Abstract
This study is aimed at determining the antimicrobial resistance (AMR) and the presence of class 1 and 2 integrons in 48 avian pathogenic Escherichia coli (APEC) strains isolated from meat turkeys during three sequential production cycles. Thirty avian faecal E. coli (AFEC) strains from the first cycle were also analysed. Strains were tested for AMR against 25 antimicrobials by disk diffusion test and were screened for the presence of integrons and associated gene cassettes by polymerase chain reaction followed by sequencing. Genetic relatedness of isolates was established by pulsed-field gel electrophoresis. High levels of resistance were detected to tetracyclines, penicillins and sulphonamides in APEC and AFEC. Resistance to aminoglycosides, fluoroquinolones, cephalosporins and phenicols was variable, based on the antimicrobial drug and the isolate (APEC vs. AFEC). Full susceptibility to colistin was detected. Multidrug resistance of up to seven antimicrobial classes was exhibited by APEC (93.8%) and AFEC (100%). Nearly 44% of strains tested positive for class 1 and/or class 2 integrons containing the dfrA, aadA and sat2 genes, alone or in combination, coding for streptomycin/spectinomycin, trimethoprim and streptothricin resistance, respectively. The estX and orfF genes of unknown function were also detected. A significant association was found between the presence of integrons and the resistance to aminoglycosides and potentiated sulphonamides. The results of this study showed that AMR, multidrug resistance and class 1 and 2 integrons are widespread among pathogenic and commensal E. coli from Italian turkeys. More attention should be addressed to limit the use of antimicrobials in turkeys and the AMR of turkey E. coli.
Introduction
Avian colibacillosis is a localized or systemic disease of poultry caused by avian pathogenic Escherichia coli (APEC) and one of the major causes of economic losses in the poultry industry worldwide (Barnes et al., Citation2008). E. coli is a common inhabitant of the intestinal microflora of poultry at abundances of up to 106 colony-forming units per gram of faeces. In healthy chickens, 10 to 15% of faecal coliforms may belong to potentially pathogenic serogroups, carrying virulence and antimicrobial resistance genes (Barnes et al., Citation2008). Serogroups most frequently associated with disease in poultry are O1, O2, O35, O36 and O78 (Dho-Moulin & Fairbrother, Citation1999). Prevention and control of colibacillosis in poultry are addressed at reducing or eliminating the introduction of APEC into the poultry house, protecting the birds from predisposing factors associated with infection, immunizing the birds by use of inactivated and live vaccines and treating birds with antimicrobial agents (Barnes et al., Citation2008; Gyles, Citation2008). Since management and vaccination procedures are not always effective, antimicrobial therapy represents the most common intervention used to reduce losses from avian colibacillosis. However, APEC strains are increasingly becoming resistant to a wide range of antimicrobial drugs (Singer & Hofacre, Citation2006; Gyles, Citation2008), indicating that the protection against avian colibacillosis in poultry could be impaired in the near future.
The spread of antimicrobial resistance among bacterial populations via horizontal gene transfer represents a serious threat both in veterinary and human medicine (FAO/WHO/OIE, Citation2008). Recently, integrons have been shown to play a significant role in the acquisition and the dissemination of antimicrobial resistance among bacteria, especially when associated with mobile DNA elements, such as transposons and plasmids (Mazel, Citation2006). Integrons constitute a site-specific recombination system capable of capturing and expressing exogenous open reading frames in the form of gene cassettes, encoding for resistance against antimicrobials and disinfectants (Hall, Citation2012). According to the sequence homology of the integrase genes, encoding the enzymes mediating the integration and the excision of gene cassettes, several distinct classes of integrons have been described to date (Partridge et al., Citation2009; Hall, Citation2012). Class 1 integrons are the most frequently detected and the best characterized, mainly among the members of the family of Enterobacteriaceae; class 2 integrons have been observed only in a few Gram-negative microorganisms (Partridge et al., Citation2009).
Related to the important role played by integrons in the horizontal transfer of antimicrobial resistance genes, over the past years many studies have been conducted to detect their presence in commensal and pathogenic E. coli from humans and animals (Partridge et al., Citation2009; Hall, Citation2012). Nevertheless, little is known about the distribution of integrons in E. coli isolated from commercial meat turkeys. Only a few studies have been carried throughout the world and most of them are focused on E. coli isolated from turkey meat (Johnson et al., Citation2005; Khaitsa et al., Citation2008; Cook et al., Citation2009; Soufi et al., Citation2009, Citation2011). To our knowledge, studies targeted at revealing the distribution and the gene cassette content of class 1 and 2 integrons in E. coli from intensive turkey flocks are not available in the literature. Therefore, the aims of this study were to determine the antimicrobial resistance profiles, the distribution of class 1 and class 2 integrons and the integron-associated gene cassettes in APEC strains isolated from diseased turkeys in Italy. Analyses were also performed in a number of avian faecal E. coli (AFEC) strains isolated from healthy birds. All strains were characterized by pulsed-field gel electrophoresis (PFGE) in order to determine the genetic relationship among them.
Materials and Methods
Sample collection
Between June 2009 and July 2010, dead male turkeys (≤14 weeks old) from three sequential production cycles in a single commercial farm were submitted to post-mortem examination. Total mortality of flocks ranged from 6.5% in the first production cycle to 8.0% in the second cycle and 7.0% in the third cycle. A downtime of 21 days among the different production cycles was implemented in the farm, during which the turkey house was cleaned and disinfected, and litter was removed. Pathological lesions were clearly indicative of colisepticaemia with pericarditis, pneumonia and airsacculitis, or arthritis. Samples from all carcasses, viscera (i.e. brain, pericardial sac, liver, lungs) and joints were collected for microbiological examination. Further, cloacal swabs from healthy turkeys of the first production cycle were collected in order to isolate faecal E. coli. No information on therapeutic antimicrobial usage in the flocks was recorded.
E. coli isolation, identification and serogrouping
Swabs from viscera, joints and cloaca were individually cultured onto 5% sheep blood agar (OXOID, Basingstoke, UK) and Eosin-Methylene Blue agar (OXOID), and were incubated aerobically at 37°C for 18 to 24 h. One presumptive E. coli colony from each sample was subcultured on tryptic soy agar (OXOID) and then biochemically identified using the commercial kit RapID E20 (bioMérieux Vitek, Hazelwood, MO, USA). Serogroups were determined according to the technique proposed by Blanco et al. (Citation1992), identifying 37 different somatic antigens.
Pulsed-field gel electrophoresis
All E. coli strains were subjected to PFGE typing according to the protocol of Ribot et al. (Citation2006), modified slightly. Electrophoresis separation of DNAs was performed in the CHEF-DRIII PFGE System (Biorad, Hercules, California, USA) and images captured using Gel Doc EZ Imager (Biorad). E. coli ATCC 25922 was used as reference strain. Interpretation of PFGE patterns was performed with GelCompar II version 6.6.11 (Applied Maths, St-Martens-Latem, Belgium) and similarity was determined using the Dice similarity coefficient. Isolates were considered to be related if their Dice similarity index was ≥80% and according to the criteria defined by Tenover (≤6 bands of difference) (Tenover et al., Citation1995).
Antimicrobial susceptibility testing
All E. coli strains were tested for antimicrobial susceptibility by the disk diffusion method, according to CLSI (Citation2008) standards, against 25 antimicrobial drugs belonging to eight classes: aminoglycosides (aminosidine, 60 µg; apramycin, 15 µg; gentamicin, 10 µg; spectinomycin, 10 µg; streptomycin, 10 µg; kanamycin, 30 µg), cephalosporins (cephalotin, 30 µg; ceftiofur, 30 µg; cefotaxime, 30 µg; ceftazidime, 30 µg; cefepime, 30 µg), fluoroquinolones (nalidixic acid, 30 µg; enrofloxacin, 5 µg; ciprofloxacin, 5 µg; norfloxacin, 10 µg), penicillins (ampicillin, 10 µg; amoxicillin + clavulanic acid, 30 µg), phenicols (chloramphenicol 30 µg; florfenicol, 30 µg), potentiated sulphonamides (triple sulphonamides, 300 µg; trimethoprim + sulfamethoxazole, 25 µg), tetracyclines (tetracycline, 30 µg; oxytetracycline, 30 µg; doxycycline, 30 µg) and polymyxins (colistin, 10 µg). All antimicrobial-containing disks were obtained from OXOID. E. coli ATCC 25922 was used as quality control strain. The level of antimicrobial resistance was defined as the percentage of resistant isolates as a proportion of the isolates tested; multidrug resistance (MDR) was defined as resistance to ≥3 different antimicrobial classes where resistance was detected against at least one antimicrobial agent. Penicillins and cephalosporins were considered separate classes.
Detection of class 1 and class 2 integrons and characterization of gene cassettes
Total DNA was extracted by boiling for 20 min of a single colony emulsified in 100 µl sterile RNase/DNase free water (Sigma-Aldrich, Milan, Italy). All E. coli isolates were screened for the presence of class 1 and class 2 integrons by real-time polymerase chain reaction (PCR) assay using previously described primer sets (Ekkapobyotin et al., Citation2008) and the protocol designed by Piccirillo et al. (Citation2013). Real-time PCR amplifications were performed in a LightCycler®480 Real-Time PCR System (Roche Diagnostics, Basel, Switzerland). All class 1 and class 2 integron-positive strains were examined by sequencing to determine the gene cassette content using the primers and the protocols suggested by Lévesque et al. (Citation1995) and White et al. (Citation2001). Both strands were sequenced using the BigDye Terminator Cycle Sequencing Kit v3.1 in the ABI PRISM 3100 Genetic Analyzer (Applied Biosystems, Foster City, CA, USA), according to the manufacturer's instructions. Editing of chromatograms and assembly of nucleotide sequences were performed by the software ChromasPro v. 1.42 (Technelysium Pty Ltd, Tewantin, Australia). Gene cassettes were identified by the BLASTN software available online (http://blast.ncbi.nlm.nih.gov/).
Statistical analysis
To test for significance in the difference of resistance between APEC and AFEC, among the three production cycles and among the serogroups, as well as in the association between resistance and presence of integrons, the intermediate isolates were grouped together with the susceptible isolates and the Fisher's exact test was performed using the General Linear Model (GLM) procedure (SAS Institute Inc., Cary, NC, USA). P ≤ 0.05 was considered statistically significant.
Results
E. coli isolation, identification and serogrouping
From 37 carcasses, 48 APEC strains were isolated: 10 from the first production cycle, 28 from the second cycle and 10 from the third cycle (). Most strains were isolated from the brain (22/48 isolates), followed by the pericardium (7/48 isolates), lungs (7/48 isolates), tibiotarsal (6/48 isolates) and coxofemoral (4/48 isolates) joints, liver (1/48 isolate) and spleen (1/48 isolate). Twenty-eight isolates belonged to the O78 serogroup, 14 isolates to the O2 serogroup, one isolate to the O111 serogroup, and five isolates were untypeable with standard O antisera. Thirty AFEC isolates from faecal samples from healthy birds of the first production cycle were also obtained ().
Table 1. Antimicrobial resistance, class 1 and 2 integrons and gene cassette content of APEC isolates.
Table 2. Antimicrobial resistance, class 1 and 2 integrons and gene cassette content of AFEC isolates.
Pulsed-field gel electrophoresis analysis
All APEC and AFEC isolates except for one and three, respectively, were successfully analysed by PFGE. Twenty-eight different PFGE types were identified: 11 for APEC strains and 17 for AFEC strains (). Among APEC isolates, three PFGE types were detected in the first production cycle, seven types in the second cycle and two types in the third cycle. One PFGE type (i.e. XVI) was shared among strains of the first and the second production cycles. Higher strain diversity was detected in AFEC compared with APEC (Simpson's index of diversity = 0.94 and 0.86, respectively), with no overlapping among their PFGE types. Among APEC and AFEC isolates, four and 13 PFGE types were represented by single strains, respectively.
Antimicrobial resistance of APEC and AFEC isolates
Overall, except for a few drugs (i.e. nalidixic acid, ciprofloxacin, and florfenicol), AFEC showed higher percentages of resistance than APEC (). Most isolates, both APEC and AFEC, were resistant to tetracyclines (95.8% for all the drugs in APEC; 96.7% for doxycycline to 100% for tetracycline and oxytetracycline in AFEC), as well as to penicillins (95.8% for all the drugs in APEC; from 90% for amoxicillin + clavulanic acid to 100% for ampicillin in AFEC). A high percentage of AFEC was also resistant to potentiated sulphonamides (80% for trimethoprim + sulfamethoxazole and 90% for triple sulphonamides), whereas APEC were less resistant to both antimicrobials (47.9% and 58.3%, respectively). Conversely, APEC exhibited higher frequencies of resistance to fluoroquinolones than AFEC (from 6.2% for enrofloxacin and ciprofloxacin to 93.7% for nalidixic acid and from 3.3% ciprofloxacin to 46.7% for nalidixic acid, respectively). The percentage of resistance to aminoglycosides ranged from 0% for apramycin to 75% for streptomycin among APEC and from 6.7% for apramycin to 86.7% for streptomycin among AFEC. Resistance to cephalosporins was higher in AFEC (from 3.3% for ceftazidime to 43.3% for cephalotin) than APEC (from 0% for 3 cephalosporins to 31.2% for cephalotin). Low levels of resistance were detected to phenicols, both in APEC (8.3% for chloramphenicol and 4.2% for florfenicol) and in AFEC (36.7% for chloramphenicol and 0% for florfenicol). All isolates were fully susceptible to colistin. The resistance rates to triple sulphonamides, trimethoprim combined with sulfamethoxazole, gentamicin, kanamycin, aminosidine, ceftiofur, cefotaxime, and chloramphenicol of AFEC were significantly (P ≤ 0.05) higher compared with APEC; resistance to nalidixic acid was significantly (P ≤ 0.05) higher in APEC than in AFEC. The prevalence of resistance of APEC to triple sulphonamides, trimethoprim combined with sulfamethoxazole, ampicillin, amoxicillin combined with clavulanic acid, streptomycin, and spectinomycin increased significantly (P ≤ 0.05) over the three production cycles; the prevalence of resistance to florfenicol decreased significantly (P ≤ 0.05) over the same interval. No significant differences (P > 0.05) in resistance rates were observed among serogroups of APEC.
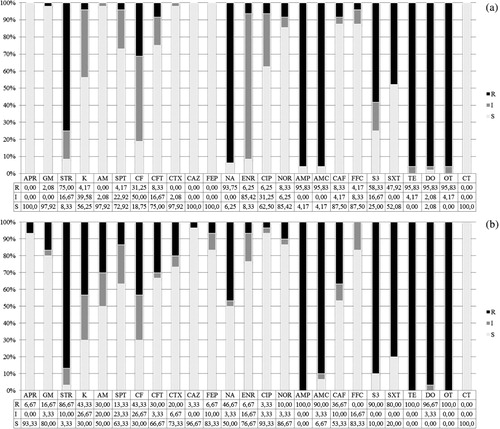
The majority (96.1%) of E. coli isolated from diseased and healthy birds exhibited resistance to three or more antimicrobial classes ( and ). Only three APEC isolates showed resistance to one and two classes of antimicrobials, and none was fully susceptible. In particular, among the 48 APEC isolates, three (6.2%), nine (18.7%), 22 (45.8%), 10 (20.8%) and one (2.1%) isolates showed MDR to three, four, five, six and seven antimicrobial classes, respectively. Out of the 30 AFEC, three (10%), eight (26.7%), six (20%), eight (26.7%) and five (16.7%) isolates were resistant to three, four, five, six and seven antimicrobial classes, respectively. MDR patterns comprised 19 different combinations, with the most common including five classes (i.e. aminoglycosides, fluoroquinolones, penicillins, sulphonamides and tetracyclines) among APEC (18/48 isolates) and four classes (i.e. aminoglycosides, penicillins, sulphonamides and tetracyclines) among AFEC (7/30 isolates).
Presence of class 1 and 2 integrons and gene cassettes content in APEC and AFEC isolates
Real-time PCR screening for intI1 and intI2 genes identified 34 (43.6%) strains carrying class 1 and/or class 2 integrons ( and ). Out of the 48 APEC isolates, the intI1 gene was detected in six (12.5%) isolates and the intI2 gene in nine (18.7%) isolates. Among the 30 AFEC isolates, 19/30 (63.3%) strains were positive for class 1 integrons and only two (6.7%) for class 2 integrons. Two strains (one APEC and one AFEC) carried both classes of integrons. The prevalence of class 1 integrons was significantly higher (P ≤ 0.001) in AFEC as compared with APEC, while that of class 2 integrons was not significantly (P > 0.05) different between APEC and AFEC. Prevalence of integrons was quite different among APEC of the various production cycles and serogroups. Indeed, none of the isolates of the first production cycle carried integrons, whereas isolates from the second and third production cycles were positive (6/28 and 8/10 isolates, respectively). Only class 1 integrons were identified in isolates from the second cycle, except for one strain carrying both classes of integrons; isolates from the third cycle carried only class 2 integrons. Furthermore, most of the intI1-positive and intI2-positive strains belonged to the O2 serogroup (11/14), 2/14 strains were untypeable and only one strain belonged to the O78 serogroup.
As shown in and , 10 different gene cassettes including aadA (variants aadA1 and aadA2), dfrA (variants dfrA1, dfrA7, dfrA12), sat2, orfF and estX genes, alone or in combination, were identified among APEC and AFEC. The aadA genes encode for the aminoglycoside adenylyltransferases (streptomycin/spectinomycin resistance), the dfrA genes for the dihydrofolate reductases (trimethoprim resistance), the sat2 gene for the streptothricin acetyltransferases, the estX gene for a putative esterase, and the orfF gene for a protein of unknown function. The most common cassette array was dfrA1-aadA1 carried by 11 intI1-positive AFEC and four APEC strains, followed by the dfrA1-sat2-aadA1 array carried by eight intI2-positive APEC strains. Isolates carrying both classes of integrons harboured the aadA1 and sat2-aadA1 genes (APEC) and the sat2 and sat2-aadA1 genes (AFEC) in class 1 and 2 integrons, respectively. Diversity of gene cassette arrays was higher in AFEC than APEC (seven vs. five different cassette arrays, respectively). In APEC isolates from the second production cycle, the most prevalent array was dfrA1-aadA1, whereas in intI2-positive APEC isolates from the third production cycle only one array (dfrA1-sat2-aadA1) was identified. The only intI1-positive O78 serogroup carried an unusual cassette array, the estX-aadA1.
Association between presence of integrons and antimicrobial resistance in E. coli isolates
For a clear assessment of the relation between antimicrobial resistance and integrons, resistance determinants not associated with integrons were excluded from the analysis. The differences between intI1-positive and intI1-negative isolates in resistance to gentamycin, streptomycin, aminosidine, triple sulphonamides and trimethoprim combined with sulfamethoxazole were significantly (P ≤ 0.05) higher in intI1-positive compared with intI1-negative isolates. The differences in intI2-positive and intI2-negative isolates in resistance to spectinomycin, triple sulphonamides and trimethoprim combined with sulfamethoxazole were significantly (P ≤ 0.05) higher in intI2-positive compared with intI2-negative isolates. All intI1-positive and intI2-positive E. coli isolates were MDR, while intI1-negative and intI2-negative isolates were 93.2%.
Discussion
Currently available studies showed that high resistance and multidrug resistance are common among avian pathogenic E. coli from turkeys (Salmon & Watts, Citation2000; Cormican et al., Citation2001; van den Bogaard et al., Citation2001; Altekruse et al., Citation2002; EFSA, Citation2010; Randall et al., Citation2011, Citation2013; Gosling et al., Citation2012). In the current study, a very high level of resistance to several antimicrobial drugs was detected and multidrug resistance to three or more antimicrobial classes was widespread both among APEC and AFEC. Moreover, a high percentage of isolates was found positive for class 1 and 2 integrons. Although some data on the presence and characterization of integrons in turkey E. coli are available in the literature (Bass et al., Citation1999; Singh et al., Citation2005; Nógrády et al., Citation2006), none of these studies was targeted to ascertain the distribution of these genetic elements in turkey flocks. Therefore, with this study we provide the first data on the presence and the gene cassette content of class 1 and 2 integrons in E. coli isolated from commercial meat turkey flocks.
The highest levels of resistance were detected against tetracyclines, penicillins and sulphonamides, as widely documented among pathogenic and commensal E. coli of turkey origin (Salmon & Watts, Citation2000; Cormican et al., Citation2001; van den Bogaard et al., Citation2001; Altekruse et al., Citation2002; Lister, Citation2005; EFSA, Citation2010; Randall et al., Citation2011, Citation2013; Gosling et al., Citation2012). The high levels of resistance to these classes may be attributed to a long-term selection pressure, since these antimicrobials are among the oldest and the most extensively used in the poultry industry all over the world (Singer & Hofacre, Citation2006), including Italy. In contrast, the very high resistance rates to streptomycin and nalidixic acid were unexpected since these drugs have not been used for a long time in our country. Similarly to our findings, a widespread resistance to these antimicrobials has been documented in turkey APEC and AFEC (Cormican et al., Citation2001; Giraud et al., Citation2001; Altekruse et al., Citation2002; Bryan et al., Citation2004; EFSA, Citation2010; Randall et al., Citation2011, Citation2013; Gosling et al., Citation2012). However, it has been reported that the resistance to specific antimicrobials, such as streptomycin, may be prevalent despite its discontinuance as a therapeutic agent (Bass et al., Citation1999) and the development of resistance to nalidixic acid may not be necessarily linked with treatment (Hofacre et al., Citation2000).
In accordance with previous data (Salmon & Watts, Citation2000; Cormican et al., Citation2001; Lister, Citation2005; EFSA, Citation2010; Randall et al., Citation2011, Citation2013; Gosling et al., Citation2012; Kempf et al., Citation2013a), a prevailing susceptibility against several members of the aminoglycoside family (streptomycin excepted) was found in E. coli from turkeys, mainly among APEC. This finding was expected since these antimicrobials are scarcely employed in the turkey industry in Italy. Similarly, low levels of resistance to fluoroquinolones (except for nalidixic acid) were found. Partially contrasting results regarding fluoroquinolone resistance can be found in the literature (Salmon & Watts, Citation2000; Cormican et al., Citation2001; Giraud et al., Citation2001; van den Bogaard et al., Citation2001; Altekruse et al., Citation2002; Lister, Citation2005; Taylor et al., Citation2008; EFSA, Citation2010; Randall et al., Citation2011, Citation2013; Gosling et al., Citation2012). It has been recognized that fluoroquinolone resistance can be related to the usage pattern of these drugs in a given country, which is in turn influenced by the market dynamics, mainly the cost of commercial products (Bywater, Citation2005). In Italy, the parsimonious use of costly products, together with the policy of the integrated company, may have limited the development of fluoroquinolone resistance in E. coli examined in the present study.
A low susceptibility to cephalotin and ceftiofur was detected in both APEC and AFEC. Resistance to third-generation and fourth-generation cephalosporins of clinical and faecal turkey E. coli has been previously reported (Salmon & Watts, Citation2000; Altekruse et al., Citation2002; Randall et al., Citation2011, Citation2013; Gosling et al., Citation2012). Dutil et al. (Citation2010) demonstrated that ceftiofur resistance was related to the use of this drug in poultry hatcheries. Although cephalosporins are not approved for use in poultry, the administration of this drug in 1-day-old chicks at the hatchery cannot be excluded. One should emphasize that the predominant susceptibility to third-generation and fourth-generation cephalosporins, as well as to fluoroquinolones, is of great value from a public health point of view given the importance of these antimicrobials as critically important antibiotics for human medicine (WHO, Citation2012).
The moderate levels of resistance to chloramphenicol and florfenicol detected in our study are unclear, since the first drug is not approved for use in food-producing animals in Europe (Commission Regulation No. 37/2010 of 22 December Citation2009) and the second is not authorized for use in poultry in Italy. However, this finding has been previously documented in other studies (Salmon & Watts, Citation2000; Cormican et al., Citation2001; Altekruse et al., Citation2002; EFSA, Citation2010; Randall et al., Citation2011, Citation2013; Gosling et al., Citation2012). Finally, the full susceptibility to colistin observed in E. coli is valuable both for poultry and human concerns, since this agent is widely used in poultry production and represents a last-resort treatment in human medicine (Livermore, Citation2004). In accordance with our results, colistin resistance seems to be extremely rare (<1%) in healthy animals, including poultry, in Europe (Kempf et al., Citation2013b).
The resistance to the majority of antimicrobials tested in this study is known to be conferred by the acquisition of foreign resistance genes through horizontal transfer, which usually emerges in the presence of antimicrobial selective pressure and involves not only pathogenic but also commensal bacteria (Schwarz et al., Citation2006; van Hoek et al., Citation2011). It is likewise well known that antimicrobial resistance and multidrug resistance in E. coli is acquired by the transfer of mobile genetic elements, such as gene cassettes in integrons (Leverstein-van Hall et al., Citation2003; Fluit & Schmitz, Citation2004; Mazel, Citation2006). In the present study, almost one-half of the isolates (APEC and AFEC) carried class 1 and/or class 2 integrons. In a large study on several poultry species including turkeys, Bass et al. (Citation1999) found that 63% (n = 100) of avian pathogenic E. coli isolates were positive for class 1 integrons. Further data suggest similar class 1 integron prevalence among APEC and AFEC from turkeys (Nógrády et al., Citation2006). Although a predominance of class 1 integron-positive strains was detected, a large number of class 2 integron-positive strains was found, with two strains positive for both classes. These findings are noteworthy since class 2 integrons are usually considered rare and strains carrying more than one class of integrons are even rarer (Mazel, Citation2006; Partridge et al., Citation2009). To our knowledge, this is the first report of the detection and the identification of class 2 integrons in turkey pathogenic and commensal E. coli. Characterization of integron-borne gene cassettes showed that, besides two genes (estX and orfF) encoding for proteins of unknown function, the streptomycin/spectinomycin (aadA), the streptothricin (sat2) and the trimethoprim (dfrA) resistance genes were present in integron-positive isolates. All of these gene cassettes, alone or in array, are among the most commonly found in both class 1 and class 2 integrons, whereas the estX and the orfF genes have been rarely reported (Partridge et al., Citation2009). Other studies looking at the presence of class 1 integrons in clinical and commensal E. coli isolates from turkeys (Bass et al., Citation1999; Singh et al., Citation2005; Nógrády et al., Citation2006) found that the dominant gene cassette was aadA1.
All strains harbouring class 1 and/or class 2 integrons showed a multidrug resistant profile (four to seven antimicrobial classes) and a significant association between the presence of the integron and the resistance phenotype to streptomycin and potentiated sulphonamides was detected. Indeed, most of the E. coli strains positive for integrons and carrying the aadA and the dfrA genes were resistant to these antimicrobials. On the other hand, the presence of the aadA gene did not correlate to the spectinomycin resistance phenotype in a large proportion of integron-positive isolates. These findings may be explained by a low level or a lack of expression of the aadA gene, as documented previously (Singh et al., Citation2005). In contrast, a large number of integron-negative isolates was phenotypically resistant to these antimicrobials. These results may be attributed to other genetic mechanisms, not necessarily associated with the presence of integrons (van Hoek et al., Citation2011).
A significant increasing resistance from the first to the third production cycle, namely to streptomycin and spectinomycin and to potentiated sulphonamides, was observed in APEC. Concurrently, the presence of integrons increased over the three production cycles; indeed, no integrons were detected in APEC during the first production cycle, whereas these genetic elements were spread among strains from the other two cycles. This finding provides further evidence that phenotypic resistance to aminoglycosides and potentiated sulphonamides may be linked to the presence of integrons. This hypothesis is in line with the general belief that integrons contribute to the resistance phenotypes against these antimicrobials (Bass et al., Citation1999; Leverstein-van Hall et al., Citation2003). Moreover, according to Bass et al. (Citation1999)—who demonstrated a link between class 1 integrons in APEC and a continued resistance to old antimicrobials (i.e. streptomycin and sulphonamides)—the presence of integrons in our E. coli isolates may explain their resistance to antimicrobials, such as streptomycin, which has been discontinued from use in turkeys for a long time.
Results of our study indicate that commensal E. coli strains are more resistant than pathogenic strains and show a higher percentage of integron positivity and a more variable gene cassette content. Few studies comparing the antimicrobial resistance profiles between APEC and AFEC isolated from turkeys of the same farm have been published. In contrast to our findings, Altekruse et al. (Citation2002) reported that more clinical than faecal E. coli strains were resistant to several antimicrobials, suggesting that resistance in APEC might have been related to the selective pressure exerted by the antimicrobial usage in the turkey industry. Furthermore, Nógrády et al. (Citation2006) identified a higher frequency of class 1 integrons in APEC from chickens and turkeys, whereas more variable resistance patterns, a greater spectrum of resistance and a higher gene cassette variability were detected in AFEC.
No significant differences in resistance were observed among the various serogroups identified in this study. In contrast to Nógrády et al. (Citation2006), who reported a predominance of integron positivity in E. coli belonging to the O78 serogroup, we found that, although the O78 serogroup was prevalent among our isolates, only one strain was positive for class 1 integrons. Furthermore, this strain harboured an unusual cassette array associated with class 1 integrons, the estX-aadA1 array. Indeed, the estX gene has usually been reported in class 2 integrons and encodes for a hypothetical protein, whose function is unknown (Partridge & Hall, Citation2005). On the other hand, the majority of intI1-positive and intI2-positive APEC belonged to the serogroup O2. This finding needs to be explored further, since a potential linkage between the antimicrobial resistance genes and the virulence traits could exist in this serogroup, as previously reported by Johnson et al. (Citation2002).
PFGE analysis showed that isolates with different antimicrobial resistance profiles and integron content were closely related to each other. Conversely, common resistance profiles and integrons were shared among isolates belonging to different PFGE types. This difference in antimicrobial susceptibility may be explained by horizontal transmission of resistance determinants among strains, as documented previously by Ozaki et al. (Citation2011). Further research is needed to ascertain the localization of integrons either on chromosome or plasmids in order to understand their contribution to the dissemination of resistance. Furthermore, the results of this study showed that the same turkey flock and even the same bird were infected by strain types harbouring different antimicrobial resistance determinants. Representative samples of the population should therefore be collected to monitor the antimicrobial resistance of E. coli in order to make the right decisions regarding the therapeutic options and to reduce the risks of antimicrobial resistance.
The development of antimicrobial resistance is a complex phenomenon that involves several different mechanisms (Schwarz et al., Citation2006; van Hoek et al., Citation2011). In this study, we investigated only one of these mechanisms (i.e. integrons and gene cassettes), and found that antimicrobial resistance associated with the presence of these genetic elements was widespread among pathogenic and commensal E. coli from Italian meat turkeys. Although much remains to be answered, we would like to underline that integrons play a crucial role in the horizontal transfer of resistance genes among bacteria and may persist into the environment also when the selective pressure of antimicrobials is low or absent. Our study shows that meat turkeys can play a role in the dissemination of antimicrobial resistance among bacterial populations, and thus considerable efforts need to be made on the prudent use of antimicrobials in turkey production and on the control of antimicrobial resistance both for bird and human health concerns.
Acknowledgements
The present study was supported by grants from the University of Padua (CPDA095771/09) and from the Italian Ministry of Higher Education and Research (PRIN 2009—2009R4KM4F_002). The authors wish to thank Dr Giulia Niero (University of Padua) for technical assistance, Dr Angela Trocino (University of Padua, Italy) for statistical analysis, and Dr Marianne Sunde (National Veterinary Institute, Norway), Dr Stefan Schwarz (Friedrich-Loeffler Institut, Germany), Dr Beatriz Guerra-Roman (Federal Institute for Risk Assessment, Germany) and Dr Dik Mevius (Centraal Veterinair Instituut, the Netherlands) who kindly provided integron-positive controls used in this study.
References
- Altekruse, S.F., Elvinger, F., Lee, K.Y., Tollefson, L.K., Pierson, E.W., Eifert, J. & Sriranganathan N. (2002). Antimicrobial susceptibilities of Escherichia coli strains from a turkey operation. Journal of the American Veterinary Medical Association, 221, 411–416. 10.2460/javma.2002.221.411
- Barnes, H.J., Nolan, L.K. & Vaillancourt, J.P. (2008). Colibacillosis. In Y.M. Saif, A.M. Fadly, J.R. Glisson, L.R. McDougald, L.K. Nolan, & D.E. Swayne (Eds.). Diseases of poultry (12th edn., pp. 691–737). Ames: Iowa State Press.
- Bass, L., Liebert, C.A., Lee, M.D., Summers, A.O., White, D.G., Thayer, S.G. & Maurer, J.J. (1999). Incidence and characterization of integrons, genetic elements mediating multiple-drug resistance, in avian Escherichia coli. Antimicrobial Agents & Chemotherapy, 43, 2925–2929.
- Blanco, J., Blanco, M., Alonso, M.P., Blanco, J.E., Garabal, J.I. & González, E.A. (1992). Serogroups of Escherichia coli strains producing cytotoxic necrotizing factors CNF1 and CNF2. FEMS Microbiology Letters, 96, 155–159. 10.1111/j.1574-6968.1992.tb05409.x
- Bryan, A., Shapir, N. & Sadowsky, M.J. (2004). Frequency and distribution of tetracycline resistance genes in genetically diverse, nonselected, and nonclinical Escherichia coli strains isolated from diverse human and animal sources. Applied & Environmental Microbiology, 70, 2503–2507. 10.1128/AEM.70.4.2503-2507.2004
- Bywater, R. (2005). Results of a European survey of antimicrobial resistance in zoonotic and indicator bacteria from poultry. In Proceedings of the 4th International Bayer Poultry Symposium (pp. 10–16). Istanbul, Bayer Eds., Business Group Animal Health Agricultural Centre.
- CLSI (Clinical and Laboratory Standards Institute). (2008). Performance standards for antimicrobial disk and dilution susceptibility tests for bacteria isolated from animals. Approved standard, 3rd edn. CLSI document M31-A3, vol. 28. Wayne, PA.
- Commission Regulation (EU) No 37/2010 of 22 December 2009 on pharmacologically active substances and their classification regarding maximum residue limits in foodstuffs of animal origin. Official Journal of the European Commission, L15, 1–72.
- Cook, A., Reid-Smith, R., Irwin, R., McEwen, S.A., Valdivieso-Garcia, A. & Ribble, C. (2009). Antimicrobial resistance in Campylobacter, Salmonella, and Escherichia coli isolated from retail turkey meat from southern Ontario, Canada. Journal of Food Protection, 72, 473–481.
- Cormican, M., Buckley, V., Corbett-Feeney G. & Sheridan F. (2001). Antimicrobial resistance in Escherichia coli isolates from turkeys and hens in Ireland. Journal of Antimicrobial Chemotherapy, 48, 587–588. 10.1093/jac/48.4.587
- Dho-Moulin, M. & Fairbrother J.M. (1999). Avian pathogenic Escherichia coli (APEC). Veterinary Research, 30, 299–316.
- Dutil, L. Irwin, R., Finley, R., Ng, L.K., Avery, B., Boerlin, P., Bourgault, A.-M., Cole, L., Daignault, D., Desruisseau, A., Demczuk, W., Hoang, L., Horsman, G.B., Ismail, J., Jamieson, F., Maki, A., Pacagnella, A. & Pillai, D. R. (2010). Ceftiofur resistance in Salmonella enterica serovar Heidelberg from chicken meat and humans, Canada. Emerging Infectious Diseases, 16, 48–54. 10.3201/eid1601.090729
- EFSA (European Food Safety Authority). (2010). The Community Summary Report on antimicrobial resistance in zoonotic and indicator bacteria from animals and food in the European Union in 2004–2007. The EFSA Journal 2010, 8, 1309.
- Ekkapobyotin, C., Padungtod, P. & Chuanchuen, R. (2008). Antimicrobial resistance of Campylobacter coli isolates from swine. International Journal of Food Microbiology, 128, 325–328. 10.1016/j.ijfoodmicro.2008.09.005
- FAO/WHO/OIE. (2008). Joint FAO/OIE/WHO expert meeting on critically important antimicrobials. Report of a meeting held in FAO, Rome, Italy, November 2007, Geneva, Switzerland.
- Fluit, A.C. & Schmitz F.-J. (2004). Resistance integrons and super-integrons. Clinical Microbiology & Infection, 10, 272–288. 10.1111/j.1198-743X.2004.00858.x
- Giraud, E., Leroy-Setrin, S., Flaujac, G., Cloeckaert, A., Dho-Moulin, M. & Chaslus-Dancla, E. (2001). Characterization of high-level fluoroquinolone resistance in Escherichia coli O78:K80 isolated from turkeys. Journal of Antimicrobial Chemotherapy, 47, 341–343. 10.1093/jac/47.3.341
- Gosling, R.J., Clouting, C.S., Randall, L.P., Horton, R.A. & Davies, R.H. (2012). Ciprofloxacin resistance in E. coli isolated from turkeys in Great Britain. Avian Pathology, 41, 83–89. 10.1080/03079457.2011.640659
- Gyles, C.L. (2008). Antimicrobial resistance in selected bacteria from poultry. Animal Health Research Reviews, 9, 149–158. 10.1017/S1466252308001552
- Hall, R.M. (2012). Integrons and gene cassettes: hotspots of diversity in bacterial genomes. Annals of the New York Academy of Sciences, 1267, 71–78. 10.1111/j.1749-6632.2012.06588.x
- Hofacre, C.L., de Cotret, A.R., Maurer, J.J., Garritty, A. & Thayer, S.G. (2000). Presence of fluoroquinolone-resistant coliforms in poultry litter. Avian Diseases, 44, 963–967. 10.2307/1593073
- Johnson, T.J., Giddings, C.W., Horne, S.M., Gibbs, P.S., Wooley, R.E., Skyberg, J., Olah, P., Kercher, R., Sherwood, J.S., Foley, S.L. & Nolan, L.K. (2002). Location of increased serum survival gene and selected virulence traits on a conjugative R plasmid in an avian Escherichia coli isolate. Avian Diseases, 46, 342352. 10.1637/0005-2086(2002)046[0342:LOISSG]2.0.CO;2
- Johnson, J.R., Delavari, P., O'Bryan, T.T., Smith, K.E. & Tatini, S. (2005). Contamination of retail foods, particularly turkey, from community markets (Minnesota, 1999–2000) with antimicrobial-resistant and extraintestinal pathogenic Escherichia coli. Foodborne Pathogens and Disease, 2, 38–49. 10.1089/fpd.2005.2.38
- Kempf, I., Fleury, M.A., Drider, D., Bruneau, M., Sanders, P. Chauvin, C., Madec, J.Y. & Jouy, E. (2013b). What do we know about resistance to colistin in Enterobacteriaceae in avian and pig production in Europe?International Journal of Antimicrobial Agents, 42, 379–383. 10.1016/j.ijantimicag.2013.06.012
- Kempf, I., Le Roux, A., Perrin-Guyomard, A., Mourand, G., Le Devendec, L., Bougeard, S., Richez, P., Le Pottier, G. & Eterradossi, N. (2013a). Effect of in-feed paromomycin supplementation on antimicrobial resistance of enteric bacteria in turkeys. The Veterinary Journal, 198, 398–403.
- Khaitsa, M.L., Oloya, J., Doetkott, D. & Kegode, R. (2008). Antimicrobial resistance and association with class 1 integrons in Escherichia coli isolated from turkey meat products. Journal of Food Protection, 71, 1679–1684.
- Leverstein-van Hall, M.A., Blok, H.E.M., Donders, R.T., Paauw, A., Fluit, A.C. & Verhoef, J. (2003). Multidrug resistance among Enterobacteriaceae is strongly associated with the presence of integrons and is independent of species or isolate origin. The Journal of Infectious Diseases, 187, 251–259. 10.1086/345880
- Lévesque, C., Piché, L., Larose, C. & Roy, P.H. (1995). PCR mapping of integrons reveals several novel combinations of resistance genes. Antimicrobial Agents & Chemotherapy, 39, 185–191. 10.1128/AAC.39.1.185
- Lister, S. (2005). Pathogenic agents involved in turkey respiratory diseases under field conditions, the UK perspective. In Proceedings of the 4th International Bayer Poultry Symposium (pp. 57–73). Istanbul, Bayer Eds., Business Group Animal Health Agricultural Centre.
- Livermore, D.M. (2004). The need for new antibiotics. Clinical Microbiology and Infection, 10, 1–9. 10.1111/j.1465-0691.2004.1004.x
- Mazel, D. (2006). Integrons: agents of bacterial evolution. Nature, 4, 608–620.
- Nógrády, N., Pászti, J., Pikó, H. & Nagy, B. (2006). Class 1 integrons and their conjugal transfer with and without virulence-associated genes in extra-intestinal and intestinal Escherichia coli of poultry. Avian Pathology, 35, 349–356. 10.1080/03079450600827007
- Ozaki, H., Esaki, H., Takemoto, K., Ikeda, A., Nakatani, Y., Someya, A., Hirayama, N. & Murase, T. (2011). Antimicrobial resistance in fecal Escherichia coli isolated from growing chickens on commercial farms. Veterinary Microbiology, 150, 132–139. 10.1016/j.vetmic.2010.12.020
- Partridge, S.R. & Hall, R.M. (2005). Correctly identifying the streptothricin resistance gene cassette. Journal of Clinical Microbiology, 43, 4298–4300. 10.1128/JCM.43.8.4298-4300.2005
- Partridge, S.R., Tsafnat, G., Coiera, E. & Iredell, J.R. (2009). Gene cassettes and cassette arrays in mobile resistance integrons. FEMS Microbiology Reviews, 33, 757–784. 10.1111/j.1574-6976.2009.00175.x
- Piccirillo, A., Dotto, G., Salata, C. & Giacomelli, M. (2013). Absence of class 1 and class 2 integrons among Campylobacter jejuni and Campylobacter coli isolated from poultry in Italy. Journal of Antimicrobial Chemotherapy, 68, 2683–2685. 10.1093/jac/dkt242
- Randall, L.P., Clouting, C., Horton, R.A., Coldham, N.G., Wu, G., Clifton-Hadley, F.A., Davies R.H. & Teale, C.J. (2011). Prevalence of Escherichia coli carrying extended-spectrum β-lactamases (CTX-M and TEM-52) from broiler chickens and turkeys in Great Britain between 2006 and 2009. Journal of Antimicrobial Chemotherapy, 66, 86–95. 10.1093/jac/dkq396
- Randall, L.P., Mueller-Doblies, D., Lemma, F.L., Horton, R.A., Teale, C.J. & Davies, R.H. (2013). Characteristics of ciprofloxacin and cephalosporin resistant Escherichia coli isolated from turkeys in Great Britain. British Poultry Science, 54, 96–105. 10.1080/00071668.2013.763902
- Ribot, E.M., Fair, M.A., Gautom, R., Cameron, D.N., Hunter, S.B., Swaminathan, B. & Barrett, T.J. (2006). Standardization of pulsed-field gel electrophoresis protocols for the subtyping of Escherichia coli O157:H7, Salmonella, and Shigella for PulseNet. Foodborne Pathogens and Disease, 3, 59–67. 10.1089/fpd.2006.3.59
- Salmon, S.A. & Watts, J.L. (2000). Minimum inhibitory concentration determinations for various antimicrobial agents against 1570 bacterial isolates from turkey poults. Avian Diseases, 44, 85–98. 10.2307/1592511
- Schwarz, S., Cloeckaert, A. & Roberts, M.C. (2006). Mechanisms and spread of bacterial resistance to antimicrobial agents. In F.M. Aarestrup (Ed.). Antimicrobial Resistance in Bacteria of Animal Origin (pp. 73–98). Washington, DC: ASM Press.
- Singer, R.S. & Hofacre, C.L. (2006). Potential impacts of antibiotic use in poultry production. Avian Diseases, 50, 161–172. 10.1637/7569-033106R.1
- Singh, R., Schroeder, C.M., Meng, J., White, D.G., McDermott, P.F., Wagner, D.D., Yang, H., Simjee, S., DebRoy, C., Walker, R.D. & Zhao, S. (2005). Identification of antimicrobial resistance and class 1 integrons in Shiga toxin-producing Escherichia coli recovered from humans and food animals. Journal of Antimicrobial Chemotherapy, 56, 216–219. 10.1093/jac/dki161
- Soufi, L., Abbassi, M.S., Sáenz, Y., Vinué, L., Somalo, S., Zarazaga, M., Abbas, A., Dbaya, R., Khanfir, L., Ben Hassen, A., Hammami, S., & Torres, C. (2009). Prevalence and diversity of integrons and associated resistance genes in Escherichia coli isolates from poultry meat in Tunisia. Foodborne Pathogens and Disease, 6, 1067–1073. 10.1089/fpd.2009.0284
- Soufi, L., Sáenz, Y., Vinué, L., Abbassi, M.S., Ruiz, E., Zarazaga, M., Ben Hassen, A., Hammami, S. & Torres, C. (2011). Escherichia coli of poultry food origin as reservoir of sulphonamide resistance genes and integrons. International Journal of Food Microbiology, 144, 497–502. 10.1016/j.ijfoodmicro.2010.11.008
- Taylor, N.M., Davies, R.H., Ridley, A., Clouting, C., Wales A.D. & Clifton-Hadley F.A. (2008). A survey of fluoroquinolone resistance in Escherichia coli and thermophilic Campylobacter spp. on poultry and pig farms in Great Britain. Journal of Applied Microbiology, 105, 1421–1431. 10.1111/j.1365-2672.2008.03877.x
- Tenover, F.C., Arbeit, R.D., Goering, R.V., Mickelsen, P.A., Murray, B.E., Persing, D.H. & Swaminathan, B. (1995). Interpreting chromosomal DNA restriction patterns produced by pulsed-field gel electrophoresis: criteria for bacterial strain typing. Journal of Clinical Microbiology, 33, 2233–2239.
- Van den Bogaard, A.E., London, N., Driessen, C. & Stobberingh E.E. (2001). Antibiotic resistance of faecal Escherichia coli in poultry, poultry farmers and poultry slaughterers. Journal of Antimicrobial Chemotherapy, 48, 587–588. 10.1093/jac/48.4.587
- Van Hoek, A.H.A.M., Mevius, D., Guerra, B., Mullany, P. Roberts, A.P. & Aarts, H.J.M. (2011). Acquired antibiotic resistance genes: an overview. Frontiers in Microbiology, 2, 1–27. 10.3389/fmicb.2011.00203
- White, P. A., McIver, C. J. & Rawlinson, W.D. (2001). Integrons and gene cassettes in the Enterobacteriaceae. Antimicrobial Agents and Chemotherapy, 45, 2658–2661. 10.1128/AAC.45.9.2658-2661.2001
- WHO (World Health Organization). (2012). Critically important antimicrobials for human medicine – 3rd rev. (38 pp). Geneva: WHO.