Abstract
The causative pathogen of necrotic enteritis is the Gram-positive bacterium Clostridium perfringens. Its main cell wall component, peptidoglycan (PGN), can be recognized by Toll-like receptor 2 and nucleotide-binding oligomerization domain (NOD). Consequently, the immune response is initiated via activation of nuclear factor kappa B (NF-κB) signalling pathway. An in vitro study was conducted to investigate chicken intestinal inflammatory responses to C. perfringens type A and one of its virulence factors, α-toxin. In primary intestinal epithelial cells, C. perfringens as well as commercially available PGN and α-toxin challenge upregulated mRNA expression of interleukin (IL)-6, IL-8 and inducible nitric oxide synthase (iNOS) with a dosage-dependent manner at 3 h post infection (p.i.; P ≤ 0.001). Time-course effects of three stimulators at high concentration were further examined. C. perfringens infection elevated IL-6, IL-8 and iNOS levels from 1 h to 9 h p.i., while PGN treatment increased IL-6 and IL-8 expression at 1 h and 3 h p.i. (P < 0.05). Bacterial and PGN treatments induced NOD1 expression at 6 h p.i. and only bacterial infection boosted NF-κB p65 expression at 6 h and 9 h p.i. (P < 0.05). α-Toxin treatment upregulated IL-6 and IL-8 expression throughout infection, as well as iNOS, TNF-α and NF-κB p65 expression at later hours p.i. (P < 0.05). In conclusion, both C. perfringens and α-toxin challenge induced intense cytokine expression associated with NF-κB activation in chicken intestinal epithelial cells. The receptors for the recognition of PGN component of C. perfringens need further investigation.
Introduction
Necrotic enteritis (NE) can be a significant disease in commercially farmed poultry species, especially broiler chickens (McDevitt et al., Citation2006). Clinical NE is characterized by confluent mucosal necrosis of large parts of the small intestine, covered with a yellow–brown or bile-stained pseudomembrane (Broussard et al., Citation1986; Olkowski et al., Citation2006). Typical subclinical cases have ulcers in the form of a depression in the mucosal surface, with discoloured and amorphous material adhering to the mucosal surface (Hofshagen & Kaldhusdal, Citation1992). The clinical form of NE usually leads to high levels of mortality, while the subclinical form of the disease is undetected and causes great economic losses in the poultry production industry (Kaldhusdal & Hofshagen, Citation1992; Dahiya et al., Citation2006).
The causative pathogen of NE is Clostridium perfringens, a Gram-positive anaerobic spore-forming and rod-shaped bacterium (Baba et al., Citation1997). Microscopic examination of early stages of NE shows strong inflammatory reactions to C. perfringens (Timbermont et al., Citation2011). The lamina propria is hyperaemic and infiltrated with numerous inflammatory cells, mainly heterophilic granulocytes. On the other hand, the patterns of cytokine expression in the intestine were changed by C. perfringens infection. Park et al. (Citation2008) reported that gene transcripts encoding interferon (IFN)-α, IFN-γ, interleukin (IL)-1β and IL-10 were significantly upregulated in intestinal intraepithelial lymphocytes from an NE model system in which chickens were co-infected by C. perfringens and Eimeria maxima. Similarly, Collier et al. (Citation2008) observed that chickens with induced NE exhibited increased levels of IFN-γ, IL-4 and IL-10 in the intestine compared with healthy controls.
However, the recognition of C. perfringens and following initiation of innate immune responses in intestinal epithelial cells are poorly understood. As a Gram-positive bacterium, the main cell wall component of C. perfringens is peptidoglycan (PGN). In human and mouse studies, PGN has been reported to activate the innate immune response via two receptors of intestinal epithelial cells, Toll-like receptor (TLR) 2 and nucleotide-binding oligomerization domain (NOD; Wu et al., Citation2007; Müller-Anstett et al., Citation2010; Lin et al., Citation2011). The recognition of PGN by receptors results in activation of downstream signalling pathways, including nuclear factor kappa B (NF-κB) and mitogen-activated protein kinase, to induce cytokine (e.g. IL-6) and chemokine (e.g. IL-8) production (Girardin et al., Citation2003c; Krishnan et al., Citation2007). In avian species, 10 TLRs have been discovered to date and the chicken genome also contains the TLR2 gene (Roach et al., Citation2005). Lu et al. (Citation2009) demonstrated that the members of TLR2 subfamily in chicken spleen and ileum were strongly involved in the host response to C. perfringens infection. However, receptors of NOD1 and NOD2 in the chicken are scarcely reported and only the predicted mRNA sequence of NOD1 is shown on the website of National Center for Biotechnology Information (http://www.ncbi.nlm.nih.gov/nuccore/XM_418777.3).
Other than the inflammation caused by C. perfringens itself, the toxins produced by the bacterium also activate innate immune responses. There are four main toxins (α, β, ε and ι) secreted by five main types of C. perfringens (A, B, C, D and E; Niilo, Citation1980). Type A is responsible for producing avian NE as well as gas gangrene and some forms of human food poisoning (Weiduo et al., Citation2007; Logue et al., Citation2013). The α-toxin is produced by all five types of C. perfringens and has been implicated as one of the virulence factors inducing avian NE for decades (Fukata et al., Citation1988; Zekarias et al., Citation2008; Cooper et al., Citation2009). Although α-toxin negative C. perfringens mutants were observed to be virulent (Keyburn et al., Citation2006), α-toxin was produced in the intestines of broiler chicks inoculated with an α-toxin mutant and there was a direct relationship between intestinal lesion severity and amount of α-toxin detected in the gut contents and mucosa (R = 0.89–0.99; Coursodon et al., Citation2010). On the other hand, C. perfringens α-toxin has been shown to be a major virulence factor required for the development of gas gangrene with inflammatory myopathies (Sakurai et al., Citation2004). In human gas gangrene, α-toxin caused large aggregates of adherent platelets, which led to the impairment of neutrophil mobility to infected tissues (Bryant et al., Citation2006). The α-toxin was also reported to induce the production of intercellular mediators in human umbilical vein endothelial cells, intercellular adhesion molecule 1, IL-8, tumour necrosis factor (TNF)-α, platelet-activating factor and the endothelial leukocyte adhesion molecule (Bryant & Stevens, Citation1996). However, little is known about the inflammatory responses to α-toxin in chicken intestinal epithelial cells.
The purpose of this study was to investigate the inflammatory responses in primary chicken intestinal epithelial cells challenged with C. perfringens type A and α-toxin. The relative expression analysis of immune gene transcripts was conducted by quantitative real-time (qRT)-PCR. It was hypothesized that C. perfringens infection activated innate immune responses through TLR2 or NOD recognition, and that α-toxin exposure induced an inflammatory response in chicken intestinal epithelial cells.
Materials and Methods
Primary culture of intestinal epithelial cells
All experimental procedures used were approved by the China Agricultural University Animal Care and Use Committee. The cell culture medium and buffers were purchased from Invitrogen-Gibco (Carlsbad, CA, USA). Cell culture plastics were obtained from Corning Life Science (Tewksbury, MA, USA). Epidermal growth factor was purchased from BD Biosciences (San Jose, CA, USA). All other chemicals, unless specified, were obtained from Sigma-Aldrich (St. Louis, MO, USA).
Primary intestinal epithelial cells were prepared from 17-day-old specific pathogen free chicken embryos, which were bred by Ross 308 broiler breeders (China Agricultural University, Beijing, China). The protocol for isolating cells was based on the isolation of intestinal epithelial cells from mice, which was developed by Athman et al. (Citation2005), with some modifications. Briefly, specific pathogen free chicken embryos were dissected, and small intestines were separated and cut into small pieces in Hank's Buffered Salt Solution prior to being digested with 1 mg/mL collagenase type I (in Hank's Buffered Salt Solution pH 7.4) for 50 min at 37°C with gentle shaking (80 cycles/min). The cells were then collected by centrifugation at 800 × g for 8 min at room temperature. The cells were washed twice by a 1:1 mixture of Dulbecco's modified Eagles medium (DMEM) and Ham's F12 medium and resuspended in DMEM/F12 medium supplemented with 100 U/mL of penicillin, 100 μg/mL of streptomycin, 20 ng/mL of epidermal growth factor, 100 μg/mL of heparin sodium salt, 5 μg/mL of insulin and 2.5% foetal bovine serum. Undigested intestinal tissues were removed by filtering the suspension with a sterile 200-mesh sieve. Fibroblasts and macrophages were discarded by 2 h adherence to a tissue culture flask at 37°C with 5% CO2 (Velge et al., Citation2002). Non-adherent cells were transferred to tissue culture plates for further use. Following 48 h of incubation, the medium was changed with fresh medium. Immunostaining for one of epithelial markers, cytokeratin 18, was performed to validate that obtained cells were intestinal epithelial cells (data not shown).
C. perfringens, PGN and α-toxin preparation
The chicken C. perfringens type A field strain was isolated from a clinical case of NE and obtained from the China Veterinary Culture Collection Center (Beijing, China). This organism does not carry the netB gene, which was determined using PCR (data not shown). The bacteria were cultured anaerobically on tryptose-sulphite-cycloserine for 18 h at 37°C and then aseptically inoculated into cooked meat medium and incubated anaerobically overnight at 37°C. The bacteria culture was then centrifuged at 3000 × g for 10 min, washed twice by sterile phosphate buffered saline (PBS, pH 7.4) and resuspended in DMEM/F12 medium for infection. Bacterial concentration was estimated by measuring the absorbance at 600 nm and by relating the optical density to colony forming units (data not shown).
Commercially available PGN (from Staphylococcus aureus) and α-toxin (phospholipase C from C. perfringens, Type I) were obtained from Sigma-Aldrich. They were also dissolved in DMEM/F12 for stimulating the cells and their concentrations used were based on previous research (Chiu et al., Citation2009; Lin et al., Citation2011; Sumners et al., Citation2012).
Quantification of relative cell viability
After isolation from small intestines as described, cells were seeded in 96-well cell culture plates at a concentration of 1.0 × 105 cells/well with a total volume of 100 μL. Cells were incubated at 37°C with 5% CO2 for 48 h. Medium was removed from each well and cells were gently washed with 100 μL pre-warmed PBS (pH 7.4). One hundred microlitres of medium containing C. perfringens [multiplicity of infection (MOI) at 1, 10 and 100], PGN (10, 30 and 50 μg/mL) or α-toxin (10, 100 and 1000 U/L) were added to wells (n = 10 wells). DMEM/F12 medium alone was used as control (n = 10 wells) and wells without seeding cells served as blanks (n = 10 wells). The incubation lasted for 3 h at 37°C with 5% CO2. Then cell medium was discarded and cells were gently washed once with 100 μL pre-warmed PBS (pH 7.4). Each well then received 100 μL of DMEM/F12 medium supplemented with 10 μL WST-8 (Dojindo Molecular Technologies, Gaithersburg, MD, USA), 100 U/mL of penicillin and 100 μg/mL of streptomycin. WST-8 can be reduced by dehydrogenase in cells to give an orange-coloured product (formazan), whose amount is proportional to the number of living cells. The plates were incubated for another 2 h at 37°C with 5% CO2. Following this incubation, formazan was detected at optical density of 450 nm with a microplate spectrophotometer (BioTek Instruments, Inc., Vinooski, VT, USA). The relative cell viability was calculated as % of control using the following formula: [(test absorbance – blank)/(control – blank)] × 100.
Cytokine responses
The isolated cells were seeded in six-well cell culture plates at a density of 2.0 × 105 cells/mL (2 mL per well). At 90% confluence, cells were gently washed three times with pre-warmed PBS (pH 7.4) and then were stimulated with C. perfringens at MOI of 1:1, 10:1 and 100:1; PGN at 10, 30 and 50 μg/mL; α-toxin at 10, 100 and 1000 U/L, respectively. The DMEM/F12 medium alone served as the control. Experiments were run in triplicate. Following 3 h of stimulation, the cells were collected for RNA extraction.
Time-course effects of bacterial and α-toxin infection
Based on the dosage experiments described above, the cells in six-well plates were stimulated with MOI of 100 bacteria. Controls consisted of mock infections using DMEM/F12 medium alone and positive control of PGN (50 μg/mL). On the other hand, cells were either stimulated with 1000 U/L α-toxin or maintained as unstimulated controls to determine the time-dependent immune responses to α-toxin stimulation. All experiments were run in triplicates for each time point (1 h, 3 h, 6 h and 9 h). Following incubation, the cells were collected for RNA isolation.
Gene expression analysis
The RNA extraction was performed using Qiagen RNeasy Mini Kit (Valencia, CA, USA) following the cell spin method and using QIAshredder columns (Qiagen). The concentration and purity of total RNA was estimated by measuring its optical density at 260 and 280 nm. One microgram of total RNA was reverse transcribed by a reverse transcription kit (Invitrogen Life Technologies, Carlsbad, CA, USA) according to the manufacturer's instructions. All of the cDNA preparations were stored frozen at –20°C until further use. The qRT-PCR assay was performed with the 7500 fluorescence detection system (Applied Biosystems, Foster City, CA, USA) according to optimized PCR protocols using the SYBR-Green PCR kit (Invitrogen Life Technologies). The primer pairs for the amplification of chicken IL-6, IL-8, TNF-α, inducible nitric oxide synthase (iNOS), TLR2.2, NOD1, NF-κB p65 and β-actin cDNA fragments, used as an endogenous reference gene, were used as listed in . To confirm amplification specificity, the PCR products from each primer pair were subjected to a melting analysis and subsequent agarose gel electrophoresis. Samples were run in triplicate in 96-well plates. Relative gene expression data were analysed using the 2–ΔΔCt method (Heid et al., Citation1996) and the average ΔCt values of control (DMEM/F12 medium alone) served as the calibrator within each time point.
Table 1. Primers used for qRT-PCR.
Statistical analysis
All the data were analysed with SPSS Version 17.0 (SPSS Inc., Chicago, IL, USA). For time-course effects of α-toxin treatment, an independent-samples t-test was used between stimulated and unstimulated groups. The other data were checked for normality with Shapiro–Wilk test and subjected to homogeneity test of variance. If both tests were positive, data were analysed by one-way analysis of variance and Tukey test was used for multiple comparisons between treatments. If not, data were analysed by Kruskal–Wallis test, which is a non-parametric test. Furthermore, linear and quadratic effects for cell viability and cytokine gene expression in response to different dosages of stimulators were performed by using orthogonal polynomial contrasts. Significance was accepted at P < 0.05 and results were reported as means and standard error (SE).
Results
Relative cell viability
Data of relative cell viability to control are shown in . Compared with the control, C. perfringens challenge at MOI of 100 significantly decreased the cell viability (P < 0.05; ). The α-toxin treatment at 100 and 1000 U/L reduced relative cell viability in contrast to the control (P < 0.05; ). The relative cell viability in the α-toxin treatment at the highest concentration was also lower than that in the treatment of 10 U/L and the relative cell viability was linearly decreased with increasing concentrations of C. perfringens or α-toxin (P < 0.001). However, PGN at various dosages did not significantly change relative cell viability ().
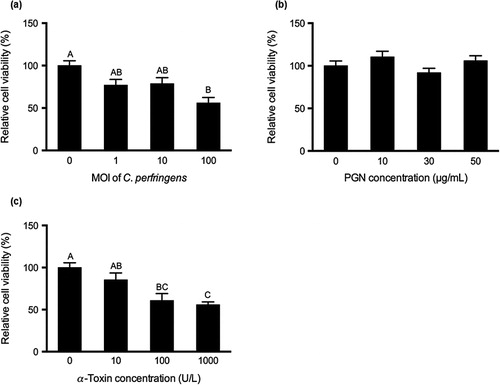
Cytokine responses
Relative mRNA expression of IL-6, IL-8, iNOS and TNF-α was measured to evaluate the cytokine responses in primary chicken intestinal epithelial cells. As shown in , C. perfringens challenge at the MOI of 100 significantly increased IL-6, IL-8 and iNOS mRNA expression compared with the treatment of control and the other two bacterial dosages (P < 0.05). At the MOI of 10, C. perfringens upregulated IL-6 and IL-8 mRNA expression compared to the control (P < 0.05). The gene expression of cytokines in cells challenged with C. perfringens at MOI of 1 was not significantly changed. The PGN treatment at concentrations of 30 and 50 μg/mL promoted IL-6, IL-8 and iNOS mRNA expression in contrast to the control (P < 0.05; ). No significant difference was observed in TNF-α mRNA expression during C. perfringens or PGN stimulation at various dosages. The cells infected with 1000 U/L α-toxin had the highest IL-6, IL-8, iNOS and TNF-α mRNA expression among toxin treatments (P < 0.05; ). The α-toxin stimulation at 100 U/L did not significantly affect the cytokine expression compared with the control. The α-toxin infection at 10 U/L had higher IL-6 expression than that in the control (P < 0.05). Generally, IL-6, IL-8 and iNOS mRNA expression in cells was linearly upregulated by the increasing concentrations of C. perfringens, PGN and α-toxin (P ≤ 0.001). The similar changes of TNF-α expression were only seen in the treatments with α-toxin (P = 0.001).
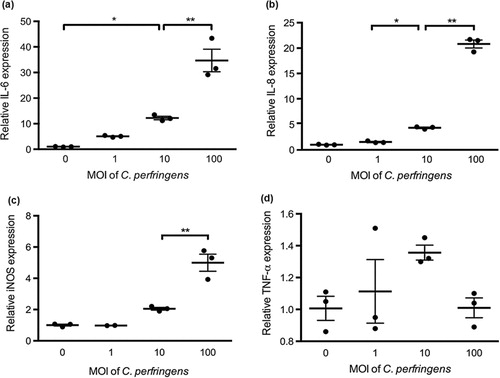
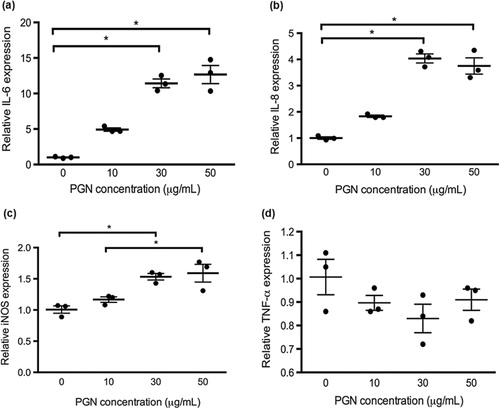
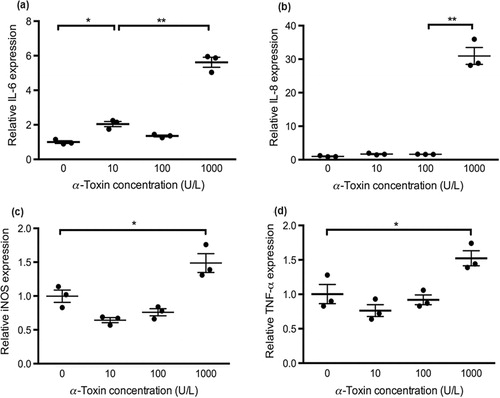
Time-course effects of C. perfringens challenge on cytokine responses
The cells were challenged with C. perfringens at the MOI of 100 for 1, 3, 6 and 9 hours. Cell medium alone was set as the control while PGN treatment at 50 μg/mL served as a positive control. The mRNA expression of cytokines (IL-6, IL-8, iNOS and TNF-α) was quantified and data are shown in . Both bacterial and PGN stimulations upregulated IL-6 and IL-8 mRNA expression at 1 h and 3 h post infection (p.i.; P < 0.05) and IL-6 mRNA expression in C. perfringens group was lower than that in PGN group at 1 h p.i. (P < 0.05). At 6 h and 9 h p.i., C. perfringens infection increased IL-6 and IL-8 mRNA expression compared with control and PGN treatment (P < 0.05). The iNOS mRNA expression in cells with bacterial stimulation was the highest among treatments at various hours p.i. (P < 0.05). The PGN treatment upregulated TNF-α mRNA expression in contrast to control and bacterial group at 6 h and 9 h p.i. (P < 0.05) but C. perfringens stimulation did not significantly affect TNF-α mRNA expression and even downregulated it at 9 h p.i. compared with the control.
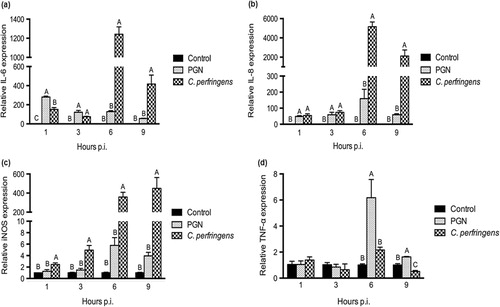
Expression of receptors and NF-κb p65 affected by C. perfringens infection
The mRNA expression of receptors, TLR2.2 and NOD1, were measured to examine the recognition of C. perfringens in chicken intestinal epithelial cells. The expression of NF-κB p65, which plays a key role in induction of inflammation, was also quantified. Data are shown in . The bacterial infection and PGN treatment exerted no significant influence on the mRNA expression of TLR2.2 in contrast to the control (). The changes of NOD1 expression were only seen at 6 h p.i., at which time point the NOD1 level in the bacterial group was intermediate between PGN treatment and the control (P < 0.05; ). Soon after infection (1 h p.i.), PGN treatment downregulated mRNA expression of NF-κB p65 compared with the control (P < 0.05; ). With longer time of infection (6 h and 9 h p.i.), C. perfringens infection boosted higher NF-κB p65 mRNA expression than that of the control and PGN treatments (P < 0.05).
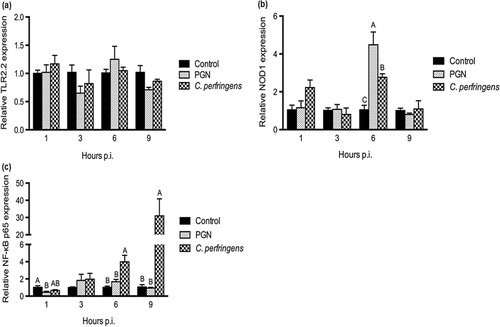
Time-course effects of α-toxin stimulation on inflammatory responses
The α-toxin stimulation at 1000 U/L caused significant changes of inflammatory gene expression in chicken intestinal epithelial cells (). The IL-6 and IL-8 mRNA expression in the toxin-stimulated group was much higher than that in the control at the various hours p.i. (P < 0.05; and ). The levels of iNOS and TNF-α were also increased by toxin stimulation at 3 h, 6 h and 9 h p.i. (P < 0.05; and ). α-Toxin treatment significantly upregulated NF-κB p65 expression at 6 h and 9 h p.i. (P < 0.05) and tended to induce its expression at 3 h p.i. (P = 0.082; ).
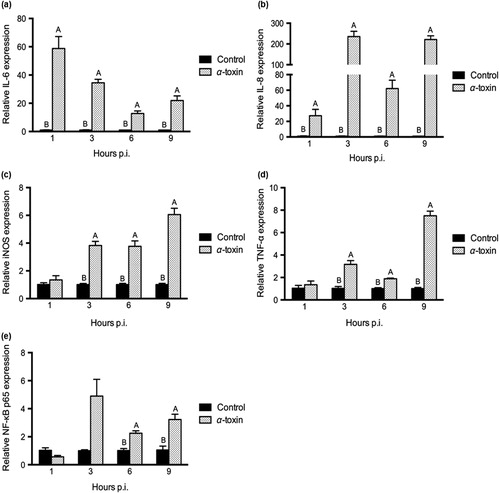
Discussion
The mucosa of the small intestine plays a key role in host defence and immune responses. The pattern-recognition receptors of intestinal epithelial cells can recognize various pathogen-associated molecular patterns and danger-associated molecular patterns. This will trigger the inflammatory response to defence against micro-organism invasion (Akira et al., Citation2006). In this in vitro study, primary chicken intestinal epithelial cells were challenged with C. perfringens type A and α-toxin to investigate the molecular mechanisms of innate immune responses evoked by the pathogen and its toxin.
The relative cell viability was linearly decreased by exposing cells to increasing concentrations of C. perfringens and α-toxin, which indicated the toxic effects of the bacterium and its toxin on intestinal epithelial cells. It was reported that C. perfringens challenge resulted in a significant increase in apoptotic index in small intestine of chickens and this might be due to the local inflammatory response caused by bacterial infection (Liu et al., Citation2012). Sumners et al. (Citation2012) obtained the similar negative effects of C. perfringens α-toxin on peripheral blood mononuclear cells from two different chicken lines. They observed that cell proliferation was inhibited due to exposure to α-toxin at 100 and 1000 U/L, and cell cytotoxicity was increased with increasing toxin concentrations at 2 h and 4 h p.i. The α-toxin is a zinc-metalloenzyme with phospholipase C and sphingomyelinase activities (Songer, Citation1997). The toxin at small concentrations could activate endogenous phospholipase C and/or sphingomyelinase while at high concentrations it induced massive degradation of phosphatidylcholine and sphingomyelin in membranes, following by membrane disruption (Sakurai et al., Citation2004). We found PGN treatment did not alter relative cell viability. The effects of PGN treatment on viability of intestinal cells were scarcely reported in the literature.
The mRNA expression of iNOS and three pro-inflammatory cytokines, IL-6, IL-8 and TNF-α were analysed by qRT-PCR. IL-6 is a multifunctional cytokine and predominantly mediates the acute-phase response, an innate immune mechanism that is triggered by infection and inflammation (Akira et al., Citation1990). IL-8, a CXC chemokine produced by macrophages, functions primarily as a chemoattractant and therefore plays an important role in inflammation (Baggiolini & Clark-Lewis, Citation1992; Shahzad et al., Citation2010). iNOS is mainly produced by macrophages, and catalyzes the production of NO, which has a critical role in the immune system by acting as a cytotoxic and tumoricidal agent (Lin et al., Citation1996). TNF-α in mammals is a member of a group of NF-κB-activated signalling cytokines that stimulate systemic inflammation (Lu et al., Citation2009). The current work showed that the mRNA expression of IL-6, IL-8 and iNOS were linearly upregulated in a dose-response manner by C. perfringens, PGN and α-toxin treatments.
In the bacterial stimulation experiment, the expression of IL-6 and IL-8 was promptly (at 1 h p.i.) upregulated by C. perfringens and PGN stimulation. As incubation time extended to 6 h and 9 h, bacterial infection led to extremely high expression of IL-6 and IL-8. In an in vivo study, jejunual IL-8 mRNA expression was increased in a co-infection model of E. maxima and C. perfringens induced NE (Lee et al., Citation2013). Park et al. (Citation2008) reported that co-infection induced upregulated intestinal IL-8 mRNA expression compared with control and single infections at day 1 post challenge, but IL-6 expression was not significantly affected, and C. perfringens infection alone had no effect on intestinal iNOS gene expression. This was different from our observation that iNOS expression in the bacterial group was much higher than control and PGN treatment at any time point p.i. Lu et al. (Citation2009) reported that ileal IL-8 mRNA expression in C. perfringens-challenged chickens was upregulated from day 1 to 4 p.i. while expression of IL-6 was failed to be detected. In contrary to the finding by Lu et al. (Citation2009) that TNF-α expression in the ileum was increased on day 1 and day 2 p.i., the present work showed that C. perfringens infection did not induce the expression of TNF-α in chicken intestinal epithelial cells and even decreased it at 9 h p.i. Although C. perfringens infection induced inflammatory responses in both in vitro studies of chicken intestinal cells and in vivo studies of chicken intestines, the expression patterns of pro-inflammatory cytokines were not absolutely consistent in these two kinds of studies, which might be due to the more complex environment of the intestine compared with cultured cells. Little is known about the immune responses to PGN treatment in the chicken intestine. However, in the rat model of Crohn's disease, the gene expression of IL-1β, IL-6 and TNF-α in cecal tissue were significantly upregulated by PGN-polysaccharide (Rahal et al., Citation2012). PGNs isolated from different commensal bacteria induced the mRNA expression of pro-inflammatory cytokines, IL-12p35, IL-8 and TNF-α, in Caco-2 cells through activation of the TLR2 pathway (Zenhoma et al., Citation2012). Consistently, in the current study, the PGN derived from S. aureus also augmented IL-6 and IL-8 mRNA expression at early stage (1 h and 3 h p.i.) and TNF-α mRNA expression at later stage (6 h and 9 h p.i.) of exposure.
In the chicken, TLR2 underwent gene duplication early in avian evolution to give TLR2.1 and TLR2.2, both of which could recognize PGN of Gram-positive bacteria (Brownlie & Allan, Citation2011). In the present study, mRNA expression of TLR2.1 was too low to be detected and C. perfringens challenge as well as PGN treatment did not significantly affect TLR2.2 expression. Lu et al. (Citation2009) obtained the similar finding that both TLR2.1 and TLR2.2 mRNA expression in ileal tissues was not significantly changed due to C. perfringens infection. However, the expression of adaptor molecules MyD88 (myeloid differentiation primary response gene 88), TRAF6 (TNF receptor-associated factor 6), TRIF (TIR domain-containing adapter-inducing IFN-β) and receptor interacting protein 1 in the ileum was increased post infection and positively correlated to the expression of TLR2.2 and TLR1.1. This implied that TLR2.2 signalling pathway participated in ileal response to C. perfringens infection.
In mammals and insects, NOD1 and NOD2 mediated the intracellular recognition of PGN and were also involved in innate immune defence responses through pathways that are independent of TLR (Chaput & Boneca, Citation2007; Royet & Dziarski, Citation2007). NOD1, detecting D-glutamyl-meso-diaminopimelic which is present in a PGN that is primarily found not only in Gram-negative bacteria but also in certain groups of Gram-positive bacteria such as Bacillus subtilis and Listeria monocytogenes (Chamaillard et al., Citation2003; Girardin et al., Citation2003a), is an upstream activator of NF-κB (Bertin et al., Citation1999; Inohara & Nuñez, Citation2003). NOD2, detecting muramyl dipeptide that is ubiquitously present in bacterial PGN (Girardin et al., Citation2003b; Inohara et al., Citation2003), is a susceptibility gene involved in the aetiology of Crohn's disease (Hugot et al., Citation2001; Ogura et al., Citation2001). As nucleotide sequence of NOD2 in chicken has not been disclosed yet, we focused on the changes of NOD1 expression here. In the current study, upregulation of NOD1 expression was seen in PGN and C. perfringens treatments at 6 h p.i. Hasegawa et al. (Citation2011) reported that Clostridium difficile, a Gram-positive anaerobic bacterium as C. perfringens, could be directly recognized by NOD1 in nonimmune cells such as mouse primary mesothelial cells, causing recruitment of neutrophils to the infected site to clear the enteric pathogen.
NF-κB signalling in intestinal epithelial cells is important for the maintenance of immune homeostasis in the gut (Pasparakis, Citation2012). Both commensal and pathogenic bacteria express microbial-associated molecular patterns, which are recognized by pattern-recognition receptors (e.g. TLRs and NOD-like receptors) present on the surface of intestinal epithelial cells. Triggering of the receptors induces the nuclear translocation of NF-κB after IκB phosphorylation and disassociation. In the nucleus, NF-κB transcription factors regulate expression of numerous genes implicated in inflammatory response, immune response, control of apoptosis and multiple other cellular processes. In the current study, NF-κB p65 expression was upregulated by C. perfringens infection at 6 h and 9 h p.i., which suggested that the increased expression of pro-inflammatory cytokines upregulated by C. perfringens was associated with the activation of NF-κB signalling pathway. However, whether TLR2.2 or NOD1 are involved in the recognition of PGN located in cell wall of C. perfringens, leading to the initiation of the NF-κB pathway, needs further investigation.
The role of α-toxin in the pathogenesis of gas gangrene has been well studied, including increasing capillary permeability, inducing platelet aggregation, haemolysis, myonecrosis and decreasing cardiac contractility (Flores-Díaz & Alape-Girón, Citation2003). This lethal effect of α-toxin was closely related to the release of TNF-α into the bloodstream, which was supported by the observation that the injection of mice with 20 ng of α-toxin induced TNF-α production in the serum at 1 and 3 h post injection, and that TNF-α-deficient mice were resistant to α-toxin (Oda et al., Citation2008). Oda et al. (Citation2012) further found that α-toxin induced production of IL-8 through the activation of two separate pathways, the extracellular regulated kinase 1/2/NF-κB and p38 mitogen-activated protein kinase pathways in A549 cells, a human lung adenocarcinoma epithelial cell line. The released IL-8 activated migration and binding of neutrophils, leading to the massive accumulation of neutrophils at the vascular endothelium around the margins of the necrotic region, but not within the infected area of gas gangrene. In the current work, the toxin at a concentration of 1000 U/L upregulated mRNA expression of IL-6, IL-8, iNOS and TNF-α at 1 h or 3 h p.i., and this effect continued until 9 h p.i. These pro-inflammatory cytokines might play an important role in the process of inflammation caused by α-toxin in the chicken intestine. In chicken peripheral blood mononuclear cells, exposure to C. perfringens α-toxin at 1000 U/L increased mRNA expression of IL-8 and iNOS at 2 h p.i (Sumners et al., Citation2012). Consistent with the finding of Oda et al. (Citation2012), we observed that the upregulation of cytokine expression was associated with NF-κB p65 expression. However, Tumurkhuu et al. (Citation2009) reported that pretreatment with wild type α-toxin longer than 6 h inhibited lipopolysaccharide-induced TNF-α and nitric oxide in RAW 264.7 cells via reduced p65 NF-κB subunit activation. They explained that bacterial sphingomyelinase activity of α-toxin led to the generation of higher amount of intracellular ceramide, which had an inhibitory action on protein kinase C activity that inhibited NF-κB activation via protein kinase B. More work needs to be done on the mechanism mediating immune responses induced by α-toxin.
In conclusion, C. perfringens type A and α-toxin challenge induced intense innate immune responses associated with NF-κB activation in primary chicken intestinal epithelial cells, and the receptors that played a key role in recognizing PGN component of C. perfringens need further investigation. Research on the molecular mechanism of immune responses to C. perfringens and α-toxin infection in chicken intestine is useful to prevent or decrease the incidence of NE.
Additional information
Funding
References
- Akira, S., Hirano, T., Taga, T. & Kishimoto, T. (1990). Biology of multifunctional cytokines: IL 6 and related molecules (IL 1 and TNF). The Journal of the Federation of American Societies for Experimental Biology, 4, 2860–2867.
- Akira, S., Uematsu, S. & Takeuchi, O. (2006). Pathogen recognition and innate immunity. Cell, 124, 783–801. 10.1016/j.cell.2006.02.015
- Athman, R., Fernandez, M.-I., Gounon, P., Sansonetti, P., Louvard, D., Philpott, D. & Robine, S. (2005). Shigella flexneri infection is dependent on villin in the mouse intestine and in primary cultures of intestinal epithelial cells. Cellular Microbiology, 7, 1109–1116. 10.1111/j.1462-5822.2005.00535.x
- Baba, E., Ikemoto, T., Fukata, T., Sasai, K., Arakawa, A. & McDougald, L.R. (1997). Clostridial population and the intestinal lesions in chickens infected with Clostridium perfringens and Eimeria necatrix. Veterinary Microbiology, 54, 301–308. 10.1016/S0378-1135(96)01289-8
- Baggiolini, M. & Clark-Lewis, I. (1992). Interleukin-8, a chemotactic and inflammatory cytokine. FEBS Letters, 307, 97–101. 10.1016/0014-5793(92)80909-Z
- Bertin, J., Nir, W-J., Fischer, C.M., Tayber, O.V., Errada, P.R., Grant, J.R., Keilty, J.J., Gosselin, M.L., Robison, K.E., Wong, G.H.W., Glucksmann, M.A. & DiStefano, P.S. (1999). Human CARD4 protein is a novel CED-4/Apaf-1 cell death family member that activates NF-B. The Journal of Biological Chemistry, 274, 12955–12958. 10.1074/jbc.274.19.12955
- Broussard, C.T., Hofacre, C.L., Page, R.K. & Fletcher, O.J. (1986). Necrotic enteritis in cage-reared commercial layer pullets. Avian Diseases, 30, 617–619. 10.2307/1590433
- Brownlie, R. & Allan, B. (2011). Avian toll-like receptors. Cell and Tissue Research, 343, 121–130. 10.1007/s00441-010-1026-0
- Bryant, A.E. & Stevens, D.L. (1996). Phospholipase C and perfringolysin O from Clostridium perfringens upregulate endothelial cell-leukocyte adherence molecule 1 and intercellular leukocyte adherence molecule 1 expression and induce interleukin-8 synthesis in cultured human umbilical vein endothelial cells. Infection and Immunity, 64, 358–362.
- Bryant, A.E., Bayer, C.R., Aldape, M.J., Wallace, R.J., Titball, R.W. & Stevens, D.L. (2006). Clostridium perfringens phospholipase C-induced platelet/leukocyte interactions impede neutrophil diapedesis. Journal of Medical Microbiology, 55, 495–504. 10.1099/jmm.0.46390-0
- Chamaillard, M., Hashimoto, M., Horie, Y., Masumoto, J., Qiu, S., Saab, L., Ogura, Y., Kawasaki, A., Fukase, K., Kusumoto, S., Valvano, M.A., Foster, S.J., Mak, T.W., Nuñez, G. & Inohara, N. (2003). An essential role for NOD1 in host recognition of bacterial peptidoglycan containing diaminopimelic acid. Nature Immunology, 4, 702–707. 10.1038/ni945
- Chaput, C. & Boneca, I.G. (2007). Peptidoglycan detection by mammals and flies. Microbes and Infection, 9, 637–647. 10.1016/j.micinf.2007.01.022
- Chiu, Y.-C., Lin, C.-Y., Chen, C.-P., Huang, K.-C., Tong, K.-M., Tzeng, C.-Y., Lee, T.-S., Hsu, H.-C. & Tang, C.-H. (2009). Peptidoglycan enhances IL-6 production in human synovial fibroblasts via TLR2 receptor, focal adhesion kinase, Akt, and AP-1-dependent pathway. The Journal of Immunology, 183, 2785–2792. 10.4049/jimmunol.0802826
- Collier, C.T., Hofacre, C.L., Payne, A.M., Anderson, D.B., Kaiser, P., Mackie, R.I. & Gaskins, H.R. (2008). Coccidia-induced mucogenesis promotes the onset of necrotic enteritis by supporting Clostridium perfringens growth. Veterinary Immunology and Immunopathology, 122, 104–115. 10.1016/j.vetimm.2007.10.014
- Cooper, K.K., Trinh, H.T. & Songer, J.G. (2009). Immunization with recombinant alpha toxin partially protects broiler chicks against experimental challenge with Clostridium perfringens. Veterinary Microbiology, 133, 92–97. 10.1016/j.vetmic.2008.06.001
- Coursodon, C.F., Trinh, H.T., Mallozzi, M., Vedantam, G., Glock, R.D. & Songer, J.G. (2010). Clostridium perfringens alpha toxin is produced in the intestines of broiler chicks inoculated with an alpha toxin mutant. Anaerobe, 16, 614–617. 10.1016/j.anaerobe.2010.09.006
- Dahiya, J.P., Wilkie, D.C., Van Kessel, A.G. & Drew, M.D. (2006). Potential strategies for controlling necrotic enteritis in broiler chickens in post-antibiotic era. Animal Feed Science and Technology, 129, 60–88. 10.1016/j.anifeedsci.2005.12.003
- Flores-Díaz, M. & Alape-Girón, A. (2003). Role of Clostridium perfringens phospholipase C in the pathogenesis of gas gangrene. Toxicon, 42, 979–986.
- Fukata, T., Hadate, Y., Baba, E., Uemura, T. & Arakawa, A. (1988). Influence of Clostridium perfringens and its toxin in germ-free chickens. Research in Veterinary Science, 44, 68–70.
- Girardin, S.E., Boneca, I.G., Carneiro, L.A.M., Antignac, A., Jéhanno, M., Viala, J., Tedin, K., Taha, M-K., Labigne, A., Zähringer, U., Coyle, A.J., DiStefano, P.S., Bertin, J., Sansonetti, P.J. & Philpott, D.J. (2003a). Nod1 detects a unique muropeptide from Gram-negative bacterial peptidoglycan. Science, 300, 1584–1587. 10.1126/science.1084677
- Girardin, S.E., Boneca, I.G., Viala, J., Chamaillard, M., Labigne, A., Thomas, G., Philpott, D.J. & Sansonetti, P.J. (2003b). Nod2 is a general sensor of peptidoglycan through muramyl dipeptide (MDP) detection. The Journal of Biological Chemistry, 278, 8869–8872. 10.1074/jbc.C200651200
- Girardin, S.E., Travassos, L.H., Herve, M., Blanot, D., Boneca, I.G., Philpott, D.J., Sansonetti, P.J. & Mengin-Lecreulx, D. (2003c). Peptidoglycan molecular requirements allowing detection by Nod1 and Nod2. The Journal of Biological Chemistry, 278, 41702–41708.
- Hasegawa, M., Yamazaki, T., Kamada, N., Tawaratsumida, K., Kim, Y-G., Nuñez, G. & Inohara, N. (2011). Nucleotide-binding oligomerization domain 1 mediates recognition of Clostridium difficile and induces neutrophil recruitment and protection against the pathogen. The Journal of Immunology, 186, 4872–4880. 10.4049/jimmunol.1003761
- Heid, C.A., Stevens, J., Livak, K.J. & Williams, P.M. (1996). Real time quantitative PCR. Genome Research, 6, 986–994. 10.1101/gr.6.10.986
- Hofshagen, M. & Kaldhusdal, M. (1992). Barley inclusion and avoparcin supplementation in broiler diets. 1. Effect on small intestinal bacterial flora and performance. Poultry Science, 71, 959–969. 10.3382/ps.0710959
- Hugot, J-P., Chamaillard, M., Zouali, H., Lesage, S., Cézard, J-P., Belaiche, J., Almer, S., Tysk, C., O'Morain, C.A., Gassull, M., Binder, V., Finkel, Y., Cortot, A., Modigliani, R., Laurent-Puig, P., Gower-Rousseau, C., Macry, J., Colombel, J-F., Sahbatou, M. & Thomas, G. (2001). Association of NOD2 leucine-rich repeat variants with susceptibility to Crohn's disease. Nature, 411, 599–603. 10.1038/35079107
- Inohara, N. & Nuñez, G. (2003). NODs: intracellular proteins involved in inflammation and apoptosis. Nature Reviews Immunology, 3, 371–382.
- Inohara, N., Ogura, Y., Fontalba, A., Gutierrez, O., Pons, F., Crespo, J., Fukase, K., Inamura, S., Kusumoto, S., Hashimoto, M., Foster, S.J., Moran, A.P., Fernandez-Luna, J.L. & Nuñez, G. (2003). Host recognition of bacterial muramyl dipeptide mediated through NOD2. The Journal of Biological Chemistry, 278, 5509–5512. 10.1074/jbc.C200673200
- Jiang, Y., Kong, Q., Roland, K.L. & Curtiss III, R. (2014). Membrane vesicles of Clostridium perfringens type A strains induce innate and adaptive immunity. International Journal of Medical Microbiology, 304, 431–443. 10.1016/j.ijmm.2014.02.006
- Kaldhusdal, M. & Hofshagen, M. (1992). Barley inclusion and avoparcin supplementation in broiler diets. 2. Clinical, pathological, and bacteriological findings in a mild form of necrotic enteritis. Poultry Science, 71, 1145–1153. 10.3382/ps.0711145
- Keyburn, A.L., Sheedy, S.A., Ford, M.E., Williamson, M.M., Awad, M.M., Rood, J.I. & Moore, R.J. (2006). Alpha-toxin of Clostridium perfringens is not an essential virulence factor in necrotic enteritis in chickens. Infection and Immunity, 74, 6496–6500. 10.1128/IAI.00806-06
- Krishnan, J., Selvarajoo, K., Tsuchiya, M., Lee, G. & Choi, S. (2007). Toll-like receptor signal transduction. Experimental and Molecular Medicine, 39, 421–438. 10.1038/emm.2007.47
- Lee, S.H., Lillehoj, H.S., Jang, S.I., Lillehoj, E.P., Min, W. & Bravo, D.M. (2013). Dietary supplementation of young broiler chickens with Capsicum and turmeric oleoresins increases resistance to necrotic enteritis. British Journal of Nutrition, 110, 840–847. 10.1017/S0007114512006083
- Lin, A.W., Chang, C.C. & McCormick, C.C. (1996). Molecular cloning and expression of an avian macrophage nitric-oxide synthase cDNA and the analysis of the genomic 5′-flanking region. The Journal of Biological Chemistry, 271, 11911–11919. 10.1074/jbc.271.20.11911
- Lin, H.Y., Tang, C.H., Chen, J.H., Chuang, J.Y., Huang, S.M., Tan, T.W., Lai, C.H. & Lu, D.Y. (2011). Peptidoglycan induces interleukin-6 expression through the TLR2 receptor, JNK, c-Jun, and AP-1 pathways in microglia. Journal of Cellular Physiology, 226, 1573–1582. 10.1002/jcp.22489
- Liu, D., Guo, S. & Guo, Y. (2012). Xylanase supplementation to a wheat-based diet alleviated the intestinal mucosal barrier impairment of broiler chickens challenged by Clostridium perfringens. Avian Pathology, 41, 291–298. 10.1080/03079457.2012.684089
- Logue, C.M., Songer, J.G., Uzal, F., Opengart, K., Skarin, H., Blomqvist, G. & Båverud, V. (2013). Clostridial diseases. In D.E. Swayne, J.R. Glisson, L.R. McDougald, L.K. Nolan, D.L. Suarez, & V.L. Nair (2013). Diseases of Poultry 13th edn (pp. 943–970). Ames: Iowa State Press.
- Lu, Y., Sarson, A.J., Gong, J., Zhou, H.J., Zhu, W.Y., Kang, Z.M., Yu, H., Sharif, S. & Han, Y.M. (2009). Expression profiles of genes in Toll-like receptor-mediated signaling of broilers infected with Clostridium perfringens. Clinical and Vaccine Immunology, 16, 1639–1647. 10.1128/CVI.00254-09
- McDevitt, R.M., Brooker, J.D., Acamovic, T. & Sparks, N.H.C. (2006). Necrotic enteritis; a continuing challenge for the poultry industry. World's Poultry Science Journal, 62, 221–247.
- Müller-Anstett, M.A., Muller, P., Albrecht, T., Nega, M., Wagener, J., Gao, Q., Kaesler, S., Schaller, M., Biedermann, T. & Götz, F. (2010). Staphylococcal peptidoglycan co-localizes with Nod2 and TLR2 and activates innate immune response via both receptors in primary murine keratinocytes. PLoS One, 5, e13153.
- Niilo, L. (1980). Clostridium perfringens in animal disease: a review of current knowledge. The Canadian Veterinary Journal, 21, 141–148.
- Oda, M., Kihara, A., Yoshioka, H., Saito, Y., Watanabe, N., Uoo, K., Higashihara, M., Nagahama, M., Koide, N., Yokochi, T. & Sakurai, J. (2008). Effect of erythromycin on biological activities induced by Clostridium perfringens α-toxin. The Journal of Pharmacology and Experimental Therapeutics, 327, 934–940. 10.1124/jpet.108.143677
- Oda, M., Shiihara, R., Ohmae, Y., Kabura, M., Takagishi, T., Kobayashi, K., Nagahama, M., Inoue, M., Abe, T., Setsu, K. & Sakurai, J. (2012). Clostridium perfringens alpha-toxin induces the release of IL-8 through a dual pathway via TrkA in A549 cells. Biochimica et Biophysica Acta, 1822, 1581–1589. 10.1016/j.bbadis.2012.06.007
- Ogura, Y., Bonen, D.K., Inohara, N., Nicolae, D.L., Chen, F.F., Ramos, R., Britton, H., Moran, T., Karaliuskas, R., Duerrk, R.H., Achkar, J.-P., Brant, S.R., Bayless, T.M., Kirschners, B.S., Hanauer, S.B., Nuñez, G. & Cho, J.H. (2001). A frameshift mutation in NOD2 associated with susceptibility to Crohn's disease. Nature, 411, 603–606. 10.1038/35079114
- Olkowski, A.A., Wojnarowicz, C., Chirino-Trejo, M. & Drew, M.D. (2006). Responses of broiler chickens orally challenged with Clostridium perfringens isolated from field cases of necrotic enteritis. Research in Veterinary Science, 81, 99–108. 10.1016/j.rvsc.2005.10.006
- Park, S.S., Lillehoj, H.S., Allen, P.C., Park, D.W., FitzCoy, S., Bautista, D.A. & Lillehoj, E.P. (2008). Immunopathology and cytokine responses in broiler chickens coinfected with Eimeria maxima and Clostridium perfringens with the use of an animal model of necrotic enteritis. Avian Diseases, 52, 14–22. 10.1637/7997-041707-Reg
- Pasparakis, M. (2012). Role of NF-κB in epithelial biology. Immunological Reviews, 246, 346–358. 10.1111/j.1600-065X.2012.01109.x
- Rahal, K., Schmiedlin-Ren, P., Adler, J., Dhanani, M., Sultani, V., Rittershaus, A.C., Reingold, L., Zhu, J., McKenna, B.J., Christman, G.M. & Zimmermann, E.M. (2012). Resveratrol has antiinflammatory and antifibrotic effects in the peptidoglycan-polysaccharide rat model of Crohn's disease. Inflammatory Bowel Diseases, 18, 613–623. 10.1002/ibd.21843
- Roach, J.C., Glusman, G., Rowen, L., Kaur, A., Purcell, M.K., Smith, K.D., Hood, L.E. & Aderem, A. (2005). The evolution of vertebrate Toll-like receptors. Proceedings of the National Academy of Sciences, 102, 9577–9582. 10.1073/pnas.0502272102
- Royet, J. & Dziarski, R. (2007). Peptidoglycan recognition proteins: pleiotropic sensors and effectors of antimicrobial defences. Nature Reviews Microbiology, 5, 264–277. 10.1038/nrmicro1620
- Sakurai, J., Nagahama, M. & Oda, M. (2004). Clostridium perfringens alpha-toxin: characterization and mode of action. Journal of Biochemistry, 136, 569–574. 10.1093/jb/mvh161
- Shahzad, A., Knapp, M., Lang, I. & Köhler, G. (2010). Interleukin 8 (IL-8) – a universal biomarker? International Archives of Medicine, 3, 11–14. 10.1186/1755-7682-3-11
- Songer, J.G. (1997). Bacterial phospholipases and their role in virulence. Trends in Microbiology, 5, 156–161. 10.1016/S0966-842X(97)01005-6
- Sumners, L.H., Cox, C.M., Kim, S., Salevsky, J.E., Siegel, P.B. & Dalloul, R.A. (2012). Immunological responses to Clostridium perfringens alpha-toxin in two genetically divergent lines of chickens as influenced by major histocompatibility complex genotype. Poultry Science, 91, 592–603. 10.3382/ps.2011-01775
- Timbermont, L., Haesebrouck, F., Ducatelle, R. & Van Immerseel, F. (2011). Necrotic enteritis in broilers: an updated review on the pathogenesis. Avian Pathology, 40, 341–347. 10.1080/03079457.2011.590967
- Tumurkhuu, G., Koide, N., Dagvadorj, J., Noman, A.S.M., Iftekar-E-Khuda, I., Naiki, Y., Komatsu, T., Yoshida, T., Oda, M., Nagahama, M., Sakurai, J. & Yokochi, T. (2009). The inhibition of lipopolysaccharide-induced tumor necrosis factor-α and nitric oxide production by Clostridium perfringens α-toxin and its relation to alpha-toxin-induced intracellular ceramide generation. International Journal of Medical Microbiology, 299, 554–562. 10.1016/j.ijmm.2009.04.003
- Velge, P., Bottreau, E., Quéré, P., Pardon, P., Nicolle, J.C., Morisson, M., Bout, D. & Dimier, I. (2002). Establishment and characterization of partially differentiated chicken enterocyte cell clones. European Journal of Cell Biology, 81, 203–212. 10.1078/0171-9335-00237
- Weiduo, S., Gong, J., Han, Y., Yu, H., Brennan, J., Zhou, H. & Chen, S. (2007). Quantification of cell proliferation and alpha-toxin gene expression of Clostridium perfringens in the development of necrotic enteritis in broiler chickens. Applied and Environmental Microbiology, 73, 7110–7113. 10.1128/AEM.01108-07
- Wu, L., Feng, B.S., He, S.H., Zheng, P.Y., Croitoru, K. & Yang, P.C. (2007). Bacterial peptidoglycan breaks down intestinal tolerance via mast cell activation: the role of TLR2 and NOD2. Immunology and Cell Biology, 85, 538–545. 10.1038/sj.icb.7100079
- Zekarias, B., Mo, H. & Curtiss III, R. (2008). Recombinatant attenuated Salmonella enterica serovar Typhimurium expressiong the carboxy-terminal domain of α-toxin from Clostridium perfringens induces protective responses against necrotic enteritis in chickens. Clinical and Vaccine Immunology, 15, 805–816. 10.1128/CVI.00457-07
- Zenhoma, M., Hydera, A., Vresea, M.D., Hellera, K.J., Roederb, T. & Schrezenmeir, J. (2012). Peptidoglycan recognition protein 3 (PglyRP3) has an anti-inflammatory role in intestinal epithelial cells. Immunobiology, 217, 412–419. 10.1016/j.imbio.2011.10.013