Abstract
The present study examined the possible role of reactive oxygen species in the pathogenesis of heart failure in broilers. Data were collected from three groups of birds at various risk of heart failure: Leghorn chickens (resistant to heart failure), slow-growing feed-restricted broilers (low risk of heart failure), fast-growing ad libitum fed broilers (high risk of heart failure), and broilers with congestive heart failure (CHF). In the first part of the study, basic clinical parameters and ultrastructural changes were examined in the context of lipid peroxidation of the ventricular myocardium. This was followed by the study of in vitro changes in the activity of selected cytosolic enzymes (creatine kinase and lactate dehydrogenase) and mitochondrial enzymes (pyruvate dehydrogenase and α-ketoglutarate dehydrogenase) in the presence of oxidants (hydrogen peroxide or tertiary butyl hydroperoxide). The distinctive clinical feature in the fast-growing broilers and in the broilers with CHF as compared with slow-growing broilers or Leghorn chickens was a significantly lower heart rate (P <0.05). Electron microscopy revealed marked morphological changes in myocardial mitochondria in these broilers (i.e. fast-growing broilers and broilers with CHF). The level of malondialdehyde equivalents, an indicator of lipid peroxidation subsequent to generated oxidative stress, was significantly higher (P <0.05) in ad libitum fed broilers and was highest (P <0.01) in broilers with CHF. In vitro, the presence of oxidants had a detrimental effect on creatine kinase and α-ketoglutarate dehydrogenase activity, while lactate dehydrogenase activity increased. The activity of pyruvate dehydrogenase was not altered by oxidants. Our results indicate that the deterioration of heart function in fast-growing commercial broilers in our experimental model is associated with oxidative stress leading to lipid peroxidation of cellular and mitochondrial membranes, and decreased activity of myocardial creatine kinase and α-ketoglutarate dehydrogenase enzymes critical for energy synthesis and transformation pathways.
Introduction
Modern fast-growing broilers are inherently more susceptible to heart diseases than laying strains. Previous studies from our laboratory revealed that a relatively large number of commercial broilers show evidence of hypoxemia associated with subclinical heart disease (Olkowski et al., Citation2005; Nain et al., 2008).
Oxidative metabolism is a normal process in all tissues. Cardiomyocytes require a constant supply of oxygen for normal cardiac functions. However, oxygen-associated metabolism in the myocardium sometimes can contribute to cardiac dysfunction, and may ultimately lead to heart failure (Giordano, Citation2005; Redout et al., Citation2007). During the normal oxidative metabolic process, various reactive oxygen species (ROS) and reactive nitrogen species (RNS) are produced. During this normal metabolism, 1% to 2% of oxygen is converted to ROS (Sheeran & Pepe, 2006). Several specialized metabolic mechanisms normally dispose off these very harmful reactive species. However, under some circumstances, increased ROS/RNS production or decreased antioxidant defenses may lead to oxidative stress where the generated reactive species can alter the properties of lipids, proteins, and nucleic acids, leading to cellular dysfunctions (for a review see Orrenius et al., Citation2007). Recent research findings from different laboratories suggest that ROS and RNS play a critical role in development of human heart failure (Andreka et al., Citation2004; Sam et al., Citation2005; Nediani et al., Citation2007).
Lipid peroxidation can alter the membrane properties of cellular and subcellular organelles (mitochondria and sarco-endoplasmic reticulum) crucial for maintenance of normal cardiomyocyte function. Broilers with congestive heart failure (CHF) show evidence of calcium overload in these subcellular components (Maxwell et al., Citation1993; Li et al., Citation2006), and evidence of breakdown and release of the protein of contractile apparatus, such as myosin and troponin T, into the circulation (Maxwell et al., Citation1994).
The role of oxidative stress has long been debated in the pathogenesis of heart failure in human and animal models of cardiomyopathy. However, limited research has been carried out to investigate the possible involvement of oxidative stress in CHF in broilers. Recent studies from our laboratory revealed that energy metabolism is compromised in broilers developing heart failure (Nain et al., 2008; Olkowski et al., Citation2007). In order to further understand the physiological and biochemical disturbances leading to CHF in fast-growing commercial broilers, we were interested to examine the possible role and molecular mechanisms of oxidative stress in the pathogenesis of chronic heart failure. In order to explain the molecular mechanisms underlying the pathogenesis of heart failure in commercial broilers we compared clinical, morphological, molecular and biochemical parameters among chickens that were categorized according to risk of developing heart failure as follows: (1) Leghorn chickens (resistant to heart failure); (2) feed-restricted, slow-growing broilers (low risk of heart failure); (3) ad libitum fed, fast-growing broilers (high risk and incidence of heart failure) and (4) broilers showing signs of congestive heart failure (broilers with CHF, observed within the ad libitum fed group). Firstly, oxidative stress associated with the deterioration of heart function was measured. Secondly, we examined the impact of simulated oxidative stress on the activities of essential enzymes involved in cardiac energy synthesis and transformation pathways.
Materials and Methods
Animal and management
The birds (all commercial broiler males; Aviagen) used to measure specific parameters represented a random sample obtained from basic flocks consisting of 48 Leghorns, 39 feed-restricted slow-growing broilers, and 83 fast-growing ad libitum fed broilers. The group of broilers designated as slow-growing was subjected to a feed restriction regime (70% ad libitum) from day 7 onwards. All other groups of birds were fed ad libitum. The birds were raised using the lowered environmental temperature protocol as described previously (Olkowski et al., Citation1999). Briefly, during the first 7 days the temperature was maintained at 34°C followed by a gradual decrease to a level approximately 21°C by the end of the third week, and 17°C by the end of the fifth week. The lowered environmental temperature forces the birds to increase their metabolic rate, and this results in increased burden on the cardiovascular system, which effectively exposes metabolic weaknesses of cardiac tissue.
The experimental protocols were approved by the University of Saskatchewan Animal Care Committee. The procedures were performed as per the requirements of the Guide to the Care and Use of Experimental Animals (Canadian Council on Animal Care, Citation1993).
Clinical evaluation
Birds were monitored daily for overt signs of heart disease such as fatigue, exercise intolerance, tachypnea, hypoxemia, generalized cyanosis, and ascites. Birds in extremis were euthanized. Detailed physical examination was performed prior to data collection. Heart rate measurements were obtained using electrocardiography as described previously (Olkowski & Classen, Citation1998) in 10 birds from each group at the end of the fifth week of the experiment. Birds showing the above listed signs were classified as broilers with CHF.
Transmission electron microscopy
To determine the ultrastructural changes in myocardium of birds at various risk of heart failure, hearts obtained from Leghorn chickens, fast-growing ad libitum fed broilers, and broilers with CHF were processed for transmission electron microscopy. Heart tissue samples from the mid portion of the left ventricular free wall were processed as described previously (Olkowski et al., Citation2001).
Heart tissue procurement for biochemical analysis
Heart tissue samples used for biochemical analysis were obtained from five randomly selected birds from each group as described above, at the end of the sixth week of the experiment. Hearts were collected following euthanasia by cervical dislocation, and were snap frozen in liquid nitrogen. These were stored in liquid nitrogen until analyzed.
Thiobarbituric acid reacting substances assay
Thiobarbituric acid reacting substances (TBARS), an indicator of oxidative damage, were measured spectrophotometrically as described previously by Ohkawa et al. (Citation1979) with modifications. Briefly, frozen/pulverized tissue samples from the mid portion of the left ventricular myocardium were homogenized first in KCl mixture (2 mM ethylene glycol tetraacetic acid, 0.02% butylated hydroxytoluene (BHT) in 1.15% KCl) using microtube homogenizer and then radioimmune precipitation assay (RIPA) buffer (50 mM Tris–HCl, 150 mM NaCl, 1 mM ethylenediamine tetraacetic acid, 1% Triton X-100, 1% sodium deoxycholate and 1% sodium dodecyl sulfate, with pH adjusted to 7.2 using NAOH) was added, and the assay was thoroughly mixed. Finally, 1 g heart tissue was present in 6 ml solution containing RIPA buffer and KCl mixture in a ratio of 4:5 v/v. The homogenate was centrifuged at 15 000×g for 10 min at 4°C. Then 200 µl supernatant was mixed with 0.2 ml of 8.1% sodium dodecyl sulfate, 2500 µl of 30% acetic acid (pH adjusted to 3.5), 375 µl of 0.8% thiobarbituric acid (TBA) and 8.25 µl of 0.02% BHT aqueous solution followed by incubation at 95°C for 1 h. After incubation and subsequent cooling, an equal volume of n-butanol/pyridine mixture (15:1 v/v ratio) was added. After vigorous shaking, the assay was centrifuged at 4000×g for 10 min. The organic layer obtained at the top following centrifugation was used for measuring thiobarbituric reactive substances at 532 nm. A calibration curve was prepared using a malondialdehyde (MDA) standard, and the dilution was made in such a way that the expected concentration of each sample falls in the middle range of the calibration curve. The coefficient of determination approached 0.99 for the standard curve. All samples were analyzed in duplicate in the same assay to avoid interassay variability.
In vitro enzyme inhibition
The activity of selected cytosolic (creatine kinase [CK] and lactate dehydrogenase [LDH]) and mitochondrial (pyruvate dehydrogenase [PDH] and α-ketoglutarate dehydrogenase [α-KGDH]) enzymes was tested using an in vitro indicator of oxidative stress (i.e. hydrogen peroxide [H2O2] or tertiary butyl hydroperoxide [TBH] or both). Enzyme sensitivity measurements were performed in heart tissue obtained from the mid portion of the left ventricle free wall of fast-growing ad libitum fed broilers.
The activities of CK, LDH, PDH and α-KGDH were measured as described previously (Nain et al., Citation2008). Briefly, aliquots of 300 mg frozen samples were homogenized in phosphate buffer (50 mM, pH 7.4) in the ratio of 1:10 (100 mg tissue:1 ml buffer) in test tubes pre-cooled with ice. The homogenate was centrifuged at 2500×g for 10 min at 4°C and the suspension was further centrifuged at 12 000×g for 10 min. The supernatant was used for CK and LDH measurements. The CK activity was measured using a CK kit (Roche Diagnostics, Indianapolis, Indiana, USA) while LDH activity was measured using LD-L10 (Sigma Diagnostics Inc., St Louis, Missouri, USA). The mitochondria-containing pellet was further washed three times with phosphate buffer to remove remnants of cytosolic enzymes, and finally this pellet was resuspended in Tris buffer (50 mM, pH 7.6 with 0.5% Triton). The PDH and α-KGDH activities were assayed in mitochondrial extracts. The PDH activity was measured using a cocktail, where the final components of the incubation media were 2 mM pyruvate, 2.5 mM nicotinamide adenine dinucleotide (NAD), 0.15 mM flavin adenine dinucleotide, 2 mM MgCl2, 0.2 mM thiamine pyrophosphate, 0.13 mM coenzyme A, 2.6 mM dithiothreitol, and 30 mM Tris buffer at pH 7.2. The α-KGDH activity was measured using a cocktail containing 3.2 mM α-keto glutaric acid, 2 mM NAD, 0.5 mM coenzyme A, 0.7 mM thiamine pyrophosphate, and 1 mM MgCl2. The assays were validated for the linearity of responses for time of reaction and protein content.
Initially, the inhibition study of all the above-mentioned enzymes was performed in presence of various concentrations of H2O2, but because the activities of PDH and α-KGDH were not affected by H2O2 we also assessed the activity of these enzymes in the presence of serial dilutions of TBH.
Before performing final measurements, each component of the assay cocktail mixture for every enzyme was incubated in the presence of H2O2 or TBH, to establish that the individual components of these enzymes were not affected by the presence of oxidants—the results obtained are purely subsequent to enzyme oxidant interaction.
The reaction was initiated by adding 20 µl cytosolic or mitochondrial fraction in 200 µl cocktail plus 5 µl serially diluted H2O2 or TBH per well in a 96-well micro plate pre-incubated at 37°C for all of the above-mentioned enzymes. Enzyme activity measurements were performed at 340 nm using a microplate reader SpectraMax Plus (Molecular Devices, California, USA). The final measurements were performed during the linear phase of responses pre-established during the validation phase as described previously. The inhibition constant (IC50) was used to evaluate the concentration of the oxidants required for 50% inhibition of an enzyme activity. IC50 values were calculated using GraphPad Prism 3.03 (GraphPad Software, San Diego, California, USA).
Statistical analysis
Statistical analyses were carried out by analysis of variance from the microcomputer package Number Cruncher Statistical System (Hintze, Citation1995). The means were compared using Fisher’ s least significant difference (LSD) Replace test. Statistical significance was assumed to exist when the probability of making a type I error was less than 0.05.
Results
Incidence of heart failure
Overall, during the course of this study, fulminant CHF (based on mortality and morbidity data) was observed in 38 out of 83 (46%) broilers from the ad libitum fed group. None of the birds from the feed-restricted and Leghorn groups showed clinical signs indicative of heart disease.
Heart rate
The cardiac measurements revealed a markedly (P < 0.05) lower heart rate (308 beats/min) in the ad libitum fed group as compared with the feed-restricted (heart rate, 371 beats/min) and Leghorn chickens (heart rate, 372 beats/min). Profound bradycardia (heart rate, 236 beats/min) was observed in broilers with fulminant CHF.
Gross pathology and ultrastructural changes
Postmortem examination was performed on birds that were euthanized or died during the course of study. Broilers with CHF showed gross dilation of the ventricular chambers along with pulmonary congestion. Approximately 80% to 90% of broilers showing these changes also showed severe pericardial effusion and the accumulation of ascitic fluid in the abdominal cavity.
Ultrastructural examination revealed significant morphological changes in the cardiomyocytes’ contractile apparatus and mitochondria. In some areas, disintegration or even total dissolution of myofibrillar elements were observed. Mitochondrial lesions were seen in some apparently normal broilers, but predominantly in broilers with CHF. Major morphological changes in the mitochondria include pleomorphism, variable degrees of swelling and vacuolization along with disintegration and loss of cristae.
A distinctive feature in fast-growing ad libitum fed broilers, as compared with Leghorn chickens, was that the inter-fibrillar mitochondria were numerous and appeared in large clusters. Careful evaluation of electron micrographs () revealed that changes were most pronounced in broilers with CHF.
Figure 1. Representative transmission electron micrographs from left ventricular myocardium of (1a) a Leghorn chicken (resistant to heart failure), (1b) a fast-growing ad libitum fed broiler (high risk of heart failure) and (1c) a broiler that developed CHF. Original magnification×24 000. Comparable presentation of electron micrographs from various categories of birds, revealing various degrees of mitochondrial lesions. 1a: Micrograph from the Leghorn reveals mitochondria are monomorphic with well-defined cristae (white arrow) and mitochondrial membrane is intact. 1b: Micrograph from fast-growing ad libitum fed broiler shows various degrees of changes to mitochondrial architecture; some mitochondria are normal while few revealed vacuolization and disintegration of cristae (black arrow). 1c: Micrograph from broiler with CHF shows mitochondrial swelling, vacuolization and disintegration of cristae (black arrow).
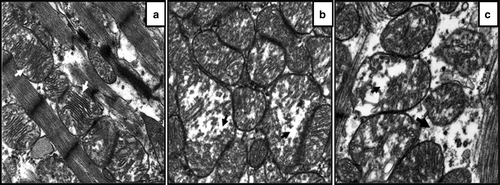
Thiobarbituric acid reacting substances assay
Findings from the TBARS assay showed that lipid peroxidation was highest (P < 0.05) in broilers with CHF as compared with slow-growing feed-restricted broilers, fast-growing ad libitum fed broilers and Leghorn chickens (). The findings from this assay further revealed that fast-growing ad libitum fed broilers had higher (P < 0.05) lipid peroxidation as compared with slow-growing feed-restricted broilers and Leghorn chickens. The values from slow-growing feed-restricted broilers and Leghorn chickens did not differ significantly (P > 0.05).
Table 1. Heart rate and MDA equivalent levels measured from Leghorn chickens, feed-restricted slow-growing broilers (Broiler-Res), fast-growing broilers fed ad libitum (Broiler-AL), and in broilers with CHF and ascites (Broiler-CHF)
Enzyme inhibition
In the first part of the study it was ensured that the enzymatic assay components (CK and LDH kits, α-KGDH and PDH cocktail components) were not affected by the presence of oxidants ().
Table 2. Effect of the presence of oxidants (H2O2 or TBH) on enzyme assay kit/cocktail components (CK and LDH kits, PDH and α-KGDH cocktails)
In vitro enzyme inhibition studies revealed a decreased CK activity (IC50=5.71 mM) in the presence of increasing H2O2 concentrations (). However, the LDH activity was increased in the presence of H2O2 at a concentration above 12.2 mM.
Figure 2. The CK, LDH, α-KGDH, and PDH enzyme sensitivity to oxidative stress tested in the presence of H2O2 or TBH. 2a: CK activity, note decreased activity (IC50=5.71 mM) as the concentration of H2O2 increases. 2b: LDH activity, note activity of LDH increases with increased concentration of H2O2. 2c: α-KGDH activity, note decreased activity (IC50=6.25 mM) as the concentration of TBH increases. 2d: PDH activity, no affect on activity in the presence of TBH. Each data point represents the average of three measurements. Vertical bars indicate the standard error of the mean. OD, optical density.
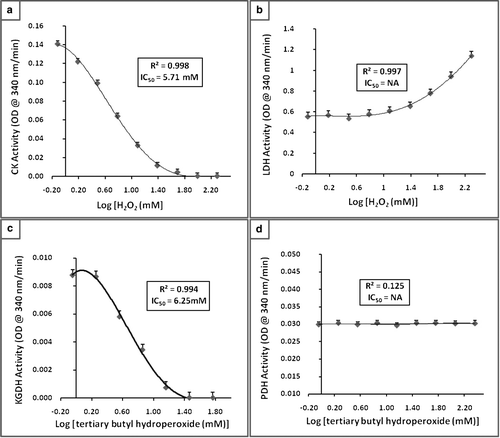
The PDH activity was not affected by the presence of H2O2 and TBH. The α-KGDH activity was not affected by the presence of H2O2 but decreased in the presence of increasing concentrations of TBH (IC50=6.25 mM).
Discussion
Several findings from the present study provide evidence that deterioration in heart function in broilers is associated with oxidative stress. The morphological changes observed in cardiac mitochondria in ad libitum fed broilers and in broilers with CHF are consistent with damage associated with oxidative stress, and this is well supported by biochemical evidence of damage to mitochondrial membranes caused by lipid peroxidation.
The Leghorn chickens in the present study did not show any sign of heart failure; hence measurements from the Leghorns were used as reference values. In order to understand the trends in the measured parameters (MDA equivalents and heart rate), the values from the slow-growing feed-restricted broilers (low risk of heart failure), fast-growing ad libitum fed broilers (high risk of heart failure) and broilers with CHF (observed within the ad libitum fed group) were plotted with respect to levels observed in Leghorn chickens (resistant to heart failure) taken as 100% ().
Figure 3. Relative heart rate and level of MDA equivalents in feed-restricted slow-growing broilers (Broiler-Res, low risk of heart failure), ad libitum fed fast-growing broilers (Broiler-AL, high risk of heart failure) and broilers with CHF (Broiler-CHF), expressed on a percentage basis of Leghorn chickens (Leghorn, resistant to heart failure).
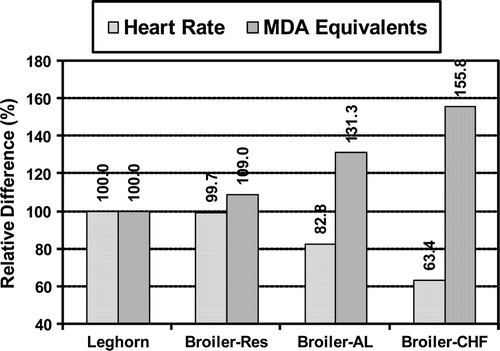
The present findings further confirmed that fast-growing commercial broilers are at increased risk of heart failure. This increased risk of heart failure in broilers was associated with deterioration of heart functions as revealed by lowered heart rate observed in ad libitum fed broilers, and in broilers with CHF as compared with feed-restricted slow-growing broilers and Leghorn chickens.
A declining heart rate is the most characteristic early pathophysiological feature of deterioration of heart pump function in fast-growing broilers. This has been reported in previous studies from our laboratory (Olkowski & Classen, Citation1998; Olkowski et al., Citation2005, Citation2007, Nain et al., 2008), as well as studies of others (Deng et al., Citation2006; Druyan et al., Citation2007), and has been confirmed in the present study. Interestingly, as demonstrated in , the pattern of lowered heart rate appears to be strongly correlated with increasing levels of TBARS. This indicates that the deterioration of heart function is associated with oxidative damage in the myocardium.
Morphological changes observed in myocardial mitochondria in the present study are consistent with oxidative damage. Notably, mitochondria are the major source of ROS, but, because of their very high component of membranes, they are also a very sensitive target of ROS attack. The membrane lipids are very sensitive to oxidative damage due to the presence of polyunsaturated fatty acids, subsequently leading to lipid peroxidation (Halliwell & Gutteridge, Citation1985). Currently, one of the most common and well recognized approaches to measure the effects of free radicals is by measuring the oxidative damage (i.e. lipid peroxidation) to cellular membranes (Lykkesfeldt & Svendsen, Citation2007).
The measurements from the lipid peroxidation in the present study showed that, in fast-growing broilers, oxidative stress increases as the risk of heart failure increases (). Moreover, the observed oxidative damage can readily be associated with morphological changes in the mitochondria.
The biochemical evidence of oxidative damage (elevated TBARS) corresponds well with the observed morphological changes in the mitochondria such as mitochondrial swelling, vacuolization, loss and disintegration of cristae. Earlier studies from our laboratory have reported insufficiency of high-energy phosphates in the myocardium of broilers developing CHF (Nain et al., 2008; Olkowski et al., Citation2007). Hence, we can argue that mitochondrial damage corresponds with the lowered energy reserve in the myocardium as the mitochondrion is the power house of the myocardial cell.
The heart is one of the greatest energy-consuming organs in the body, which requires a constant supply of oxygen to maintain its metabolic functions (Giordano, Citation2005). In the cardiac tissue, mitochondria comprise 30% of the cardiomyocyte volume (Sheeran & Pepe, Citation2006). The major sites of ROS formation are at complex I and complex III of the electron transport chain located in the inner membrane of mitochondria (Turrens & Boveris, Citation1980; Turrens et al., Citation1985). During normal metabolism, 1% to 2% of oxygen is converted to ROS. Hence, increased ROS or RNS production or decreased antioxidant defenses leads to oxidative stress.
α-KGDH, one of the key rate-limiting enzymes of the tricarboxylic acid cycle, is involved in energy synthesis pathways. Studies in rats have demonstrated that α-KGDH is a sensitive target of H2O2 (Nulton-Persson & Szweda, Citation2001), but this enzyme can also act as a source of H2O2 in the mitochondria (for a review see Tretter & Adam-Vizi, Citation2005). It has been demonstrated that α-KGDH may generate ROS, leading to a state of oxidative stress (Starkov et al., Citation2004; Tretter & Adam-Vizi, Citation2004). Although the activity of α-KGDH does not appear to be directly sensitive to H2O2, it is notable that this enzyme was sensitive to TBH. In this context it is important to stress that TBH can produce both alkoxyl and hydroxyl radicals, two of the most potent ROS.
CK is involved in energy transformation pathways. A sizable fraction of CK is present inside mitochondria (mi-CK) in addition to that present in sarcoplasm. The ATP synthesized inside the mitochondria is converted to phosphocreatine by mi-CK, later transported to sarcoplasm and reconverted to ATP by CK present in sarcoplasm. As CK and α-KGDH are susceptible to oxidative damage, the mi-CK and α-KGDH are more likely to be affected in vivo, due to its closeness to the site of ROS production—that is, present inside the mitochondria is the electron transport chain, a major source of free radicals.
In addition to the mitochondrial electron transport chain, xanthine oxidase is another important source of free radicals (Mekhfi et al., Citation1996). Xanthine oxidase has the ability to produce H2O2. In vitro studies have demonstrated that xanthine and xanthine oxidase had inhibitory effects on CK activity in heart tissues in a dose-dependent manner (Mekhfi et al., Citation1996; Genet et al., Citation2000).
In an anaerobic situation, LDH contributes to energy synthesis by anaerobic glycolysis. An increased production of ROS/RNS occurs during tissue hypoxia (Chen & Meyrick, Citation2004), which can negatively affect the activity of energy synthesis and transformation pathways. With hypoxia, activation of LDH enzyme by ROS may work as a force to counter the negative effect of other enzymes on energy synthesis and transformation pathways. Recently, we observed higher LDH activity in broilers developing CHF (Nain et al., 2008). Hence, increased activity of LDH in broilers developing CHF is most probably due to generated oxidative stress in the broilers.
Insufficiency of creatine phosphate and ATP leads to deterioration in heart pump function in broilers (Nain et al., 2008; Olkowski et al., Citation2007). However, the decline in these energy substrates could not be explained by changes in the end point kinetic activities of the rate-limiting enzymes such as α-KGDH and PDH (Nain et al., 2008). In view of the present findings it is entirely possible that the inhibition of α-KGDH and CK activity by oxidants can occur, regardless of their apparent kinetic activity in vivo (which may include compensatory up-regulation). This suggests that the observed decline in energy phosphates with deterioration in heart functions might be associated with the decreased activity of these enzymes during oxidative stress.
Our findings from the lipid peroxidation assay strongly suggest that oxidative stress is generated during development of heart failure. In fast-growing broilers, oxidative damage is probably associated with excessive generation of H2O2. Maxwell et al. (Citation1996) provide further supportive evidence as these authors demonstrated higher H2O2 activity in the cardiomyocytes from failing heart in broilers. However, as discussed above, several other enzymatic pathways may generate oxidants. Among the most relevant to oxidative damage in broiler heart is xanthine oxidase complex, which has very high activity in birds (Edson et al., Citation1936). This may be biochemically related to specific features of nitrogen metabolism in fast-growing broilers as uric acid is the end product of purine metabolism in all vertebrates, and in particular amino acid metabolism in birds. This reasoning is supported by the study of Enkvetchakul et al. (Citation1993) who found that uric acid levels are higher in broilers with CHF, providing indirect evidence that xanthine oxidase may be involved in the pathogenesis of heart failure in broilers.
Based on the findings from the present study, we conclude that the deterioration in heart pump function and ultrastructural lesions observed in the mitochondria are subsequent to oxidative stress, which affects mitochondrial energy generation and synthesis pathways, leading to lowered energy reserve in the myocardium.
Acknowledgements
The research presented in this paper was supported by grants provided by the Lilydale Hatchery, the Poultry Industry Council of Canada, and the Natural Sciences and Engineering Research Council of Canada.
References
- Andreka , P. , Tran , T. , Webster , K.A. and Bishopric , N.H. 2004 . Nitric oxide and promotion of cardiac myocyte apoptosis . Molecular and Cellular Biochemistry , 263 : 35 – 53 .
- Canadian Council on Animal Care 1993 . Guide to the Care and Use of Experimental Animals Vol. 1 and 2 . Ontario, Canada: CCAC, 1993 .
- Chen , J.X. and Meyrick , B. 2004 . Hypoxia increases Hsp90 binding to eNOS via PI3K-Akt in porcine coronary artery endothelium . Laboratory Investigation , 84 : 182 – 190 .
- Deng , G. , Zhang , Y. , Peng , X. , Guo , D. and Li , C. 2006 . Echocardiographic characteristics of chickens with ascites syndrome . British Poultry Science , 47 : 756 – 762 .
- Druyan , S. , Shlosberg , A. and Cahaner , A. 2007 . Evaluation of growth rate, body weight, heart rate, and blood parameters as potential indicators for selection against susceptibility to the ascites syndrome in young broilers . Poultry Science , 86 : 621 – 629 .
- Edson , N.L. , Krebs , H.A. and Model , A. 1936 . The synthesis of uric acid in the avian organism: hypoxanthine as an intermediary metabolite . The Biochemical Journal , 30 : 1380 – 1385 .
- Enkvetchakul , B. , Bottje , W. , Anthony , N. , Moore , R. and Huff , W. 1993 . Compromised antioxidant status associated with ascites in broilers . Poultry Science , 72 : 2272 – 2280 .
- Genet , S. , Kale , R.K. and Baquer , N.Z. 2000 . Effects of free radicals on cytosolic creatine kinase activities and protection by antioxidant enzymes and sulfhydryl compounds . Molecular and Cellular Biochemistry , 210 : 23 – 28 .
- Giordano , F.J. 2005 . Oxygen, oxidative stress, hypoxia and heart failure . The Journal of Clinical Investigation , 115 : 500 – 508 .
- Halliwell , B. and Gutteridge , J.M. 1985 . The importance of free radicals and catalytic metal ions in human diseases . Molecular Aspects of Medicine , 8 : 89 – 193 .
- Hintze , J. ( 1995 ). NCSS Statistical software . Kayville , UT : Number Cruncher Statistical System, Dr Jerry L. Hintze .
- Li , K. , Qiao , J. , Zhao , L. , Dong , S. , Ou , D. , Wang , J. , Wang , H. and Xu , T. 2006 . Increased calcium deposits and decreased Ca2 + -ATPase in right ventricular myocardium of ascitic broiler chickens . Journal of Veterinary Medicine A , 53 : 458 – 463 .
- Lykkesfeldt , J. and Svendsen , O. 2007 . Oxidants and antioxidants in disease: oxidative stress in farm animals . The Veterinary Journal , 173 : 502 – 511 .
- Maxwell , M.H. , Robertson , G.W. and Mitchell , M.A. 1993 . Ultrastructural demonstration of mitochondrial calcium overload in myocardial cells from broiler chickens with ascites and induced hypoxia . Research in Veterinary Science , 54 : 267 – 277 .
- Maxwell , M.H. , Robertson , G.W. and Moseley , D. 1994 . Potential role of serum troponin T in cardiomyocyte injury in the broiler ascites syndrome . British Poultry Science , 35 : 663 – 667 .
- Maxwell , M.H. , Robertson , G.W. and Farquharson , C. 1996 . Evidence of ultracytochemical mitochondria-derived hydrogen peroxide activity in myocardial cells from broiler chickens with an ascites syndrome . Research in Veterinary Science , 61 : 7 – 12 .
- Mekhfi , H. , Veksler , V. , Mateo , P. , Maupoil , V. , Rochette , L. and Ventura-Clapier , R. 1996 . Creatine kinase is the main target of reactive oxygen species in cardiac myofibrils . Circulation Research , 78 : 1016 – 1027 .
- Nain , S. , Ling , B.B. Wojnarowicz , C. Laarveld , B. , Alcorn , J. & Olkowski , A.A. , ( 2008 ). Biochemical factors limiting myocardial energy in a chicken genotype selected for rapid growth . Comparative Biochemistry and Physiology. Part A , 149 ( 1 ), 36 – 43 .
- Nediani , C. , Borchi , E. , Giordano , C. , Baruzzo , S. , Ponziani , V. , Sebastiani , M. , Nassi , P. , Mugelli , A. , d'Amati , G. and Cerbai , E. 2007 . NADPH oxidase-dependent redox signaling in human heart failure: relationship between the left and right ventricle . Journal of Molecular and Cellular Cardiology , 42 ( 4 ) : 826 – 834 .
- Nulton-Persson , A.C. and Szweda , L.I. 2001 . Modulation of mitochondrial function by hydrogen peroxide . The Journal of Biological Chemistry , 276 ( 26 ) : 23357 – 23361 .
- Ohkawa , H. , Ohishi , N. and Yagi , K. 1979 . Assay for lipid peroxides in animal tissues by thiobarbituric acid reaction . Analytical Biochemistry , 95 : 351 – 358 .
- Olkowski , A.A. and Classen , H.L. 1998 . Progressive bradycardia, a possible primary factor in the pathogenesis of ascites in fast growing broiler chickens raised at low altitude . British Poultry Science , 39 : 139 – 146 .
- Olkowski , A.A. , Korver , D. , Rathgeber , B. and Classen , H.L. 1999 . Cardiac index, oxygen delivery, and tissue oxygen extraction in slow growing and fast growing chickens, and in chickens with heart failure and ascites: a comparative study . Avian Pathology , 28 : 137 – 146 .
- Olkowski , A.A. , Rathgeber , B.M. , Sawicki , G. and Classen , H.L. 2001 . Ultrastructural and molecular changes in the left and right ventricular myocardium associated with ascites syndrome in broiler chickens raised at low altitude . Journal of Veterinary Medicine A , 48 : 1 – 14 .
- Olkowski , A.A. , Duke , T. and Wojnarowicz , C. 2005 . The aetiology of hypoxaemia in chickens selected for rapid growth . Comparative Biochemistry and Physiology. Part A , 141 : 122 – 131 .
- Olkowski , A.A. , Nain , S. , Wojnarowicz , C. , Laarveld , B. , Alcorn , J. and Ling , B.B. 2007 . Comparative study of myocardial high energy phosphate substrate content in slow and fast growing chicken and in chickens with heart failure and ascites . Comparative Biochemistry and Physiology. Part A , 148 : 230 – 238 .
- Orrenius , S. , Gogvadze , V. and Zhivotovsky , B. 2007 . Mitochondrial oxidative stress: implications for cell death . Annual Review of Pharmacology and Toxicology , 47 : 143 – 183 .
- Redout , E.M. , Wagner , M.J. , Zuidwijk , M.J. , Boer , C. , Musters , R.J. , van Hardeveld , C. , Paulus , W.J. and Simonides , W.S. 2007 . Right-ventricular failure is associated with increased mitochondrial complex II activity and production of reactive oxygen species . Cardiovasc Research , 75 ( 4 ) : 770 – 781 .
- Sam , F. , Kerstetter , D.L. , Pimental , D.R. , Mulukutla , S. , Tabaee , A. , Bristow , M.R. , Colucci , W.S. and Sawyer , D.B. 2005 . Increased reactive oxygen species production and functional alterations in antioxidant enzymes in human failing myocardium . Journal of Cardiac Failure , 11 ( 6 ) : 473 – 480 .
- Sheeran , F.L. and Pepe , S. 2006 . Energy deficiency in the failing heart: linking increased reactive oxygen species and disruption of oxidative phosphorylation rate . Biochimica et Biophysica Acta , 1757 : 543 – 552 .
- Starkov , A.A. , Fiskum , G. , Chinopoulos , C. , Lorenzo , B.J. , Browne , S.E. , Patel , M.S. and Beal , M.F. 2004 . Mitochondrial alpha-ketoglutarate dehydrogenase complex generates reactive oxygen species . The Journal of Neuroscience , 24 ( 36 ) : 7779 – 7788 .
- Tretter , L. and Adam-Vizi , V. 2004 . Generation of reactive oxygen species in the reaction catalyzed by alphaketoglutarate dehydrogenase . The Journal of Neuroscience , 24 ( 36 ) : 7771 – 7778 .
- Tretter , L. and Adam-Vizi , V. 2005 . Alpha-ketoglutarate dehydrogenase: a target and generator of oxidative stress . Philosophical Transactions of the Royal Society of London Series B , 360 ( 1464 ) : 2335 – 2345 .
- Turrens , J.F. and Boveris , A. 1980 . Generation of superoxide anion by the NADH dehydrogenase of bovine heart mitochondria . The Biochemical Journal , 191 : 421 – 427 .
- Turrens , J.F. , Alexandre , A. and Lehninger , A.L. 1985 . Ubisemiquinone is the electron donor for superoxide formation by complex III of heart mitochondria . Archives of Biochemistry and Biophysics , 237 : 408 – 414 .