ABSTRACT
Cells are highly decorated with glycans (carbohydrates) and this complex and dominating coating on cells, which also carries the glycan blood group antigens, is critically important to immunology and many related clinical practices such as transfusion, transplantation and immunotherapy. The ability to modify this glycan coat is difficult to achieve with standard techniques like molecular biology or direct chemical ligation, particularly as glycosylation is probably the most complex secondary gene event in a cell. However, the natural ability of lipid-linked structures to self-insert and hydrophobically anchor into cell membranes opened up the opportunity to develop synthetic Function-Spacer-Lipid technology for cell surface modification. From this glycan modification aspect Kode Technology has been used to add glycan related blood group antigens such as ABO, Lewis, P, FORS, as well as sialo-oligosaccharides and hyaluronic acid onto cells to study immunology and cell glycosylation and more recently as a potential immuno-oncotherapeutic therapy.
Introduction
The cell membrane is much more than a simple package to retain the intracellular components within the cell; among other things it is also an interface of the cell with the environment deciding on what may enter or leave the cell, and a ‘message-board’ which allows the cell to talk and interact with its immediate environment. Traditionally images of cells show the surface of a cell being a relatively simple smooth surface decorated with a few embedded proteins, much like a sandy beach with a few shells and seaweed scattered around. In reality the surface of most cells is more like a jungle landscape covered in a range of shapes (Dane et al. Citation2015; Chevalier et al. Citation2017), some of which are close to and embedded in the surface, while others are presented on long branched tree-like structures some distance away from the surface ().
Figure 1. A, Glycocalyx ‘jungle’ of a renal endothelial cell, with a large 500 nm glycocalyx reproduced from Dane et al. Citation2015 with permission. B, In contrast the red blood cell (RBC) glycocalyx is much smaller at only 15–20 nm (for comparative purposes a 15 nm high red bar has been placed adjacent the scale of image A). C, Several schematic space filling representative Kode Technology constructs (molecules c, a, d, e and b in order from left to right as shown in ) are superimposed onto an enlarged RBC glycocalyx image, with their lipid tails inserted into the membrane and their functional moieties within the glycocalyx. Glycocalyx electron microscopy images B & C are from Voet and Voet Citation2011, Biochemistry, Figure 12-40, adapted with permission of the publisher John Wiley & Sons and remains subject to the copyright conditions of the publisher. The original image was by courtesy of Harrison Latta, UCLA.
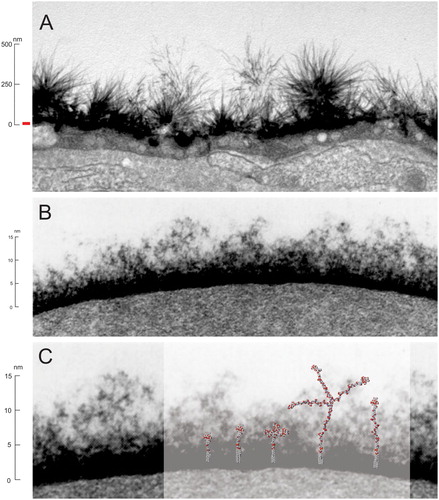
Most of these pericellular matrix components are proteoglycans, glycoproteins and glycolipids and collectively this highly glycosylated layer is termed as the glycocalyx (Mulivor and Lipowsky Citation2002; Ebong et al. Citation2011; Tarbell and Cancel Citation2016; Chevalier et al. Citation2017). Most animal epithelial cells and prokaroyte cells like bacteria have a glycocalyx and its relative size varies considerably. Some endothelial cells having a very large glycocalyx (Ebong et al. Citation2011) (A) and others such as red blood cells have a much smaller glycocalyx of around ∼10–20 nm (B) (Voet and Voet Citation2011; Chevalier et al. Citation2017). Regardless of size, the glycocalyx is a critical component to interfacing of the cell with its environment, and many important blood group antigens are present in the glycocalyx (e.g. ABO blood group). Additionally, even non-glycocalyx components at the cell surface are still influenced by glycocalyx, as anything interacting, like antibodies and ligands, must navigate their way through this coat to interact with the cell surface. The glycocalyx of a cell is involved in many different specific and non-specific biological interactions (Huang et al. Citation2014) and the ability to be able modify this external coat with specific glycans (or other moieties), and thereby influence its interactions with the environment, is useful to understanding the role of glycobiology.
Traditionally modification of the cells has been done by genetic engineering (Stephan and Irvine Citation2011). Unfortunately, molecular biology approaches are unsuited for additive glycan modification of cells with the outcomes being unpredictable and poorly controlled. This is because glycosylation is probably the most complex secondary gene event in a cell, and multiple glycosyltransferase enzymes are required to make even simple oligosaccharides, which is complicated by the fact that a single enzyme can be responsible for synthesis of different glycans (degeneracy) and different enzymes can make the same glycan (redundancy) (Oriol et al. Citation1986). Simply adding (‘knocking-in’) one enzyme is probably insufficient to create a new antigen, and such a modification can radically affect the biosynthetic pathway of many glycoconjugates, thus confound experimental observations. However, molecular biology glycan ‘knock-outs’ (resulting in deletion of a glycan family by removal of a glycosyltransferase) are useful techniques for understanding the absence of glycans, although they may still produce confounding results due to disruption of normal glycan biosynthesis. For instance, knocking-out the αGal-transferase gene, responsible for synthesis of important xeno-transplant linear B antigen (Galili Citation2015), also causes formation of new antigens as a consequence of up-regulation of other glycosyltransferase genes (Huai et al. Citation2016).
The glycolandscape of cells can certainly be modified by other methods, such as direct use of glycosidases (Olsson and Clausen Citation2007) and glycosyltransferases (Galili and Anaraki Citation1995; Stephan and Irvine Citation2011), or metabolic labelling (Dube and Bertozzi Citation2003) but the ability to controllably modify the glycolandscape by these methods is very limited, and usually incomplete and undefined.
Similarly, direct chemical modification although possible (Stephan and Irvine Citation2011; Ryzhov et al. Citation2018) is very limited as the chemistry involved is usually incompatible with cell viability, it can modify biological processes (Huang et al. Citation2017), and can alter antigenicity (Cowley et al. Citation1999).
Alternatively, the attachment of lipid linked molecules (hydrophobic insertion) into the cell membrane is possible. This natural phenomenon where glycolipids are able to spontaneously incorporate themselves into cell membranes has been known since the 1950’s (Sneath and Sneath Citation1955; Marcus and Cass Citation1969), but these natural molecules have several major limitations – in particular the onerous methods of purification from natural sources, lack of homogeneity, limited range of structures, and problematic insertion characteristics due to poor solubility. Similarly, glycosylphosphatidylinositol anchored proteins have been used to label cells (Stephan and Irvine Citation2011; Heider et al. Citation2016) with the same problems as natural glycolipids but are even more limited in utility. However, this natural phenomenon of using lipid (hydrophobic anchor) linked structures to attach small molecules to the cell membranes was the founding concept for Kode Technology.
Kode Technology constructs, also known as Function-Spacer-Lipid (FSL) constructs () were designed to modify and change the functionality of a cell surface by attaching small molecule chemical/antigenic moieties to the cell membrane with their lipid tails anchored into the lipid plasma membrane and their functional moieties presented within the glycocalyx (C). Terminology for Kode Technology was adopted to distinguish this type of modification from other types of surface modifications the techniques (Heathcote et al. Citation2010; Blake et al. Citation2011; Henry et al. Citation2018a). Similarly, the terms ‘koding’ for the process of modification, ‘kodecyte’ for any living (or dead) cell modified and still displaying FSL constructs, and ‘koded’ as a catch-all for any non-biological solution or surface modified with and displaying, FSL constructs, were adopted (Henry et al. Citation2018a). Kode Technology was originally designed only to modify cells, but the technology later expanded in use to also modify viruses (kodevirions), zebrafish, liposomes, bacteria and biological and non-biological surfaces (Blake et al. Citation2011; Hadac et al. Citation2011; Korchagina et al. Citation2012; Lan et al. Citation2012; Tesfay et al. Citation2013; Barr et al. Citation2014; Ilyushina et al. Citation2014; Korchagina and Henry Citation2015; Williams et al. Citation2016a; Henry et al. Citation2018a).
Figure 2. Examples of representative glycan Function-Spacer-Lipid constructs – A, natural blood group A glycosphingolipid, B, tetrasaccharide blood group A type 2 FSL construct with adipate (Ad) short spacer, C, tetrasaccharide blood group A type 2 FSL construct with long CMG2 spacer, D, ALeb pentasaccharide FSL with CMG2 spacer, E, FSL 6’SLN (sialo-trisaccharide), F, linear B antigen or Gal-alpha trisaccharide FSL, G, H, FSL hyaluronic acid oligomer (note the polymer repeating disaccharide D-glucuronic acid and N-acetyl-D-glucosamine can be as many units as denoted by the square brackets, with (H) showing an example FSL HA20mer with 40 saccharides.
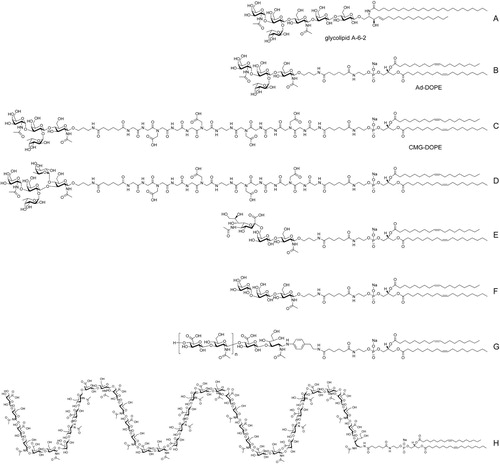
Although Kode Technology is capable of attaching almost any small molecule (Frame et al. Citation2007; Blake et al. Citation2011; Henry et al. Citation2011; Korchagina et al. Citation2012; Korchagina and Henry Citation2015) onto the outside of a cell, this review will explore in detail only the use of Kode Technology for glycan modification of cell surfaces.
Kode Technology Glycan Function-Spacer-Lipid (FSL) constructs
The Kode Technology platform is based on a single core design of construct known as a Function-Spacer-Lipid (FSL) or Kode construct. These constructs were originally designed to be synthetic glycolipids able to utilize the spontaneous cell labelling phenomenon known to associate with natural glycolipids (Sneath and Sneath Citation1955; Marcus and Cass Citation1969) (A).
However, during prototype development it became apparent that the spacer was able to confer a number of additional features to the FSL construct including a high degree of dispersibility in water, improved spontaneously insertion into cell membrane, rapid self-assembly on non-biological surfaces, and optimization of antigen presentation by spacing the functional head away from the surface (Korchagina et al. Citation2012; Korchagina and Henry Citation2015). Thus, the spacer became the core component of Kode Technology and today many variations of spacers exist, including short, long, branched, clustered and even functionalized, each of which can be further tailored to optimize bioactivity ().
Figure 3. Schematic representation of different Kode function-spacer-lipid (FSL) construct presentations of functional heads. Upper image shows a generic Kode construct based on a carboxymethylglycine spacer linked to a DOPE lipid tail. The ‘building block toy figure’ representations beneath show a yellow head representative of a single type of functional head, the red body represents a spacer, and the grey legs represent a lipid tail. The 9 structures shown at the bottom of the figure are space-filling molecular models of the building block toy figures with each having the same tetrasaccharide blood group A functional head except model f which has an (8-mer) hyaluronic acid functional head. Variation representations shown are (a) short 1 nm adipate spacer, (b) CMG 7 nm spacer, (c) sterol lipid instead of DOPE, (d) clustered head, (e) trimeric CMG spacer, (f) linear repeating functional heads, (g) double CMG spacer and (h) functionalized CMG spacer where the spacer can undertake a secondary function, in this example, the fluorophore BODIPY is attached, (i) secondarily attached functional head, which is this case uses click coupling chemistry. Figure copyright of Kode Biotech and reproduced and adapted with permission.
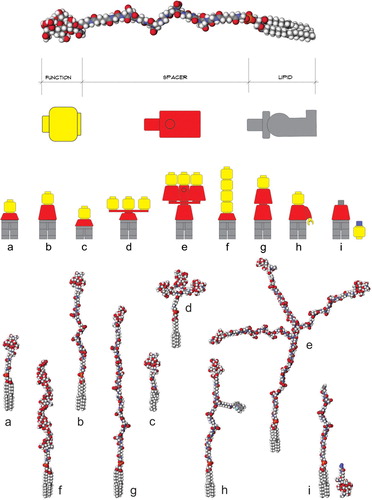
Synthesis of FSLs is carried out in a block-wise fashion (). First the individual parts (Function, Spacer and Lipid) are chemically synthesised, each with appropriate chemical conjugation handles (Korchagina et al. Citation2012). Next the spacer and lipid are conjugated together, resulting in a Spacer-Lipid precursor with one of selection of conjugation handles selected to be optimal for reactivity with the functional head group. Typically, glycans are synthesised as aminopropyl glycosides (Solís et al. Citation2015), or if the glycan is isolated from natural sources as free oligosaccharide, it is modified with a glycylamido linker (Tuzikov et al. Citation2000; Solís et al. Citation2015). Glycans are reacted with Spacer-Lipids with an activated carboxyl group (usually N-hydroxysuccinimide); maleimide is used for interacting with cysteine handles on glycopeptides (). Sometimes an alternative strategy is used where a pre-synthesised Function-Spacer is conjugated to a Lipid (in contrast to the Spacer-Lipid being conjugated to a Function); the choice of strategy is due to several factors but primarily the simplicity of isolation of the final FSL product from the reaction mixture. Because Kode Technology is inherently flexible a large range of different additional covalent chemistries can be utilised, including click chemistry (Fink and Seibel Citation2018). Furthermore, the functional head can be designed to secondarily capture other functional heads to allow post FSL modification of a cell (kodecyte) to capture of larger molecules at the cell surface. For example, primary FSL constructs containing chelators, enzyme substrates (like sortase), alkyne or azido handles (for click chemistry) and biotin (Dube and Bertozzi Citation2003; Henry et al. Citation2018a) have all been demonstrated to attach larger molecules to the surface of cells (kodecytes).
Figure 4. Two basic chemistries of conjugation: thiol-to-maleimide coupling (top), and amidation of N-oxysuccinimide-activated carboxyl group with amine (bottom); S-L is spacer-lipid.
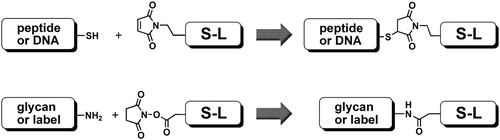
The toolbox for Function-Spacer-Lipid construction includes a variety of different lipid (L) and spacers groups (S) and this ‘lego-strategy’ facilitates the optimisation of FSL molecule for particular application () (Henry et al. Citation2018b).
Kodecytes
Once the Kode construct has inserted into the cell it becomes known as a kodecyte, which is a generic terminology to describe Kode™ Technology modified cells that detectably display Kode constructs on their surface (Henry et al. Citation2018a).
The ability of Kode constructs to attach and be retained in lipid cell membranes can be attributed to the lipid tail inserting (hydrophobic anchoring) into the cell membrane, analogous to the natural phenomenon seen with glycolipids (Sneath and Sneath Citation1955; Marcus and Cass Citation1969). Labelling of cells (‘koding’) is a very simple process and involves contacting a solution of Kode constructs with a suspension of cells and incubating for 60–120 minutes at 37°C. The amount of construct inserted into a cell is simply controlled by its concentration (Frame et al. Citation2007; Hult et al. Citation2012; Korchagina et al. Citation2012; Korchagina and Henry Citation2015; Henry et al. Citation2018a), and more than one construct can be simultaneously inserted (Frame et al. Citation2007; Henry Citation2009). The main caveat to the koding process is it should be carried out in the absence (or low concentrations) of free lipids or detergents (Oliver et al. Citation2011a, Citation2011b). Kode construct modification of cells is a temporary process and once the koding process is completed, the time the constructs will remain in the kodecyte depends on the environment that the kodecytes are exposed to, and membrane recycling activity of the kodecytes. If the kodecyte is able to recycle its membrane (such as with cell culture cells or embryos), the clearance of the Kode constructs may occur within a day. In contrast if membrane recycling is inactive as with red cells (or dead cells), and they are in a storage media, then the Kode constructs remain indefinitely in the cell membrane. However, if these red cells are exposed to lipid containing media (such as plasma or infused into the circulation of an animal) (Oliver et al. Citation2011a, Citation2011b) then the Kode constructs, just like natural glycolipids (Marcus and Cass Citation1969), will be slowly lost to the environment. Typically, in an in vivo setting red cell kodecytes will lose their Kode constructs within a few days (Oliver et al. Citation2011b).
Blood group-related glycan kodecytes
There is a large variety of glycans (carbohydrates) in the cell membrane and a small percentage of them express polymorphisms recognised as blood group systems. Many of these glycan-blood group systems are also expressed in the mucosal tissues and mucus of the airways and gastrointestinal tract (Oriol et al. Citation1986). Although the biological drivers behind these glycan polymorphisms are somewhat uncertain there is evidence to suggest that their presence, absence and masking has arisen in response to interactions with micro-organisms (Varki and Lowe Citation2017). However, assigning potential microbial interactions to a specific blood type is very complex as the expression of some blood group glycans in mucosal tissues is controlled by the secretor system (Oriol et al. Citation1986) and biosynthesis by one blood group system may use another blood group system as its precursor, thereby masking or modifying the original antigen (e.g. H, A, B, Lewis). A further layer of biological complexity is added when it is considered that the absence/presence of a glycan antigen usually allows for the presence/absence of complementary naturally occurring antibodies, which in their own right can exert a biological effect. Despite many reported associations, no one glycan blood group polymorphism appears to have an obvious advantage over another, at least in modern times.
The glycan blood group antigens present in the human red cell include those of the ABO, H, Lewis, P1PK, I, GLOB, and FORS systems (Storry et al. Citation2014). Within each of these systems there are often multiple antigenic variations, for example the ABO system has four well-recognised blood groups of A, B, AB and O. However, each individual blood type has its own variations; for example, blood group A has five core structural variations (A type 1, A type 2, A type 3, A type 4, A type 6) and additional antigenic variations formed by complex interactions with other blood group systems to form combination antigens (e.g. ALeb) (Oriol et al. Citation1986). Each of these variations is further confounded by different carriers of the antigen (glycoproteins & glycolipids, and each with multiple structural variations). As if these complexities were not enough, quantitative variations of the same antigen between individuals can create different blood phenotypic variations (e.g. A1, A2, A3, Am, Ael phenotypes). Thus, glycan blood group phenotypes are not simply defined by the presence or absence of a single qualitative (structural difference) structure, but are also a quantitative (amount present) cluster of related families of specific qualitative antigens (and their precursors), against which antibody and lectin reagents that detect them have been specifically formulated. This lack of an ‘all or nothing’ relationship adds substantial complexities to glycan profiling of cells and their blood group phenotyping – but highlights the fact that carbohydrate profiling of cells must account for both qualitative and quantitative differences while also accommodating for complexities in antibody specificity and cross-reactivity (Barr et al. Citation2014; Williams et al. Citation2016b).
Glycan complexities are not unique to human cells and all animal cells and microbes have their own monomorphic (single variation) and polymorphic (multiple variations) qualitative and quantitative glycan profiles. Fortunately, most of each of these glycan moieties can be created as FSL glycan constructs and used to create glycan specific kodecytes to analyse the biologically effect of a single glycan.
The basic process for creating glycan kodecytes is to select a cell lacking the glycan of interest and then using Kode Technology FSL construct(s) add into that cell a known glycan(s) at a known concentration(s) (Frame et al. Citation2007; Henry et al. Citation2018b). Usually the cell selected for modification is available in large quantities, thereby allowing multiple different (quantitative and qualitative) kodecytes preparations to be made from the same base cell, which can also be used in an unmodified form as the negative control. Furthermore, as the amount of antigen inserted into the kodecyte membrane can be controlled (e.g. created over a range for optimised reactivity) it is possible to make cells expressing molar equivalents of antigen, thus allowing for direct comparison of activity of a specific antigen against a specific reagent. Such unique antigen expressing qualitative and quantitative kodecytes can allow the fine specificity of blood group antibodies to be dissected and have been used to evaluate ABO (Frame et al. Citation2007; Henry Citation2009; Hult et al. Citation2012; Barr et al. Citation2014, Citation2015) and Lewis reagents (Ryzhov et al. Citation2016, Williams et al. Citation2016b). The kodecytes in those studies were made to have only a single type of antigen in their cell membrane, something which is impossible to occur naturally, and they were thus able to tease out the fine specificity of antibodies. Similarly, ABO incompatible transfusions were able to be modelled in mice because mouse red cells which do not naturally have ABO antigens could be artificially made into blood group A antigen kodecytes (Oliver et al. Citation2011a, Citation2011b).
Obtaining blood samples from weak subgroups of the ABO system, or the bacterially induced acquired B phenotype, or the FORS blood type are very problematic due to the rarity of these phenotypes. Fortunately, subgroups of A (Hult et al. Citation2012), acquired B (Frame et al. Citation2007), and the extremely rare FORS phenotype (Svensson et al. Citation2013; Jesus et al. Citation2016) can all be artificially created as kodecytes in almost unlimited quantities. Likewise, human cells bearing glycan antigens of animals (xeno-antigens) (Perry et al. Citation2016b; Middleton et al. Citation2017; Henry et al. Citation2018b) or conversely animal cells bearing specific human antigens (Oliver et al. Citation2011a, Citation2011b) can be easily made as kodecytes.
The ability to control the type and amount of antigen in a cell has quality control and diagnostic applications (Henry Citation2009; Hult et al. Citation2012). In 2004 an ABO blood group quality control product Securacell® based on Kode Technology modification red cells was introduced, and is still in the market today as the only analytic quality reagent for the ABO blood group system. Intriguingly the first-generation of this product was based on the minimum trisaccharide epitope and it occasionally had performance issues with some reagents. Subsequently it was learned that some monoclonal reagents prefer tetrasaccharide epitopes, and the product was changed accordingly (Barr et al. Citation2014). More recently we have been investigating the potential to create standardised ABO red cells for accurate quantitation of antibodies levels, for use in determining clinical levels of antibodies for ABO incompatible transplantation (Perry and Henry Citation2016a, Henry et al. Citation2018b).
Non-blood group-related glycan kodecytes.
Despite the importance of the blood group related glycans to immunology and transfusion /transplantation medicine, there is no known specific biological role for blood group glycans. However, there are many other glycans present at the cell surface, most with only a generic protective function, but some with specific functions (Varki and Lowe Citation2017). Kode constructs can be made for many of these functional glycans including sialic acid variations (Korchagina et al. Citation2012), hyaluronic acid polymers (Korchagina et al. Citation2012) and other cell differentiation markers like Lex (Williams et al. Citation2016b). The ability of these FSL constructs to alter the biological interaction of a cell has been demonstrated by changing the ability of a cell to be infected by different types of influenza virus by simply changing the sialic acid receptor on a cell surface (Korchagina et al. Citation2012). Additionally, the infectivity of a cell by the HIV virus was altered by in vitro inserting decoy FSL globotria (Gb3/Pk/CD77) glycan into peripheral blood mononuclear cells and Jurkat T-cells (Harrison et al. Citation2010; Harrison et al. Citation2011). Similarly, red blood cells were altered to interact with pathogenic bacteria by addition of the FORS antigen (Svensson et al. Citation2013).
Complement activation by glycan kodecytes
Complement is a core component of the immune system and can result in the rapid destruction of cells when activated (Klein and Anstee Citation2013). One pathway (the classical pathway) for the activation of the complement cascade is through the binding of antibodies, with antibodies directed against glycan antigens being particularly good at activating the complement cascade (Klein and Anstee Citation2013). Kodecytes bearing glycan antigens (Georgakopoulos et al. Citation2012; Perry et al. Citation2016b; Middleton et al. Citation2017) and peptide antigens (Georgakopoulos et al. Citation2012) can be created that will activate the classical complement pathway. Complement activating kodecytes using the linear B antigen (F) have been used to study the storage characteristic of complement in serum (finding it to be at least twice as stable as previously accepted) (Perry et al. Citation2016b) and as the basis of a method for determining IVIG antibody functionality (Georgakopoulos et al. Citation2012).
The complement activating ability of kodecytes is a major feature of the potential immuno-oncotherapeutic product AGI-134 based on Kode Technology (Middleton et al. Citation2017; Kristian et al. Citation2016). The concept for this immunotherapy was developed by Uri Galili where he used glycolipids isolated from rabbit red cells as the source of glycan linear B xeno antigens for inserting into cell membranes (Abdel-Motal et al. Citation2009; Galili Citation2013). Later, Kode Technology glycan construct (AGI-134, F) substituted the natural glycolipids and pre-clinical trials of this material on cell culture lines (thereby creating kodecytes) established their ability to bind human alpha-Gal antibodies and active complement (Middleton et al. Citation2017). In August 2018 the Kode Technology licensee BioLineRx (Israel) started Phase 1/2a first-in-man solid tumour human clinical trial (www.biolinerx.com) with the Kode Technology product AGI-134.
The theoretical basis for this immune oncotherapeutic product (BiolineRx Citation2018) is ‘AGI-134 is a synthetic alpha-Gal glycolipid in development for solid tumors that is highly differentiated from other cancer immunotherapies. AGI-134 is designed to label cancer cells with alpha-Gal via intratumoral administration, thereby targeting the body’s pre-existing, highly abundant anti-alpha-Gal (anti-Gal) antibodies and redirecting them to treated tumors. Binding of anti-Gal antibodies to the treated tumors results in activation of the complement cascade, which destroys the tumour cells and creates a pro-inflammatory tumor microenvironment that also induces a systemic, specific anti-tumor (vaccine) response to the patient’s own tumor neo-antigens’.
Prospective glycan koding technologies
After the first Kode construct was synthesized in 2001 it was soon appreciated that the technology had the potential to attach almost any bioactive compound to a cell membrane, and create so-called kodecytes. Today many hundreds of different kodecytes have been made, and several of them have found practical diagnostic and research product applications (Blake et al. Citation2011; Henry et al. Citation2018b), or are in clinical trials as a potential therapeutic (Middleton et al. Citation2017). New glycan (and non-glycan) FSLs are under intensive investigation for novel applications including wound healing, cell therapy, liposomal and drug targeting and as anti-bacterial coatings. The absence of any visible effect on the normal functionality of modified cells, (other than that conferred on the kodecyte by the functional group on the FSL construct) makes Kode Technology a useful and perhaps unique tool for therapeutic and diagnostic applications.
With a large repertoire of potential bioactive glycans able to be made into FSL constructs, the potential of explore many more aspects of glycobiology using Kode Technology modification of cells remains a promising avenue for further understanding cellular glycobiology.
Acknowledgments
Authors thank Dr Alexander Tuzikov and Eleanor Williams for their help in manuscript preparation.
Disclosure statement
Both authors are employees and stockholders of Kode Biotech Limited, the intellectual property owner of Kode™ Technology.
Additional information
Funding
References
- Abdel-Motal UM, Wigglesworth K, Galili U. 2009. Intratumoural injection of α-gal glycolipids induces a protective anti-tumour T cell response which overcomes Treg activity. Cancer Immunology, Immunotherapy. 58(10):1545–1556. doi: 10.1007/s00262-009-0662-2
- Barr K, Korchagina E, Popova I, Bovin N, Henry S. 2015. Monoclonal anti-A activity against the FORS1 (Forssman) antigen. Transfusion. 55:129–136. doi: 10.1111/trf.12773
- Barr K, Korchagina E, Ryzhov I, Bovin N, Henry S. 2014. Mapping the fine specificity of ABO monoclonal reagents with A and B type-specific function-spacer-lipid constructs in kodecytes and inkjet printed on paper. Transfusion. 54:2477–2484. doi: 10.1111/trf.12661
- BiolineRx. 2018. Press Release August 1, 2018. BioLineRx Initiates Phase 1/2a Clinical Study for AGI-134, a Novel Immunotherapy for Treatment of Solid Tumors www.biolinerx.com/default.asp?pageid=16&itemid=617.
- Blake DA, Bovin NV, Bess D, Henry SM. 2011. FSL Constructs: A simple method for modifying cell/virion surfaces with a range of biological markers without affecting their viability. Journal of Visualized Experiments. Aug 5(54):e3289. (http://www.jove.com/details.php?id=3289).
- Chevalier L, Selim J, Genty D, Baste JM, Piton N, Boukhalfa I, Hamzaoui M, Pareige P, Richard V. 2017. Electron microscopy approach for the visualization of the epithelial and endothelial glycocalyx. Morphologie. 101:55–63. doi: 10.1016/j.morpho.2017.04.001
- Cowley H, Wojda U, Cipolone KM, Procter JL, Stroncek DF, Miller JL. 1999. Biotinylation modifies red cell antigens. Transfusion. 39(2):163–168. doi: 10.1046/j.1537-2995.1999.39299154730.x
- Dane MJC, van den Berg BM, Lee DH, Boels MGS, Tiemeier GL, Avramut MC, van Zonneveld AJ, van der Vlag J, Vink H, Rabelink TJ. 2015. A microscopic view on the renal endothelial glycocalyx. American Journal of Physiology-Renal Physiology. 308:F956–F966. doi: 10.1152/ajprenal.00532.2014
- Dube DH, Bertozzi CR. 2003. Metabolic oligosaccharide engineering as a tool for glycobiology. Current Opinion in Chemical Biology. 7:616–625. doi: 10.1016/j.cbpa.2003.08.006
- Ebong EE, Macaluso FP, Spray DC, Tarbell JM. 2011. Imaging the Endothelial Glycocalyx In Vitro by Rapid Freezing/Freeze Substitution Transmission Electron Microscopy. Arteriosclerosis. Thrombosis and Vascular Biology. 31:1908–1915. doi: 10.1161/ATVBAHA.111.225268
- Fink J, Seibel J. 2018. Click reactions with functional sphingolipids. Biological Chemistry. Jun 19. pii: /j/bchm.ahead-of-print/hsz-2018-0169/hsz-2018-0169.xml. doi:10.1515/hsz-2018-0169.
- Frame T, Carroll T, Korchagina E, Bovin N, Henry S. 2007. Synthetic glycolipid modification of red blood cell membranes. Transfusion. 47:876–882. doi: 10.1111/j.1537-2995.2007.01204.x
- Galili U. 2013. In situ conversion of tumours into autologous tumour-associated antigen vaccines by intratumoral injection of α-gal glycolipids. OncoImmunology. 2(1). Article: e22449. doi: 10.4161/onci.22449
- Galili U. 2015. Significance of the evolutionary α1,3-galactosyltransferase (GGTA1) gene inactivation in preventing extinction of apes and old world monkeys. Journal of Molecular Evolution. 80(1):1–9. doi: 10.1007/s00239-014-9652-x
- Galili U, Anaraki F. 1995. α-galactosyl (Galα1-3Galβ1-4GlcNAc-R) epitopes on human cells: synthesis of the epitope on human red cells by recombinant primate α1,3galactosyltransferase expressed in E.coli. Glycobiology. 5(8):775–782. doi: 10.1093/glycob/5.8.775
- Georgakopoulos T, Komarraju S, Henry S, Bertolini J. 2012. An improved Fc function assay utilising CMV antigen coated red blood cells generated with synthetic Function-Spacer-Lipid constructs. Vox Sanguinis. 102:72–78. doi: 10.1111/j.1423-0410.2011.01512.x
- Hadac EM, Federspiel MJ, Chernyy E, Tuzikov A, Korchagina E, Bovin NV, Russell S, Henry SM. 2011. Fluorescein and radiolabeled Function-Spacer-Lipid constructs allow for simple in vitro and in vivo bioimaging of enveloped virions. Journal of Virological Methods. 176:78–84. doi: 10.1016/j.jviromet.2011.06.005
- Harrison AL, Henry S, Mahfoud R, Manis A, Albertini A, Gaudin Y, Lingwood CA, Branch DR. 2011. A novel VSV/HIV pseudotype approach for the study of HIV microbicides without requirement for level 3 biocontainment. Future Virology. 6(10):1241–1259. doi: 10.2217/fvl.11.88
- Harrison AL, Olsson ML, Jones BR, Ramkumar S, Sakac D, Binnington B, Henry S, Lingwood CA, Branch DR. 2010. A synthetic globotriaosylceramide analogue inhibits HIV-1 infection in vitro by two mechanisms. Glycobiology. 27:515–524.
- Heathcote D, Carroll T, Wang JJ, Flower R, Rodionov I, Tuzikov A, Bovin N, Henry S. 2010. Novel antibody screening cells, MUT + Mur kodecytes, created by attaching peptides onto erythrocytes. Transfusion. 50:635–641. doi: 10.1111/j.1537-2995.2009.02480.x
- Heider S, Dangerfield JA, Metzner C. 2016. Biomedical applications of glycosylphosphatidylinositol-anchored proteins. Journal of Lipid Research. 57(10):1778–1788. doi: 10.1194/jlr.R070201
- Henry S. 2009. Modification of red blood cells for laboratory quality control use. Current Opinion in Hematology. 16(6):467–472. doi: 10.1097/MOH.0b013e328331257e
- Henry S, Perry H, Bovin N. 2018b. Applications for kodecytes in immunohematology. ISBT Science Series. 13:229–237. doi: 10.1111/voxs.12403
- Henry S, Williams E, Barr K, Korchagina E, Tuzikov A, Ilyushina N, Abayzeed SA, Webb KF, Bovin N. 2018a. Rapid one-step biotinylation of biological or non-biological surfaces. Scientific Reports. 8:2845. doi: 10.1038/s41598-018-21186-3
- Henry SM, Komarraju S, Heathcote D, Rodinov I. 2011. Designing peptide-based FSL constructs to create Miltenberger kodecytes. ISBT Science Series. 6:306–312. doi: 10.1111/j.1751-2824.2011.01505.x
- Huai G, Qi P, Yang H, Wang Y. 2016. Characteristics of α-Gal epitope, anti-Gal antibody, α1,3 galactosyltransferase and its clinical exploitation. International Journal of Molecular Medicine. 37:11–20. doi: 10.3892/ijmm.2015.2397
- Huang ML, Smith RAA, Trieger GW, Godula K. 2014. Glycocalyx remodeling with proteoglycan mimetics promotes neural specification in embryonic stem cells. Journal of American Chemical Society. 136:10565–10568. doi: 10.1021/ja505012a
- Huang X, Leroux J-C, Castagner B. 2017. Well-defined multivalent ligands for hepatocytes targeting via asialoglycoprotein receptor. Bioconjugate Chemistry. 28:283–295. doi: 10.1021/acs.bioconjchem.6b00651
- Hult AK, Frame T, Chesla S, Henry S, Olsson ML. 2012. Flow cytometry evaluation of red blood cells mimicking naturally-occurring ABO subgroups following modification with variable amounts of FSL-A and B constructs. Transfusion. 52:247–251. doi: 10.1111/j.1537-2995.2011.03268.x
- Ilyushina NA, Chernyy ES, Korchagina EY, Gambaryan AS, Henry SM, Bovin NV. 2014. Labeling of influenza viruses with synthetic fluorescent and biotin-labeled lipids. Virologica Sinica. 29(4):199–210. doi: 10.1007/s12250-014-3475-1
- Jesus C, Hesse C, Rocha C, Osório N, Valado A, Caseiro A, Gabriel A, Svensson L, Moslemi A-R, Siba WA, et al. 2016. Prevalence of antibodies to a new histo-blood system: the FORS system. Blood Transfusion. epub. doi 10.2450/2016.0120-16
- Klein HG, Anstee DJ. 2013. Mollison’s blood transfusion in clinical medicine. Somerset: Wiley.
- Korchagina E, Tuzikov A, Formanovsky A, Popova I, Henry S, Bovin N. 2012. Toward creating cell membrane glycolandscapes with glycan lipid constructs. Carbohydrate Research. 356:238–246. doi: 10.1016/j.carres.2012.03.044
- Korchagina Y, Henry SM. 2015. Synthetic glycolipidlike constructs as tools for glycobiology research, diagnostics, and as potential therapeutics. Biochemistry (Moscow). 80:857–871. doi: 10.1134/S0006297915070068
- Kristian SA, Shaw SM, Wigglesworth K, Middleton J, Glossop M, Whalen GF, Old R, Galili U, Westby M, Pickford C. 2016. AGI-134, a fully synthetic α-Gal-based cancer immunotherapy: synergy with an anti-PD-1 antibody and pre-clinical pharmacokinetic and toxicity profiles. Journal of Clinical Oncology. 34(suppl):3083. doi: 10.1200/JCO.2016.34.15_suppl.3083
- Lan C-C, Blake D, Henry S, Love DR. 2012. Fluorescent Function-Spacer-Lipid construct labelling allows for real-time in vivo imaging of cell migration and behaviour in zebrafish (Danio rerio.). Journal of Fluorescence. 22:1055–1063. doi: 10.1007/s10895-012-1043-3
- Marcus DM, Cass LE. 1969. Glycosphingolipids with Lewis blood group activity: Uptake by human erythrocytes. Science. 164:553–555. doi: 10.1126/science.164.3879.553
- Middleton JL, Schulz O, Charlemagne A, Kristian SA, Shaw S. 2017. The novel α-Gal-based immunotherapy AGI-134 invokes CD8+ T cell-mediated immunity by driving tumour cell destruction, phagocytosis and tumour-associated antigen cross-presentation via multiple antibody-mediated effector functions (abstract nr 616) AACR. Cancer Research. 77(13 Suppl). doi:10.1158/1538-7445.AM2017-616.
- Mulivor W, Lipowsky HH. 2002. Role of glycocalyx in leukocyte-endothelial cell adhesion. American Journal of Physiology-Heart and Circulatory Physiology. 283:H1282–H1291. doi: 10.1152/ajpheart.00117.2002
- Oliver C, Blake D, Henry S. 2011a. In vivo neutralization of anti-A and successful transfusion of a antigen incompatible red cells in an animal model. Transfusion. 51:2664–2675. doi: 10.1111/j.1537-2995.2011.03184.x
- Oliver C, Blake D, Henry S. 2011b. Modeling transfusion reactions and predicting in vivo cell survival with kodecytes. Transfusion. 51:1723–1730. doi: 10.1111/j.1537-2995.2010.03034.x
- Olsson ML, Clausen H. 2007. Modifying the red cell surface: towards an ABO-universal blood supply. British Journal of Haematology. 140:3–12.
- Oriol R, Le Pendu J, Mollicone R. 1986. Genetics of ABO, H, Lewis, X and related antigens. Vox Sanguinis. 51(3):161–171. doi: 10.1111/j.1423-0410.1986.tb01946.x
- Perry H, Bovin N, Henry S. 2016b. Antibody complement-mediated hemolytic studies with kodecytes reveal human complement utilized in the classical pathway is more stable than generally accepted. Transfusion. 56:2495–2501. doi: 10.1111/trf.13719
- Perry H, Henry SM. 2016a. Standardized blood group A kodecytes allow for determination of anti-A,B titres without the need for sample dilution (SP248). AABB Florida, October 2016, Transfusion. S4, 3A-262.
- Ryzhov IM, Korchagina EY, Tuzikov AB, Popova IS, Tyrtysh TV, Pazynina GV, Henry SM, Bovin NV. 2016. Function-Spacer-Lipid constructs of Lewis and chimeric Lewis/ABH glycans. Synthesis and use in serological studies. Carbohydrate Research. 435:83–96. doi: 10.1016/j.carres.2016.09.016
- Ryzhov IM, Tuzikov AB, Perry H, Korchagina EY, Bovin NV. 2018. Blood Group O → A transformation by chemical ligation of erythrocytes. ChemBioChem. in press. doi: 10.1002/cbic.201800289
- Sneath JS, Sneath PHA. 1955. Transformation of Lewis groups of human red cells. Nature. 176:172. doi: 10.1038/176172a0
- Solís D, Bovin NV, Davis AP, Jiménez-Barbero J, Romero A, Roy R, Smetana Jr K, Gabius H-J. 2015. A guide into glycosciences: how chemistry, biochemistry and biology cooperate to crack the sugar code. Biochimica et Biophysica Acta (General subjects). 1850:186–235. doi: 10.1016/j.bbagen.2014.03.016
- Stephan MT, Irvine DJ. 2011. Enhancing cell therapies from the outside in: cell surface engineering using synthetic nanomaterials. Nano Today. 6(3):309–325. doi: 10.1016/j.nantod.2011.04.001
- Storry JR, Castilho L, Daniels G, Flegel WA, Garratty G, de Haas M, Hyland C, Lomas-Francis C, Moulds JM, Nogues N, et al. 2014. International Society of Blood Transfusion Working Party on red cell immunogenetics and blood group terminology: Cancun report (2012). Vox Sanguinis. 107:90–96. doi: 10.1111/vox.12127
- Svensson L, Hult AK, Stamps R, Angstršm J, Teneberg S, Jorgensen R, Rydberg L, Henry SM, Olsson ML. 2013. Forssman expression on human erythrocytes: Biochemical and genetic evidence of a new histo-blood group system. Blood. 121:1459–1468. doi: 10.1182/blood-2012-10-455055
- Tarbell JM, Cancel LM. 2016. The glycocalyx and its significance in human medicine. Journal of Internal Medicine. 280(1):97–113. doi: 10.1111/joim.12465
- Tesfay MZ, Krik A, Hadac EM, Griesmann GE, Federspiel MJ, Barber GN, Henry SM, Peng K-W, Russell SJ. 2013. PEGylation of vesicular stomatitis virus extends virus persistence in blood circulation of passively immunized mice. Journal of Virology. 87:3752–3759. doi: 10.1128/JVI.02832-12
- Tuzikov AB, Gambaryan AS, Juneja LR, Bovin NV. 2000. Conversion of complex oligosaccharides into polymeric conjugates and their anti-influenza virus inhibitory potency. Journal of Carbohydrate Chemistry. 19:1191–1200. doi: 10.1080/07328300008544143
- Varki A, Lowe JB. 2017. Biological roles of Glycans. Glycobiology. 27:3–49.
- Voet D, Voet JG. 2011. Biochemistry. 4th ed. Chapter 12 Lipids and Membranes. Hoboken, N.J.: John Wiley & Sons.
- Williams E, Barr K, Korchagina E, Tuzikov A, Henry S, Bovin N. 2016a. Ultra-fast glyco-coating of non-biological surfaces. International Journal of Molecular Sciences. 17(1):118. doi: 10.3390/ijms17010118
- Williams E, Korchagina E, Frame T, Ryzhov I, Bovin N, Henry S. 2016b. Glycomapping the fine specificity of monoclonal and polyclonal Lewis antibodies with type-specific kodecytes and FSL constructs printed on paper. Transfusion. 56:325–333. doi: 10.1111/trf.13384