.
- Arnoczky SP, Lavagnino M, Egerbacher M, Caballero O, Gardner K. Matrix metalloproteinase inhibitors prevent a decrease in the mechanical properties of stress-deprived tendons: an in vitro experimental study. Am J Sports Med. 2007;35(5):763–769. doi:10.1177/0363546506296043.
- Garner W, McDonald JA, Kuhn C, Weeks PM. Autonomous healing of chicken flexor tendons in vitro. J Hand Surg Am. 1988;13(5):697–700. doi:10.1016/S0363-5023(88)80127-8.
- Abrahamsson S-O, Lundborg G, Lohmander LS. Tendon healing in vivo: an experimental model. Scand J Plast Reconstr Surg. 1989;23(3):199–205. doi:10.3109/02844318909075118.
- Screen HR, Shelton JC, Bader DL, Lee DA. Cyclic tensile strain upregulates collagen synthesis in isolated tendon fascicles. Biochem Biophys Res Commun. 2005;336(2):424–429. doi:10.1016/j.bbrc.2005.08.102.
- Usmani S, Aurand ER, Medelin M, Fabbro A, Scaini D, Laishram J, Rosselli FB, Ansuini A, Zoccolan D, Scarselli M, De Crescenzi M, Bosi S, Prato M, Ballerini L. 3D meshes of carbon nanotubes guide functional reconnection of segregated spinal explants. Sci Adv. 2016;2(7):e1600087. doi:10.1126/sciadv.1600087.
- Imler SM, Doshi AN, Levenston ME. Combined effects of growth factors and static mechanical compression on meniscus explant biosynthesis. Osteoarthritis Cartilage. 2004;12(9):736–744. doi:10.1016/j.joca.2004.05.007.
- Wang X, Zhang J, Li G, Sai N, Han J, Hou Z, Kachelmeier A, Shi X. Vascular regeneration in adult mouse cochlea stimulated by VEGF-A165 and driven by NG2-derived cells ex vivo. Hear Res. 2019;377:179–188. doi:10.1016/j.heares.2019.03.010.
- Maeda T, Sakabe T, Sunaga A, Sakai K, Rivera AL, Keene DR, Sasaki T, Stavnezer E, Iannotti J, Schweitzer R, Ilic D. Conversion of mechanical force into TGF-beta-mediated biochemical signals. Curr Biol. 2011;21(11):933–941. doi:10.1016/j.cub.2011.04.007.
- Lavagnino M, Arnoczky SP, Tian T, Vaupel Z. Effect of amplitude and frequency of cyclic tensile strain on the inhibition of MMP-1 mRNA expression in tendon cells: an in vitro study. Connect Tissue Res. 2003;44(3–4):181–187. doi:10.1080/03008200390215881.
- Spiesz EM, Thorpe CT, Thurner PJ, Screen HR. Structure and collagen crimp patterns of functionally distinct equine tendons, revealed by quantitative polarised light microscopy (qPLM). Acta Biomater. 2018;70:281–292. doi:10.1016/j.actbio.2018.01.034.
- Shepherd JH, Legerlotz K, Demirci T, Klemt C, Riley GP, Screen HR. Functionally distinct tendon fascicles exhibit different creep and stress relaxation behaviour. Proc Inst Mech Eng H. 2014;228(1):49–59. doi:10.1177/0954411913509977.
- Lavagnino M, Brooks AE, Oslapas AN, Gardner KL, Arnoczky SP. Crimp length decreases in lax tendons due to cytoskeletal tension, but is restored with tensional homeostasis. J Orthop Res. 2017;35(3):573–579. doi:10.1002/jor.23489.
- Camp RJ, Liles M, Beale J, Saeidi N, Flynn BP, Moore E, Murthy SK, Ruberti JW. Molecular mechanochemistry: low force switch slows enzymatic cleavage of human type I collagen monomer. J Am Chem Soc. 2011;133(11):4073–4078. doi:10.1021/ja110098b.
- Ruberti JW, Hallab NJ. Strain-controlled enzymatic cleavage of collagen in loaded matrix. Biochem Biophys Res Commun. 2005;336(2):483–489. doi:10.1016/j.bbrc.2005.08.128.
- Saini K, Cho S, Dooling LJ, Discher DE. Tension in fibrils suppresses their enzymatic degradation - A molecular mechanism for ‘use it or lose it’. Matrix Biol. 2019. doi:10.1016/j.matbio.2019.06.001.
- Carreau A, Hafny-Rahbi BE, Matejuk A, Grillon C, Kieda C. Why is the partial oxygen pressure of human tissues a crucial parameter? Small molecules and hypoxia. J Cell Mol Med. 2011;15(6):1239–1253. doi:10.1111/jcmm.2011.15.issue-6.
- Kubo K, Ikebukuro T, Tsunoda N, Kanehisa H. Noninvasive measures of blood volume and oxygen saturation of human Achilles tendon by red laser lights. Acta Physiol (Oxf). 2008;193(3):257–264. doi:10.1111/j.1748-1716.2008.01841.x.
- Avery N, Bailey A. Restraining cross-links responsible for the mechanical properties of collagen fibers: natural and artificial. In: Fratzl P, editor. Collagen. Boston (MA): Springer; 2008. p. 81–110.
- Makris EA, Responte DJ, Paschos NK, Hu JC, Athanasiou KA. Developing functional musculoskeletal tissues through hypoxia and lysyl oxidase-induced collagen cross-linking. Proc Natl Acad Sci U S A. 2014;111(45):E4832–41. doi:10.1073/pnas.1414271111.