ABSTRACT
Purpose/Aim of the study
Osteogenesis imperfecta is a heritable bone disorder that is usually caused by mutations in collagen type I encoding genes. The impact of such mutations on tendons, a structure with high collagen type I content, remains largely unexplored. We hypothesized that tendon properties are abnormal in the context of a mutation affecting collagen type I. The main purpose of the study was to assess the anatomical, mechanical, and material tendon properties of Col1a1Jrt/+ mice, a model of severe dominant OI.
Materials and Methods
The Flexor Digitorum Longus (FDL) tendon of Col1a1Jrt/+ mice and wild-type littermates (WT) was assessed with in vitro mechanical testing.
Results
The results showed that width and thickness of FDL tendons were about 40% larger in WT (p < 0.01) than in Col1a1Jrt/+ mice, whereas the cross-sectional area was 138% larger (p < 0.001). The stiffness, peak- and yield-force were between 160% and 194% higher in WT vs. Col1a1Jrt/+ mice. The material properties did not show significant differences between mouse strains with differences <15% between WT and Col1a1Jrt/+ (p > 0.05). Analysis of the Achilles tendon collagen showed no difference between mice strains for the content but collagen solubility in acetic acid was 66% higher in WT than in Col1a1Jrt/+ (p < 0.001).
Conclusions
This study shows that the FDL tendon of Col1a1Jrt/+ mice has reduced mechanical properties but apparently normal material properties. It remains unclear whether the tendon phenotype of Col1a1Jrt/+ mice is secondary to muscle weakness or a direct effect of the Col1a1 mutation or a combination of both.
Introduction
Osteogenesis imperfecta (OI) is a heritable bone disorder that is usually caused by mutations in collagen type I encoding genes {Misof, 1997 #1688;McBride, 1997 #1689}. To study osteogenesis imperfecta at the molecular and cellular level, at least 20 mouse models of OI have been generatedCitation1. Those models have allowed assessing some aspects of OI that are overlooked or harder to investigate in a human populationCitation2–5. For example, our research group have recently shown that compared to WT, Col1a1Jrt/+ mice display numerous changes in muscle tissue gene expressionCitation6, composition Citation7 and functionCitation8.
In recent years, research has shown that there is a strong relationship between muscles and bones which led to the development of a whole new field of research on the functional muscle-bone unit. As such, research in OI mouse models aiming at elucidating the origin of muscle weakness has mainly focused on the muscle itself or on mediating factors coming from the bone crosstalk with muscleCitation9,Citation10. At present, no study has brought strong conclusions on the origin of muscle weaknesses. Tendons connect bone and muscle and are known for their importance for transmitting muscle contraction forces to the bones to generate motion, and have a high collagen type I content. However, very little is known regarding the mechanical, material, and anatomical properties of tendons in OI.
Three pioneering studies from 1997 showed the first evidence of a tendon phenotype in OI mice models, both related to the homotrimeric configuration of the collagen in the OIM mouse modelCitation11,Citation12. Electron microscopy showed decreased axial packing of collagen fibrils in the absence of the collagen alpha 2 chainCitation12, suggesting disorganization of the tendon matrix. Further studies showed that collagen type I was degrading at a lower temperature in OIM mice than in WT mice, possibly due to decreased cross-linking of collagen fibrilsCitation13. Finally, material properties (stress/strain failure) were lower (half the values of WT) in OIM/OIM mice than in OIM/+ mice, both being lower than the WTCitation11.
While there is evidence of a tendon phenotype the OIM model, there is a scarcity of data from Col1a1Jrt/+ mice. The initial description of these mice reported that Col1a1Jrt/+ tendon were “more frayed” than WTCitation14, but no detailed information on tendon material or mechanical properties was provided. The pertinence of assessing tendon properties in Col1a1Jrt/+ mice is that the alpha-2 chain seems to provide a distinct structural function compared to the alpha-1 chainCitation15,Citation16. The OIM mouse model lacks an alpha-2 chain resulting in a homotrimeric collagen configuration with three alpha-1 chains in contrast to a normal heterotrimetric configuration (two alpha-1 and one alpha-2 chain). In contrast, the Col1a1Jrt/+ model has a heterotrimeric configuration with normal alpha-2 chain and mutated alpha-1 chain. Therefore, given the different functions of alpha-1 and alpha-2 chains, it is possible that the tendon properties will differ in these mouse models. However, one needs to keep in mind that given that the Col1a1 mutation is not tissue specific, this study will not ascertain that abnormalities in tendon properties are the main contributor to muscle weakness observed in this mouse model or that tendon abnormalities are secondary to the muscle defect previously reportedCitation8. This study thus aims at describing the tendon phenotype in a mouse model of OI carrying a Col1a1 mutation.
To determine the effect of a Col1a1 mutation on tendon properties, we used the Col1a1Jrt/+ mouse. This mouse model of OI carries a unique mutation which causes a T to C transition at the exon 9 splice donor site which results in the skipping of exon 9 and an 18-amino acid deletion within the N-terminal region of the triple helical domain of Col1a1Citation14,Citation17,Citation18. In the present study, we hypothesized that the anatomical, mechanical, and material properties of the flexor digitorum longus (FDL) tendon of Col1a1Jrt/+ mice would differ from WT mice due to the compromised architecture of the collagen type I molecule.
Materials and methods
Mice
All experiments were approved by the Animal Care Committee at McGill University and conformed to the ethical guidelines of the Canadian Council on Animal Care. Female Col1a1Jrt/+ mice on FVB background were developed by screening of N-ethyl-N-nitrosourea-induced mutagenesis which is generated by a base transition (T to C) mutation at the exon nine splice donor site resulting in an 18 amino acid deletion in Col1a1Citation14. Col1a1Jrt/+ mice were obtained as describedCitation9. The breeding colony was maintained at the Animal Care Facility of the Shriners Hospitals for Children-Canada. Animals were housed in groups of up to 5 animals on a 12-h alternating light and dark cycle and had unrestricted access to water and food (Charles River rodent diet 5075, Charles River Laboratories, Wilmington, MA, USA).
These mice were euthanized at 14 weeks of age. Blood samples were collected at euthanasia by intracardiac puncture. Both hind limbs were dissected, snap-frozen and kept at −80°C until all the materials were collected and sent for analysis.
Mechanical testing procedure
The FDL tendons were dissected, including the proximal aponeurosis and distal digital branches to ensure maximal surface area for clamping. Each end of the FDL tendon was allowed to air dry at room temperature, while the mid-portion was covered with gauze soaked in phosphate buffered saline (PBS) to keep it hydrated. Subsequently, the dried tendon fascicle ends were fastened to the clamps of a custom-made Deben mechanical rig (20N tensile stage, Petri dish version, Deben Ltd, Stuffolk, UK) with cyanoacrylate glue. The glue was allowed to cure and then the sample was transferred to the test stage. The Deben software was used to load the tissue under “deformation” control. To get rid of any original slack before testing, a force of 0.1N was applied to the tendon and the length, width, and thickness (using a 45° mirror) of the tendon was measured on images acquired through a dissecting microscope (Microscope: Nikon SMZ1000, Camera: Olympus SC50). The mechanical testing protocol consisted of five preconditioning cycles, a 60 s stress-relaxation test, and a failure test. Preconditioning was essential because the tendons had been thawed from −80°C and this procedure could modify the fiber alignment. Preconditioning and stress-relaxation were performed at 3% strain. Deformation rate was 4 mm/second and sampling rate was 10 Hz throughout the tests. Values were normalized with tendon dimensions to generate stress—strain graphs using Excel® (Microsoft®, WA, USA). Mechanical properties, including peak force (N), yield force (N) and peak stiffness (N/mm), that are dependent on tissue dimensions and material properties, such as peak stress (MPa), yield stress (MPa) and Young’s modulus (MPa), that are independent of tissue dimensions were analyzedCitation19. In addition, the relative amount of stress-relaxation (%) after 60 s at 3% strain was analyzed. The anatomical properties that were measured include width (µm), thickness (µm) and cross-sectional area (mm2).
Collagen content and acid solubility
Following mechanical testing, the FDL tendon was cut from the testing machine and the collagen content was determined by measurement of hydroxyproline as previously describedCitation20. In brief, the tissue was freeze-dried, weighed, and hydrolyzed in 6 M HCl at 110°C overnight. The hydrolyzate was dried under nitrogen, washed with distilled water and dried again before reconstituting in distilled water. Hydroxyproline was determined colorimetrically by reaction with 4-dimethyl-aminobenzaldehyde. Collagen content was determined by assuming a hydroxyproline content of 13.3% by weight.
As an indicator of collagen cross-link maturity, acid solubility of the free Achilles tendon was determined. Different types of collagen cross-links exist, which can be broadly categorized into three groups, immature enzymatic (lysine derived), mature enzymatic (hydroxylysine derived) and non-enzymaticCitation21. The immature enzymatic cross-links are labile to acetic acid while the mature enzymatic and non-enzymatic are mostly stabile, acid solubility therefore does not indicate the total amount of cross-linking but rather the level of cross-link maturity. The free Achilles tendon was dissected 1 mm away from its muscle and bone insertions to ensure pure tendon tissue. Transverse incisions were made into the tendon to increase surface area, then the tissue was freeze-dried and weighed before being extracted in 1 mL of 50 mM acetic acid for 24 h at 5°C with mild agitation. The solubilized sample was centrifuged at 21,000 rcf for 1 h at 5°C, and 0.5 mL of supernatant was taken to determine the soluble fraction. The remaining 0.5 mL of supernatant was left with the insoluble fraction because it forms an extended gel rather than a dense pellet. To each fraction was added 0.5 mL of 12 M HCl (6 M final concentration) and they were hydrolyzed for 20 h at 110°C. Hydrolysates were dried and assessed for collagen content as described above. Solubility was calculated as twice the collagen content of the supernatant (because only half the supernatant was taken) divided by the total collagen content of the supernatant and pellet.
Statistical analyses
All data are shown as mean ± SEM in this report. Differences between WT and OI groups were assessed using Student t-test (GraphPad Prism Software for Microsoft, San Diego, CA, USA). Significance is shown by symbols: * p < 0.05, ** p < 0.01, ***p < 0.001, ns: not significant (p ≥ 0.05).
Results
Qualitative observation of tendons
During the experiments, it was observed that differences in tendons between the Col1a1Jrt/+ mouse ( right panel) and the WT mouse (, left panel) were visually apparent. The tendons of the Col1a1Jrt/+ mouse exhibited a more transparent appearance and were smaller to the point that they in some cases were almost impossible to identify, whereas the WT mouse exhibited the normal white color and were easily distinguishable.
Mice flexor digitorum longus (FDL) tendon fascicles
Anatomical and compositional properties (): Tendon fascicle width was 47% larger in the WT mice group (564 ± 9 µm) compared to the Col1a1Jrt/+ mice group (383 ± 12 µm) (). Tendon thickness was 43% higher in the WT mice (304 ± 11 µm) than the Col1a1Jrt/+ mice (212 ± 10 µm) (). The tendon cross-sectional area was 100% greater in the WT mice (0.14 ± 0.01 mm2) than the Col1a1Jrt/+ mice (0.07 ± 0.01 mm2) (). The collagen content of WT mice (85 ± 2%) was not significantly different from that of Col1a1Jrt/+ mice (79 ± 4%) ().
Figure 2. Anatomical and compositional properties of the FDL tendons in WT (black bars; n= 10) and Col1A1Jrt mice (white bars; n = 10). Results are presented as mean and SEM. Panel A. Tendon width; Panel B. Tendon thickness; Panel C. Tendon cross-sectional area; Panel D. Collagen content relative to dry weight. *** indicates significance at the <0.001 level, ns: non-significant at >0.05.
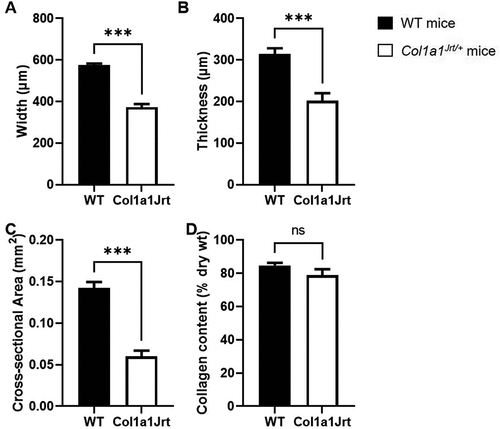
Mechanical properties (): Peak tendon force was 196% greater in the WT mice (11.9 ± 0.3 N) than the Col1a1Jrt/+ mice (4.0 ± 0.3 N) (). Force at the yield point was 160% greater in WT mice (8.3 ± 0.3 N) than Col1a1Jrt/+ mice (3.2 ± 0.2 N) (). Peak tendon stiffness was 178% greater in the WT mice (16.4 ± 0.2 N/mm) than the Col1a1Jrt/+ mice (5.9 ± 0.2 N/mm) ().
Figure 3. Mechanical properties of the FDL tendons in WT (black bars; n=10) and Col1A1Jrt mice (white bars; n=10). Results are presented as mean and SEM. Panel A. Tendon peak force; Panel B. Tendon yield force; Panel C. Peak stiffness. *** indicates significance at the <0.001 level.
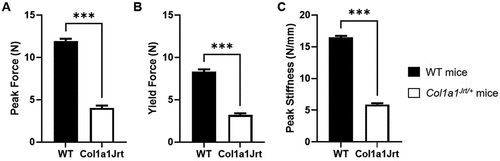
Material properties (): Peak tendon stress in the WT mice (85 ± 4 MPa) was not significantly different from the Col1a1Jrt/+ mice (74 ± 8 MPa) (). Tendon yield stress in WT mice (59 ± 3 MPa) did not significantly differ from the Col1a1Jrt/+ mice (59 ± 7 MPa) (). Tendon Young’s modulus of WT mice (938 ± 45 MPa) was not significantly different from that of Col1a1Jrt/+ mice (874 ± 92 MPa) (). The relative stress-relaxation after 60 s was 13% greater in the WT mice (16.0 ± 0.4%) than the Col1a1Jrt/+ mice (14.1 ± 0.5%) (). Note that the initial stress at 3% strain in the relaxation test did not differ between the WT (20.1 ± 0.9 MPa) and Col1a1Jrt/+ mice (21.5 ± 2.3%).
Figure 4. Material properties of the FDL tendons in WT (black bars; n=10) and Col1A1Jrt mice (white bars; n=10). Results are presented as mean and SEM. Panel A. Tendon peak stress; Panel B. Tendon yield stress; Panel C. Tendon Young’s modulus. Panel D. Relative stress-relaxation at 60 s. ** indicates significance at the <0.01 level, ns: non-significant at >0.05.
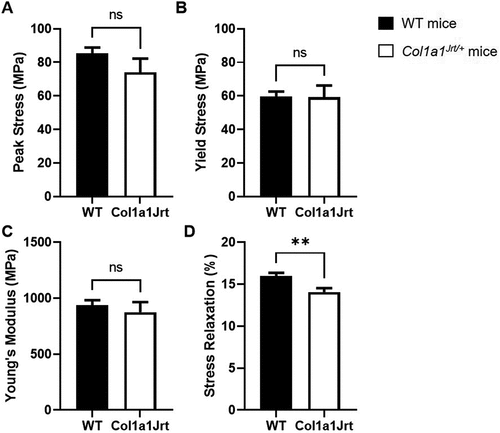
Mice achilles tendons
Composition and acid solubility (): Achilles tendon dry weight was 189% greater in the WT mice (390 ± 14 µg) than the Col1a1Jrt/+ mice (129 ± 5 µg). There were no differences in water content between WT (73 ± 1%) and Col1a1Jrt/+ (74 ± 1%) mice. Achilles tendon collagen content did not differ between WT (88 ± 2%) and Col1a1Jrt/+ (88 ± 2%) mice either. Acid solubility in acetic acid was 66% greater in the WT (2.0 ± 0.1%) compared to the Col1a1Jrt/+ group (1.2 ± 0.1%).
Figure 5. Composition and solubility of the Achilles tendon in WT (black bars; n = 10) and Col1A1Jrt mice (white bars; n = 10). Results are presented as mean and SEM. Panel A. Tendon dry weight; Panel B. Tendon water content; Panel C. Collagen content relative to dry weight; Panel D. Collagen solubility in acetic acid as a percent of total collagen. *** indicates significance at the <0.001 level, ns: non-significant at >0.05.
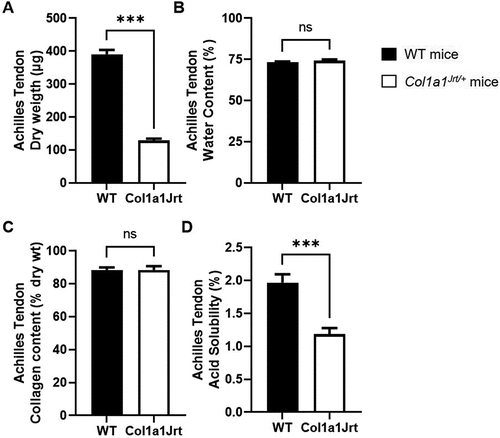
Discussion
This study’s primary aim was to quantify the FDL tendon’s anatomical, mechanical, and material properties in a mouse model of severe OI, Col1a1Jrt/+ mice. We hypothesized that the anatomical, mechanical, and material properties of the FDL tendon of Col1a1Jrt/+ mice would be lower, considering the 20% to 25% reduced body size of the Col1a1Jrt/+ mice Citation17 and the negatively impacted collagen type I molecule as a result of the Col1a1 mutation. Our primary hypothesis was partially supported as the anatomical and mechanical properties of the FDL tendons of Col1a1Jrt/+ mice were significantly lower than those of WT mice. Surprisingly, material properties (peak stress, yield stress, and Young’s modulus) were not significantly different between the Col1a1Jrt/+ and WT mice, although modest reductions in viscoelasticity (stress-relaxation) hint at possible subtle changes. Thus, even with severely abnormal collagen type I, the functional tendon structure is achieved in Col1a1Jrt/+ mice.
Consistent with a previous study in the homozygous OIM mouse modelCitation13, we report lower tendon mechanical properties for the Col1a1Jrt/+ mice. Peak force, yield force and peak stiffness were all lower in Col1a1Jrt/+ FDL tendon compared to WT. The lack of significant difference in material properties (peak stress, yield stress and Young’s modulus) between the FDL tendons of Col1a1Jrt/+ and WT mice was surprising. This observation is further supported by the smaller size but equal relative collagen content in the two groups for both the FDL and the Achilles tendons. The deletion of 18 amino acids (helix residue 37–54) in the Col1a1Jrt molecule would be expected to cause misalignment of the cross-link sites, which could reduce formation of stabile cross-links. In contrast, a reduction in acid solubility of the Achilles tendon in Col1a1Jrt/+ mice was observed, indicating increased stabile cross-linking, which, assuming that cross-linking is similar in Achilles and FDL tendons, could possibly explain the reduced stress relaxation observed. However, since acid solubility was very low in both groups the difference may have been too small to affect the stress and modulus. It is difficult to say why stabile cross-linking would be increased in Col1a1Jrt/+ mice, but it can be speculated that the majority of mutant collagen molecules may not be incorporated in the final tendon structure, leaving a matrix of mostly normal quality but reduced quantity. Greater levels of stabile cross-linking could then be a compensatory mechanism for the lower tissue quantity. It is important to note that the collagen content measurements were not specific to collagen type I and, therefore, there could have been an increase in collagen type III to compensate a possible lack of collagen type I. However, a major shift in composition would likely have affected material properties and the fact that there were no significant changes in these suggests that it was not the case.
Based on the observation that mechanical properties were lower in Col1a1Jrt/+mice but not the material properties, one could be tempted to conclude that the tendons are simply adapted to the smaller animal size with no functional impact. The Col1a1Jrt/+mice tendons were around 50% smaller in terms of cross-sectional area (the parameter used to normalize the material properties) and previous studies in Col1a1Jrt/+mice showed that body mass is roughly 25% lower in Col1a1Jrt/+mice compared to WTCitation14. Therefore, given that the difference in body mass is less than the difference in tendon size/mechanics, then a functional deficit becomes a possibility as the tendon will be carrying proportionally more loads in the Col1a1Jrt/+ mice compared to the WT.
The finding of normal material properties in the Col1a1Jrt/+ animals is not consistent with the lower stress/strain slope (Young’s modulus) observed in the homozygous OIM/OIM mice tail tendons, which demonstrate that the material properties of the collagen of tail tendons were negatively affected in OIM miceCitation11. A potential hypothesis explaining the lower material properties observed in the OIM mice but not in the Col1a1Jrt/+ mice might lie in the mutations found in each mouse strain. Whereas the OIM/OIM have a homotrimeric (only the α1(I) chain is present) configuration of its collagen, the Col1a1Jrt/+ have collagen molecules with a heterotrimeric configuration and carries a mutation in the α1(I) chain, whereas the α2(I) chain is normal. The material properties of a tendon might be negatively affected in the OIM mice because the absence of the α2(I) chain might cause the collagen type I molecule to lose its ability to confer mechanical strength and rigidity to the tendon fiber. In a previous study, it was shown that the presence of a mutated α2(I) chain results in a nonfunctional proα2(I) chain that cannot covalently bind to the other two proα1(I) chains, hindering the formation of the heterotrimeric collagen type I moleculeCitation16. This phenomenon potentially occurs because the mutation in OIM mice is found in the C-propeptide of the α2(I) chain, which plays a critical role in determining the correct alpha chain association during the trimerization and folding of the helical moleculeCitation22. Simply stated, these results suggest that affecting the molecular assembly, either by a mutation of the Col1a2 C-propeptide or complete absence of Col1a2, may result in a more structurally abnormal tendon phenotype than a mutation in the triple helical region of Col1a1, in spite of the heterozygous a1 chain mutation statistically being present in a larger fraction of the assembled molecules (75%) than a heterozygous a2 chain mutation would (50%).
The observation that material properties of tendons are mostly normal in Col1a1Jrt/+ mice contrasts with findings in bone, where material properties, such as ultimate stress, toughness and modulus and failure strain are clearly abnormal in Col1a1Jrt/+ miceCitation14. However, abnormal material properties in bone may reflect abnormal mineralization as the bone matrix is hypermineralized in Col1a1Jrt/+ miceCitation23, whereas tendon tissue is unmineralized.
Limitations
Given that tendon deficits is a fairly new research interest in OI mice models, there is not enough data to support the use of this model to determine tendon defects. Therefore, this work mainly shows the phenotypic alterations in biomechanical properties of the FDL tendon in a severe OI mouse model. Although this provides new insights into tendons in OI, the generalizability of the findings is limited to this specific mouse model. Results from mice studies are not generalizable to humans. For example, two recent studies aimed at evaluating the effect of voluntary wheel running training in two different OI mouse model strains and showed that OI mice muscles did not benefit from such exercise training. On the contrary, the only human study in which exercise training was performed by OI patients, showed good response. Thus, studies evaluating tendon in patients with OI are required to determine if a tendon phenotype is also present in humans, and may become a therapeutic target.
Conclusion
This study showed that the FDL tendon of Col1a1Jrt/+ mice has reduced mechanical properties but apparently normal material properties. At this point, it remains unclear whether the tendon phenotype of Col1a1Jrt/+ mice is secondary to muscle weakness or a direct effect of the Col1a1 mutation or a combination of both.
Acknowledgements
We would like to thank Shriners International and Precithera who supported this work.
Disclosure statement
No potential conflict of interest was reported by the author(s).
Larissa Sinkam: None
Iris Boraschi-Diaz: None
Svetlana Komarova: None
René Svensson: None
Michael Kjaer: None
Raynald Bergeron: None
Frank Rauch: PreciThera: Study grant to institution
Louis-Nicolas Veilleux: PreciThera: Study grant to institution
Additional information
Funding
References
- Enderli TA, Burtch SR, Templet JN, Carriero A. Animal models of osteogenesis imperfecta: applications in clinical research. Orthop Res Rev. 2016;8:41–55. doi:10.2147/ORR.S85198.
- Takken T, Terlingen HC, Helders PJ, Pruijs H, der Ent CK V, Engelbert RH. 2004. Cardiopulmonary fitness and muscle strength in patients with osteogenesis imperfecta type I. J Pediatr. 145(6):813–818. doi:10.1016/j.jpeds.2004.08.003
- Graf A, Hassani S, Krzak J, Caudill A, Flanagan A, Bajorunaite R, et al. Gait characteristics and functional assessment of children with type I osteogenesis imperfecta. J Orthop Res. 2009;27(9):1182–1190. doi:10.1002/jor.20871.
- Veilleux LN, Lemay M, Pouliot-Laforte A, Cheung MS, Glorieux FH, Rauch F. 2014. Muscle anatomy and dynamic muscle function in osteogenesis imperfecta type I. J Clin Endocrinol Metab. 99(2):E356–62. doi:10.1210/jc.2013-3209
- Veilleux LN, Darsaklis VB, Montpetit K, Glorieux FH, Rauch F. 2017. Muscle Function in Osteogenesis Imperfecta Type IV. Calcif Tissue Int. 101(4):362–370. doi:10.1007/s00223-017-0287-y
- Moffatt P, Boraschi-Diaz I, Bardai G, Rauch F. Muscle transcriptome in mouse models of osteogenesis imperfecta. Bone. 2021;148:115940. doi:10.1016/j.bone.2021.115940.
- Tauer JT, Rauch F. Novel ActRIIB ligand trap increases muscle mass and improves bone geometry in a mouse model of severe osteogenesis imperfecta. Bone. 2019;128:115036. doi:10.1016/j.bone.2019.115036.
- Tauer JT, Rigo Canevazzi GH, Schiettekatte-Maltais J, Rauch F, Bergeron R, Veilleux LN. Muscle-bone properties after prolonged voluntary wheel running in a mouse model of dominant severe osteogenesis imperfecta. J Musculoskelet Neuronal Interact. 2021;21(4):517–527.
- Tauer JT, Abdullah S, Rauch F. 2019. Effect of anti-tgf-beta treatment in a mouse model of severe osteogenesis imperfecta. J Bone Miner Res. 34(2):207–214. doi:10.1002/jbmr.3617
- Oestreich AK, Carleton SM, Yao X, Gentry BA, Raw CE, Brown M, et al. Myostatin deficiency partially rescues the bone phenotype of osteogenesis imperfecta model mice. Osteoporos Int. 2016;27(1):161–170. doi:10.1007/s00198-015-3226-7.
- Misof K, Landis WJ, Klaushofer K, Fratzl P. 1997. Collagen from the osteogenesis imperfecta mouse model (oim) shows reduced resistance against tensile stress. J Clin Invest. 100(1):40–45. doi:10.1172/JCI119519
- McBride DJ Jr., Choe V, Shapiro JR, Brodsky B. 1997. Altered collagen structure in mouse tail tendon lacking the alpha 2(I) chain. J Mol Biol. 270(2):275–284. doi:10.1006/jmbi.1997.1106
- Sims TJ, Miles CA, Bailey AJ, Camacho NP. Properties of collagen in OIM mouse tissues. Connect Tissue Res. 2003;44(1):202–205. doi:10.1080/03008200390181663. Suppl.
- Chen F, Guo R, Itoh S, Moreno L, Rosenthal E, Zappitelli T, et al. First mouse model for combined osteogenesis imperfecta and Ehlers-Danlos syndrome. J Bone Miner Res. 2014;29(6):1412–1423. doi:10.1002/jbmr.2177.
- Miles CA, Sims TJ, Camacho NP, Bailey AJ. 2002. The role of the alpha2 chain in the stabilization of the collagen type I heterotrimer: a study of the type I homotrimer in oim mouse tissues. J Mol Biol. 321(5):797–805. doi:10.1016/s0022-2836(02)00703-9
- Chang SW, Shefelbine SJ, Buehler MJ. 2012. Structural and mechanical differences between collagen homo- and heterotrimers: relevance for the molecular origin of brittle bone disease. Biophys J. 102(3):640–648. doi:10.1016/j.bpj.2011.11.3999
- Boraschi-Diaz I, Tauer JT, El-Rifai O, Guillemette D, Lefebvre G, Rauch F, et al. Metabolic phenotype in the mouse model of osteogenesis imperfecta. J Endocrinol. 2017;234(3):279–289. doi:10.1530/JOE-17-0335.
- Tauer JT, Robinson ME, Rauch F. 2019. Osteogenesis Imperfecta: new Perspectives from Clinical and Translational Research. JBMR plus. 3(8):e10174. doi:10.1002/jbm4.10174
- Tran PHT, Skrba T, Wondimu E, Galatioto G, Svensson RB, Olesen AT, et al. The influence of fibrillin-1 and physical activity upon tendon tissue morphology and mechanical properties in mice. Physiol Rep. 2019;7(21):e14267. doi:10.14814/phy2.14267.
- Svensson RB, Smith ST, Moyer PJ, Magnusson SP. Effects of maturation and advanced glycation on tensile mechanics of collagen fibrils from rat tail and Achilles tendons. Acta Biomater. 2018;70:270–280. doi:10.1016/j.actbio.2018.02.005.
- Bailey AJ, Paul RG, Knott L. 1998. Mechanisms of maturation and ageing of collagen. Mech Ageing Dev. 106(1–2):1–56. doi:10.1016/s0047-6374(98)00119-5
- Sharma U, Carrique L, Vadon-Le Goff S, Mariano N, Georges RN, Delolme F, et al. Structural basis of homo- and heterotrimerization of collagen I. Nat Commun. 2017;8(1):14671. doi:10.1038/ncomms14671.
- Roschger A, Roschger P, Keplingter P, Klaushofer K, Abdullah S, Kneissel M, et al. Effect of sclerostin antibody treatment in a mouse model of severe osteogenesis imperfecta. Bone. 2014;66:182–188.