Abstract
Riemerella anatipestifer is a major bacterial pathogen of waterfowl, globally responsible for avian septicaemic disease. As chemotherapy is the predominant method for the prevention and treatment of R. anatipestifer infection in poultry, the widespread use of antibiotics has favoured the emergence of antibiotic-resistant strains. However, little is known about R. anatipestifer susceptibility to macrolide antibiotics and its resistance mechanism. We report for the first time the identification of a macrolide resistance mechanism in R. anatipestifer that is mediated by the ribosomal RNA methyltransferase ermF. We identified the presence of the ermF gene in 64/206 (31%) R. anatipestifer isolates from different regions in China. An ermF deletion strain was constructed to investigate the function of the ermF gene on the resistance to high levels of macrolides. The ermF mutant strain showed significantly decreased resistance to macrolide and lincosamide, exhibiting 1024-, 1024-, 4- and >2048-fold reduction in the minimum inhibitory concentrations for erythromycin, azithromycin, tylosin and lincomycin, respectively. Furthermore, functional analysis of ermF expression in E. coli XL1-blue showed that the R. anatipestifer ermF gene was functional in E. coli XL1-blue and conferred resistance to high levels of erythromycin (100 µg/ml), supporting the hypothesis that the ermF gene is associated with high-level macrolide resistance. Our work suggests that ribosomal RNA modification mediated by the ermF methyltransferase is the predominant mechanism of resistance to erythromycin in R. anatipestifer isolates.
Introduction
Riemerella anatipestifer is the aetiological agent of serositis and septicaemia in domestic ducks, geese, turkeys and several other domestic and wild birds (Ruiz & Sandhu, Citation2013). These bacteria cause the anatipestifer syndrome in ducks, which is characterized by fibrinous pericarditis, perihepatitis, caseous salpingitis and meningitis and diarrhoea (Ruiz & Sandhu, Citation2013). R. anatipestifer infection results in serious morbidity and mortality as high as 75% (Gerlach, Citation1970) and leads to enormous global economic losses in the duck industry (Ruiz & Sandhu, Citation2013). To date, at least 21 serotypes of R. anatipestifer have been isolated (Sandhu & Harry, Citation1981; Loh et al., Citation1992; Pathanasophon et al., Citation1995; Pathanasophon et al., Citation2002), with serotypes 1, 2 and 10 having been responsible for most of the major outbreaks in China (Anchun et al., Citation2003). Variable levels of virulence have been reported among R. anatipestifer serotypes and even within a given serotype (Subramaniam et al., Citation2000). Unfortunately, subunit vaccines (Subramaniam et al., Citation2000) and vaccines based on a single serotype of inactivated bacteria or live or cell-free culture filtrates (Pathanasophon et al., Citation1996; Huang et al., Citation2002) have not provided significant cross-protection among R. anatipestifer serotypes (Liu et al., Citation2013).
Chemotherapy is very important in the prevention and treatment of R. anatipestifer infection in poultry, and the antimicrobial susceptibility of R. anatipestifer has been reported (Chang et al., Citation2003; Zhong et al., Citation2009). However, the widespread overuse of antibiotics has accelerated the emergence and prevalence of antibiotic resistance in R. anatipestifer strains in China (Hvistendahl, Citation2012). R. anatipestifer has a very wide drug resistance spectrum, showing resistance to most antibiotics. Several drug resistance genes have been identified in R. anatipestifer (Sun et al., Citation2012), including a chloramphenicol resistance gene (cat; Chen et al., Citation2010), a chloramphenicol and florfenicol resistance gene (floR; Chen et al., Citation2012), aminoglycoside resistance genes (ant, aac and aph; Yang et al., Citation2012) and tetracycline resistance gene (tet; Zhong et al., Citation2013).
Macrolide, lincosamide and streptogramin B (collectively referred to as MLS) antibiotics have a similar mode of action, regardless of their different chemical structure. MLS antibiotics inhibit protein synthesis by binding to the 50S ribosomal subunit (Poehlsgaard & Douthwaite, Citation2005). Bacterial resistance to macrolide antibiotics is mainly mediated by one of three mechanisms: target site modification by gene mutation or methylation (Weisblum, Citation1995), efflux pumps (Poole, Citation2007) and gene inactivation. In most bacteria, MLS resistance can be attributed to post-transcriptional modification of the 23S rRNA mediated by a ribosomal RNA methyltransferase encoded by one of the genes of the erm family (Weisblum, Citation1995). Various erm genes have been identified in a wide range of host bacteria, such as members of Bacteroides and Streptococcus consisting of transposon-borne ermB, ermF and ermA genes and the plasmid-borne ermC gene. Methyltransferase proteins (Erm) are classified according to whether they add one (monomethyltransferases) or two (dimethyltransferases) methyl groups to a specific 23S rRNA nucleotide position. Dimethyltransferases Erm generally confer high-level resistance to MLS antibiotics, while monomethyltransferases mediate high-level resistance to lincosamides, but low- or medium-level resistance to macrolides and streptogramin B (Weisblum, Citation1995; Takaya et al., Citation2013). To date, none of the molecular mechanisms involved in resistance and susceptibility to macrolide antibiotics reported in bacteria has been identified in R. anatipestifer.
The genome of several R. anatipestifer strains has been sequenced (Mavromatis et al., Citation2011; Yuan et al., Citation2011; Zhou et al., Citation2011; Cheng et al., Citation2012; Wang et al., Citation2012). With the goal of understanding the genetic basis of MLS resistance in R. anatipestifer, we analysed the genome of R. anatipestifer strain CH-1 and identified a gene with high nucleotide and deduced amino acid sequence homology to ermF genes from bovine streptococcal species and Bacteroides fragilis (Chung et al., Citation1999a). This gene encodes a 23S rRNA adenine N-6-methyltransferase and is predicted to play a role in R. anatipestifer sensitivity to macrolide antibiotics. To test this hypothesis, we generated an ermF gene deletion mutant in R. anatipestifer strain CH-1 (CH-1ΔermF) by allelic exchange and expressed the R. anatipestifer ermF gene in Escherichia coli XL1-blue.
Materials and Methods
Bacterial strains, plasmids and culture conditions
Bacterial strains, plasmids and their relevant characteristics are listed in . R. anatipestifer strains were cultured on tryptic soy agar (TSA; Oxoid, Basingstoke, UK) or in tryptic soy broth (TSB; Oxoid) at 37°C in 5% CO2 atmosphere. E. coli strains were grown on Luria-Bertani (LB; Oxoid) agar plates or broth at 37°C. When necessary, the medium was supplemented with the appropriate antibiotics at the following concentrations: ampicillin (100 µg/ml, Sigma-Aldrich, St Louis, MO, USA), chloramphenicol (25 µg/ml, Sigma), erythromycin (100 µg/ml, Sigma) or spectinomycin (40 µg/ml, Sigma). Diaminopimelic acid (50 µg/ml; Sigma) was added when growing E. coli X7213 (Edwards et al., Citation1998).
Table 1. Strains, plasmids and primers used in this study.
Detection of the ermF gene in R. anatipestifer isolates
Two hundred and six R. anatipestifer isolates were collected from different regions of China among which 75 isolates were from Sichuan province, 27 from Jiangshu, 24 from Guizhou, 18 from Guangdong, 15 from Chongqing, 12 from Guangxi, 12 from Hainan, 10 from Jiling, 7 from Henan and 6 from Beijing. All isolates were identified using the Biolog Microbial Identification System (Biolog, Hayward, CA, USA), polymerase chain reaction (PCR) and their biochemical characteristics. The presence of the ermF gene in these strains was analysed by PCR after lysing bacteria in lysis buffer (0.5% NP-40, Sigma; 200 ng/ml proteinase K, Takara Biotechnology Co., Ltd. Dalian, China) using primers Erm F and Erm R ().
Construction of R. anatipestifer ermF mutant strain CH-1ΔermF
An ermF mutant strain was generated using the R. anatipestifer strain CH-1. The ermF gene was deleted by allelic exchange using recombinant suicide vector pYA4278 (Kong et al., Citation2011; donated by Professor Kong), which replaced the entire ermF gene with an 1145-bp SpecR cassette, following the methods of Choi & Schweizer (Citation2005) and Kong et al. (Citation2011). Briefly, fragments approximately 620 bp long were amplified by PCR from genomic regions upstream (L) and downstream (R) the R. anatipestifer CH-1 ermF gene using primers Erm-Left F and Erm-Left R, and Erm-Right F and Erm-Right R, respectively. A 1145-bp SpecR cassette (S) was PCR-amplified from plasmid pYES1 new using primers Spc F and Spc R. The three fragments were then spliced together in vitro by overlap extension using primers Erm-Left F and Erm-Right R, producing the LSR fragment. Adenosine nucleotides were added to both ends of the PCR product, which was then ligated to AhdI-digested T-cloning suicide vector pYA4278 to generate pYA4278-LSR (SpecR), which carries a deletion of the entire ermF gene. Subsequently, pYA4278-LSR was transformed into E. coli X7232 (Edwards et al., Citation1998), and then further transformed into E. coli X7213λpir. E. coli X7213λpir transformed with pYA4278-LSR, the donor strain, and R. anatipestifer CH-1 (KanamycinR, SpecS), the recipient strain, were grown in LB or TSB, respectively, at 37°C with shaking to optical density at 600 nm (OD600) = 0.6–0.7. Donor and recipient strains were mixed in a 10-mM MgSO4 solution and filtered through a 0.22-µm Millipore membrane filter. The filter was then incubated on TSB agar with diaminopimelic acid at 37°C for 24 h. SpecR transconjugants were further selected in media containing spectinomycin (40 µg/ml). Transconjugants’ identity was further confirmed by PCR (see and its legend for additional details). Primers and strains are listed in .
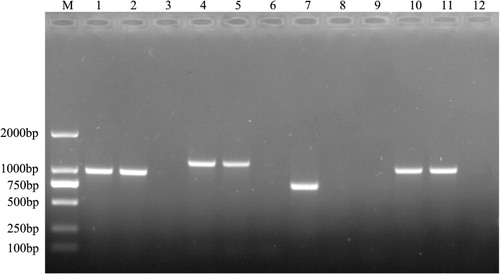
Determination of R. anatipestifer CH-1ΔermF growth rate
Wild-type R. anatipestifer CH-1 and mutant CH-1ΔermF were grown in TSB at 37°C with shaking. The growth rate was analysed for each strain by inoculating 1 ml from an overnight broth culture into 100-ml fresh TSB and further growing at 37°C for 20 h with shaking, as described by Penuelas-Urquides et al. (Citation2013). OD600 was recorded at 1-h intervals using a spectrophotometer, and growth curves were plotted in , as previously described (Hu et al., Citation2002).
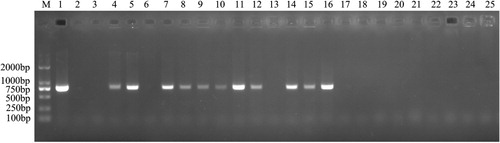
Testing of R. anatipestifer CH-1δermf antimicrobial susceptibility in vitro
R. anatipestifer antibiotic susceptibility was assessed by disc-diffusion analysis on TSA plates supplemented with sheep blood (5%, v/v) (Zhong et al., Citation2009). R. anatipestifer CH-1 and CH-1ΔermF strains were grown overnight in TSB medium, re-inoculated in the same medium (25 ml) and allowed to grow to OD600=0.4–0.6 (exponential growing phase). Bacteria were coated on TSA blood agar plates, and plates were solidified for 10–15 min at room temperature. After solidification of the medium, 10-mm sterile discs saturated with erythromycin (15 µg), clarithromycin (15 µg), azithromycin (15 µg), spiramycin (100 µg), clindamycin (2 µg), ampicillin (10 µg), ceftriaxone (30 µg), chloromycetin (30 µg), florfenicol (30 µg), gentamicin (10 µg), sulphamethoxazole (25 µg) or enrofloxacin (5 µg) were spotted on the plate, 3 cm apart from each other. Plates were incubated for 18–24 h at 37°C in a candle jar (approximately 5% CO2) and zones of inhibition were measured for each antibiotic. Staphylococcus aureus [American Type Culture Collection (ATCC) 25923] was used as quality-control strain. All sensitivity-testing experiments were performed in triplicate and repeated at least twice.
MLS sensitivity was compared between R. anatipestifer CH-1 and CH-1ΔermF by calculating the minimum inhibitory concentration for erythromycin, azithromycin, tylosin, lincomycin, tetracycline and florfenicol using the standard microbroth dilution method, according to Clinical and Laboratory Standard Institute criteria (CLSI, Citation2014). S.aureus was used as a quality-control strain. MICs for each antibiotic were determined twice.
Expression of the ermF gene in E. coli XL1-blue
To test whether the R. anatipestifer ermF gene was responsible for high-level macrolide resistance, R. anatipestifer CH-1 ermF coding region was cloned into E. coli XL1-blue (Stratagene). Briefly, the ermF gene was amplified from R. anatipestifer CH-1 genomic DNA using primers Erm-N-EcoRI and Erm-C-KpnI. The amplified PCR product was digested with EcoRI and KpnI and cloned into the pBAD24 EcoRI/KpnI site, under control of the arabinose pBAD promoter (Guzman et al., Citation1995). The resulting expression construct (pBAD24-ermF) was transformed into E. coli XL1-blue. E. coli transformants were selected on LB agar plates containing erythromycin (100 g/ml) and arabinose (0.4%). Insertion of the ermF gene was confirmed by colony PCR. One millilitre E. coli XL1-blue (pBAD24-ermF) from overnight-grown colonies was transferred into 100-ml fresh LB medium (1%, v/v), and ermF expression was induced by addition of arabinose to a final concentration of 0.4%. Growth curves were plotted for E. coli XL1-blue harbouring the pBAD24 empty vector or pBAD24-ermF grown in LB medium with or without erythromycin (100 µg/ml) at 37°C. OD600 was recorded at 2-h intervals.
We deduced the amino acid sequence for the R. anatipestifer ermF gene and used the BLASTP program to search the NCBI protein database (Altschul et al., Citation1990).
Results
Characterization of R. anatipestifer CH-1 ermF gene
The genomes of R. anatipestifer serotype 1 strain RA-CH-1 has been sequenced in our laboratory (GenBank Accession No.CP003787) (Wang et al., Citation2014). The genome sequence of R. anatipestifer CH-1, which is one of the most prevalent serotypes in China, is significantly different from the genomes of other R. anatipestifer strains (Wang et al., Citation2014). In R. anatipestifer CH-1, we identified gene B739-0033 (located at position 27429–28229 nt. on the genome of R. anatipestifer CH-1), which we propose is an ermF gene. Among all genomes of R. anatipestifer strains deposited in the NCBI, the ermF gene was only identified in wild-type CH-1 strain. The ermF gene is 801-bp long and shows 99% nucleotide identity to various ermF genes present in different bacterial plasmid and transposons, such as Bacteroides fragilis plasmid pBF4 (GenBank Accession No. M14730; Rasmussen et al., Citation1986), Capnocytophaga sputigena strain Be58 (GenBank Accession No. JQ707297; Ehrmann et al., Citation2014) and Tn4551 (GenBank Accession No. M17808; Smith, Citation1987). The results of BLASTP showed that the ermF gene protein product contains a highly conserved protein domain present in the S-adenosylmethionine-dependent methyltransferases superfamily (Martin & McMillan, Citation2002). The prototype of these enzymes, 23S rRNA adenine N-6-methyltransferase, is responsible for bacterial high-level resistance to macrolide antibiotics (Roberts et al., Citation1999). So we are interested if the R. anatipestifer ermF gene has this function.
Identification of the ermF gene in R. anatipestifer isolates
The ermF gene sequence was successfully PCR-amplified in 64/206 (31%) R. anatipestifer isolates collected from different regions of China, suggesting that the ermF gene may be widely distributed among MLS-resistant R. anatipestifer strains. PCR analysis of a subset of R. anatipestifer isolates is shown in .
Construction and characterization of R. anatipestifer ermF mutant strain CH-1ΔermF
The R. anatipestifer ermF mutant strain CH-1ΔermF was constructed by allelic exchange (see Materials and Methods for details). R. anatipestifer CH-1 and mutant CH-1ΔermF exhibited similar colony morphologies. Both wild-type and ermF mutant strains formed similar convex, entire, transparent and butyrous, non-pigmented colonies on TSA plates. The growth curves for R. anatipestifer CH-1 and mutant CH-1ΔermF strains were similar ().
Sensitivity of R. anatipestifer CH-1ΔermF to MLS anitibiotics
Our in silico analysis suggested that the R. anatipestifer CH-1ΔermF mutant might be sensitive to peptidyl-transferase centre-binding antibiotics. Therefore, we compared the sensitivity of R. anatipestifer CH-1 and mutant CH-1ΔermF to several antibiotics. As predicted, CH-1ΔermF showed altered sensitivity only to MLS antibiotics, exhibiting MICs that were 1024-, 1024-, 4- and >2048-fold lower for erythromycin, azithromycin, tylosin and lincomycin, respectively, than the corresponding MICs for its parent strain (). These results suggest that the R. anatipestifer CH-1 ermF gene conferred resistance to MLS antibiotics.
Table 2. Antimicrobial susceptibility of R. anatipestifer CH-1ΔermF and parent strain.
In vivo functional analysis of R. anatipestifer ermF
We confirmed the function of the R. anatipestifer CH-1 ermF gene in vivo by testing susceptibility to erythromycin of E. coli strains expressing the R. anatipestifer ermF gene. While growth of E. coli XL1-blue (harbouring the empty pBAD24 vector) was almost completely inhibited in LB broth supplemented with a high concentration of erythromycin (100 µg/ml), ermF-expressing E. coli grew significantly better in the same media conditions (). These results indicate that the R. anatipestifer CH-1 ermF gene is functional in E. coli XL1-blue strain and confers high-level resistance to MLS antibiotics.
Discussion
Methylation of 23S rRNA by the methyltransferase encoded by erythromycin ribosome methylation (erm) gene is the most prevalent mechanism of resistance to macrolide antibiotics in bacteria (Arthur et al., Citation1987; Chung et al., Citation1999a). Methylation of the 23S rRNA in the peptidyl-transfer centre reduces the affinity of the 50S ribosomal subunit for MLS antibiotics, conferring the MLS phenotype (Leclercq & Courvalin, Citation1991; Chung et al., Citation1999b). Loss of 23S rRNA methylation could increase sensitivity to MLS antibiotics due to a reduction in hydrophobicity or an increase in the affinity for these antibiotics. To date, approximately 40 different classes of erm genes have been described in a variety of species (http://faculty.washington.edu/marilynr/; Vester & Long, Citation2009). Some strains carry multiple erm genes, such as ermA, B, C, F and Q, with ermF being the most common.
A mechanism of macrolide resistance has not been described in R. anatipestifer. In this study, we describe the identification of the 23SrRNA methyltransferase ermF gene and propose that it mediates resistance to MLS antibiotics in R. anatipestifer. The R. anatipestifer gene B739-0033 is unique to strain CH-1, and its deduced amino acid sequence shares high similarity with the 23S rRNA adenine N-6-methyltransferase from other bacteria. Importantly, we also detected the presence of the ermF gene in 64/206 R. anatipestifer isolates from different regions of China.
To provide experimental evidence that this putative ermF gene confers macrolide antibiotic resistance, we deleted the ermF gene from R. anatipestifer CH-1 by allelic exchange. The R. anatipestifer deletion mutant was significantly more sensitive to all MLS antibiotics than its parent strain R. anatipestifer CH-1, which showed a constitutive MLS resistance phenotype. Further work to study potential hydrophobicity changes due to the lack of 23S rRNA methylation in the ermF mutant should be completed.
In order to further confirm a functional role of the R. anatipestifer ermF gene in macrolide antibiotic resistance, we expressed the ermF gene in E. coli XL1-blue strain. E. coli strains expressing the ermF gene could resist high levels of erythromycin. This result indicated that ermF can be successfully expressed in E. coli and is involved in MLS antibiotic resistance, supporting our hypothesis that the ermF gene is a macrolide antibiotic resistance determinant factor in R. anatipestifer.
It is noteworthy that even though macrolide antibiotics are rarely used for treating infections of R. anatipestifer, the methyltransferase gene ermF widely exists in R. anatipestifer. Interestingly, many ermF genes are located in conjugative or non-conjugative transposons and plasmids, with only few having been found on chromosomes (Roberts et al., Citation1999). The ermF gene (GenBank Accession No. M14730) is found on Bacteroides transposons Tn4351 and Tn4000, and on Bacteroides fragilis plasmid pBF4. It is often associated with other antibiotic resistance genes, for example, the tetQ gene (Salyers et al., Citation1995). These conjugative transposons often have a wide host range, suggesting that their dissemination potentially occurred via horizontal gene transfer (Chung et al., Citation1999a, b), which may explain why many different bacteria carry various erm genes (Qin et al., Citation2014). However, in R. anatipestifer CH-1, the ermF gene is chromosomally located. Where or how the ermF gene was acquired by R. anatipestifer CH-1 needs further study.
In conclusion, this study describes, for the first time, the identification of the R. anatipestifer ermF gene, which is suggested to be responsible for R. anatipestifer resistance to macrolide antibiotics. MLS resistance in R. anatipestifer was determined to be constitutive and chromosomally mediated. Gene deletion studies and in vivo expression analysis support our hypothesis that the ermF gene encodes a 23S rRNA methyltransferase conferring resistance to MLS antibiotics. To our knowledge, this is the first report that resistance to macrolide antibiotics in R. anatipestifer is attributed solely to the expression of the ermF gene.
Additional information
Funding
References
- Altschul, S.F., Gish, W., Miller, W., Myers, E.W. & Lipman, D.J. (1990). Basic local alignment search tool. Journal of Molecular Biology, 215, 403–410.10.1016/S0022-2836(05)80360-2
- Anchun, C., Mingshu, W., DeKang, Z., Cheng, H., Fei, L., Yi, Z., Yufei, G., Zhaoyu, L. & Pengfei, F. (2003). Epidemiology and new serotypes of Riemerella anatipestifer isolated from ducks in China and studies on their pathogenic characteristics. Chinese Journal of Veterinary Science, 23, 320–323.
- Arthur, M., Brisson-Noel, A. & Courvalin, P. (1987). Origin and evolution of genes specifying resistance to macrolide, lincosamide and streptogramin antibiotics: data and hypotheses. Journal of Antimicrobial Chemotherapy, 20, 783–802.10.1093/jac/20.6.783
- Chang, C.F., Lin, W.H., Yeh, T.M., Chiang, T.S. & Chang, Y.F. (2003). Antimicrobial susceptibility of Riemerella anatipestifer isolated from ducks and the efficacy of ceftiofur treatment. Journal of Veterinary Diagnostic Investigation, 15, 26–29.10.1177/104063870301500106
- Chen, Y.P., Tsao, M.Y., Lee, S.H., Chou, C.H. & Tsai, H.J. (2010). Prevalence and molecular characterization of chloramphenicol resistance in Riemerella anatipestifer isolated from ducks and geese in Taiwan. Avian Pathology, 39, 333–338.10.1080/03079457.2010.507761
- Chen, Y.P., Lee, S.H., Chou, C.H. & Tsai, H.J. (2012a). Detection of florfenicol resistance genes in Riemerella anatipestifer isolated from ducks and geese. Veterinary Microbiology, 154, 325–331.10.1016/j.vetmic.2011.07.012
- Cheng, L.F., Chen, H.M., Zheng, T., Fu, G.H., Shi, S.H., Wan, C.H. & Huang, Y. (2012b). Complete genomic sequence of the virulent bacteriophage RAP44 of Riemerella anatipestifer. Avian Diseases, 56, 321–327.10.1637/9770-050411-Reg.1
- Choi, K.H. & Schweizer, H.P. (2005). An improved method for rapid generation of unmarked Pseudomonas aeruginosa deletion mutants. BMC Microbiology, 5, 30.10.1186/1471-2180-5-30
- Chung, W.O., Werckenthin, C., Schwarz, S. & Roberts, M.C. (1999a). Host range of the ermF rRNA methylase gene in bacteria of human and animal origin. Journal of Antimicrobial Chemotherapy, 43, 5–14. 10.1093/jac/43.1.5
- Chung, W.O., Young, K., Leng, Z. & Roberts, M.C. (1999b). Mobile elements carrying ermF and tetQ genes in Gram-positive and Gram-negative bacteria. Journal of Antimicrobial Chemotherapy, 44, 329–335. 10.1093/jac/44.3.329
- Clinical and Laboratory Standards Institute (CLSI) (2014). Performance Standards for Antimicrobial Susceptibility Testing; Twenty-fourth Informational Supplement M100-S24. Wayne, PA: Clinical and Laboratory Standards Institute.
- Edwards, R.A., Keller, L.H. & Schifferli, D.M. (1998). Improved allelic exchange vectors and their use to analyze 987P fimbria gene expression. Gene, 207, 149–157.10.1016/S0378-1119(97)00619-7
- Ehrmann, E., Handal, T., Tamanai-Shacoori, Z., Bonnaure-Mallet, M. & Fosse, T. (2014). High prevalence of beta-lactam and macrolide resistance genes in human oral Capnocytophaga species. Journal of Antimicrobial Chemotherapy, 69, 381–384.10.1093/jac/dkt350
- Gerlach, H. (1970). Pasteurella anatipestifer infection in ducklings. Deutsche Tierärztliche Wochenschrift, 77, 541–542.
- Guzman, L.M., Belin, D., Carson, M.J. & Beckwith, J. (1995). Tight regulation, modulation, and high-level expression by vectors containing the arabinose PBAD promoter. Journal of Bacteriology, 177, 4121–4130.
- Halula, M.C., Manning, S. & Macrina, F.L. (1991). Nucleotide sequence of ermFU, a macrolide-lincosamide-streptogramin (MLS) resistance gene encoding an RNA methylase from the conjugal element of Bacteroides fragilis V503. Nucleic Acids Research, 19, 3453.10.1093/nar/19.12.3453
- Hu, Q., Chen, H., Liu, X., Zhan, M., Zhang, Z., Deen, S. & Zhang, Y. (2002). Determination of growth curve of Riemerella anatipestifer. Animal Husbandry & Veterinary Medicine, 34, 8–9.
- Huang, B., Subramaniam, S., Frey, J., Loh, H., Tan, H.M., Fernandez, C.J. & Kwang, J., Chua, K.L. (2002). Vaccination of ducks with recombinant outer membrane protein (OmpA) and a 41 kDa partial protein (P45N’) of Riemerella anatipestifer. Veterinary Microbiology, 84, 219–230.10.1016/S0378-1135(01)00456-4
- Hvistendahl, M. (2012). China takes aim at rampant antibiotic resistance. Science, 336, 795–795.10.1126/science.336.6083.795
- Kong, Q., Yang, J., Liu, Q., Alamuri, P., Roland, K.L. & Curtiss, R. (2011). Effect of deletion of genes involved in lipopolysaccharide core and O-antigen synthesis on virulence and immunogenicity of Salmonella enterica serovar Typhimurium. Infection and Immunity, 79, 4227–4239.10.1128/IAI.05398-11
- Leclercq, R. & Courvalin, P. (1991). Bacterial resistance to macrolide, lincosamide, and streptogramin antibiotics by target modification. Antimicrobial Agents and Chemotherapy, 35, 1267–1272.10.1128/AAC.35.7.1267
- Liu, H., Wang, X., Ding, C., Han, X., Cheng, A., Wang, S. & Yu, S. (2013). Development and evaluation of a trivalent Riemerella anatipestifer inactivated vaccine. Clinical and Vaccine Immunology, 20, 691–697.10.1128/CVI.00768-12
- Loh, H., Teo, T. & Tan, H.C. (1992). Serotypes of ‘Pasteurella’ anatipestifer isolates from ducks in Singapore: a proposal of new serotypes. Avian Pathology, 21, 453–459.10.1080/03079459208418863
- Martin, J.L. & McMillan, F.M. (2002). SAM (dependent) I AM: the S-adenosylmethionine-dependent methyltransferase fold. Current Opinion in Structural Biology, 12, 783–793.10.1016/S0959-440X(02)00391-3
- Mavromatis, K., Lu, M., Misra, M., Lapidus, A., Nolan, M., Lucas, S. Luca, S., Hammon, N., Deshpande, S., Cheng, J.F., Tapia, R., Han, C., Goodwin, L., Pitluck, S., Liolios, K., Pagani, I., Ivanova, N., Mikhailova, N., Pati, A., Chen, A., Palaniappan, K., Land, M., Hauser, L., Jeffries, C.D., Detter, J.C., Brambilla, E.M., Rohde, M., Göker, M., Gronow, S., Woyke, T., Bristow, J., Eisen, J.A., Markowitz, V., Hugenholtz, P., Klenk, H.P. & Kyrpides, N.C. (2011). Complete genome sequence of Riemerella anatipestifer type strain (ATCC 11845). Standards in Genomic Sciences, 4, 144–153. 10.4056/sigs.1553865
- Pathanasophon, P., Phuektes, P., Tanticharoenyos, T., Narongsak, W. & Sawada, T. (2002). A potential new serotype of Riemerella anatipestifer isolated from ducks in Thailand. Avian Pathology, 31, 267–270.10.1080/03079450220136576
- Pathanasophon, P., Sawada, T. & Tanticharoenyos, T. (1995). New serotypes of Riemerella anatipestifer isolated from ducks in Thailand. Avian Pathology, 24, 195–199.10.1080/03079459508419059
- Pathanasophon, P., Sawada, T., Pramoolsinsap, T. & Tanticharoenyos, T. (1996). Immunogenicity of Riemerella anatipestifer broth culture bacterin and cell-free culture filtrate in ducks. Avian Pathology, 25, 705–719.10.1080/03079459608419176
- Penuelas-Urquides, K., Villarreal-Trevino, L., Silva-Ramirez, B., Rivadeneyra-Espinoza, L., Said-Fernandez, S. & de Leon, M.B. (2013). Measuring of Mycobacterium tuberculosis growth: a correlation of the optical measurements with colony forming units. Brazilian Journal of Microbiology, 44, 287–289.10.1590/S1517-83822013000100042
- Poehlsgaard, J. & Douthwaite, S. (2005). The bacterial ribosome as a target for antibiotics. Nature Reviews Microbiology, 3, 870–881.10.1038/nrmicro1265
- Poole, K. (2007). Efflux pumps as antimicrobial resistance mechanisms. Annals of Medicine, 39, 162–176.10.1080/07853890701195262
- Qin, S., Wang, Y., Zhang, Q., Zhang, M., Deng, F., Shen, Z., Wu, C., Wang, S., Zhang, J. & Shen, J. (2014). Report of ribosomal RNA methylase gene erm(B) in multidrug-resistant Campylobacter coli. Journal of Antimicrobial Chemotherapy, 69, 964–968.10.1093/jac/dkt492
- Rasmussen, J.L., Odelson, D.A. & Macrina, F.L. (1986). Complete nucleotide sequence and transcription of ermF, a macrolide-lincosamide-streptogramin B resistance determinant from Bacteroides fragilis. Journal of Bacteriology, 168, 523–533.
- Roberts, M.C., Sutcliffe, J., Courvalin, P., Jensen, L.B., Rood, J. & Seppala, H. (1999). Nomenclature for macrolide and macrolide-lincosamide-streptogramin B resistance determinants. Antimicrobial Agents and Chemotherapy, 43, 2823–2830.
- Roland, K., Curtiss III, R. & Sizemore, D. (1999). Construction and evaluation of a Δcya Δcrp Salmonella typhimurium strain expressing avian pathogenic Escherichia coli O78 LPS as a vaccine to prevent airsacculitis in chickens. Avian Diseases, 43, 429–441.10.2307/1592640
- Ruiz, J. & Sandhu, T. (2013). Riemerella anatipestifer infection. In Y.M. Saif, A.M. Fadly, J.R. Glisson, L.R. McDougald, L.K. Nolan, D.E. Swayne (Eds.), Diseases of Poultry 13th edn (pp. 407–465). Iowa: Blackwell Publishing.
- Salyers, A.A., Shoemaker, N.B., Stevens, A.M. & Li, L.Y. (1995). Conjugative transposons: an unusual and diverse set of integrated gene transfer elements. Microbiology and Molecular Biology Reviews, 59, 579–590.
- Sandhu, T. & Harry, E. (1981). Serotypes of Pasteurella anatipestifer isolated from commercial White Pekin ducks in the United States. Avian Diseases, 25, 497–502. 10.2307/1589941
- Smith, C.J. (1987). Nucleotide sequence analysis of Tn4551: use of ermFS operon fusions to detect promoter activity in Bacteroides fragilis. Journal of Bacteriology, 169, 4589–4596.
- Subramaniam, S., Huang, B., Loh, H., Kwang, J., Tan, H.M., Chua, K.L. & Frey, J. (2000). Characterization of a predominant immunogenic outer membrane protein of Riemerella anatipestifer. Clinical and Vaccine Immunology, 7, 168–174. 10.1128/CDLI.7.2.168-174.2000
- Sun, N., Liu, J.H., Yang, F., Lin, D.C., Li, G.H., Chen, Z.L. & Zeng, Z.L. (2012). Molecular characterization of the antimicrobial resistance of Riemerella anatipestifer isolated from ducks. Veterinary Microbiology, 158, 376–383. 10.1016/j.vetmic.2012.03.005
- Takaya, A., Sato, Y., Shoji, T. & Yamamoto, T. (2013). Methylation of 23S rRNA nucleotide G748 by RlmAII methyltransferase renders Streptococcus pneumoniae telithromycin susceptible. Antimicrobial Agents and Chemotherapy, 57, 3789–3796. 10.1128/AAC.00164-13
- Vester, B. & Long, K.S. (2009). Antibiotic resistance in bacteria caused by modified nucleosides in 23S ribosomal RNA. In H. Grosjean (Ed.), DNA and RNA Modification Enzymes: Structure, Mechanism, Function and Evolution (pp. 537–549). Austin, TX: Landes Bioscience.
- Wang, X., Zhu, D., Wang, M., Cheng, A., Jia, R., Zhou, Y., Chen, Z., Luo, Q., Liu, F., Wang, Y. & Chen, X.Y. (2012). Complete genome sequence of Riemerella anatipestifer reference strain. Journal of Bacteriology, 194, 3270–3271. 10.1128/JB.00366-12
- Wang, X., Liu, W., Zhu, D., Yang, L., Liu, M., Yin, S., Wang, M., Jia, R., Cheng, A. & Chen, X. (2014). Comparative genomics of Riemerella anatipestifer reveals genetic diversity. BMC Genomics, 15, 479. 10.1186/1471-2164-15-479
- Weisblum, B. (1995). Erythromycin resistance by ribosome modification. Antimicrobial Agents and Chemotherapy, 39, 577–585. 10.1128/AAC.39.3.577
- Yang, F.F., Sun, Y.N., Li, J.X., Wang, H., Zhao, M.J., Su, J., Zhang, Z.J., Liu, H.J. & Jiang, S.J. (2012). Detection of aminoglycoside resistance genes in Riemerella anatipestifer isolated from ducks. Veterinary Microbiology, 158, 451–452. 10.1016/j.vetmic.2012.02.027
- Yuan, J., Liu, W., Sun, M., Song, S., Cai, J. & Hu, S. (2011). Complete genome sequence of the pathogenic bacterium Riemerella anatipestifer strain RA-GD. Journal of Bacteriology, 193, 2896–2897. 10.1128/JB.00301-11
- Zhong, C.Y., Cheng, A.C., Wang, M.S., Zhu, D.K., Luo, Q.H., Chuan, D.Z., Li, L. & Duan, Z. (2009). Antibiotic susceptibility of Riemerella anatipestifer field isolates. Avian Diseases, 53, 601–607. 10.1637/8552-120408-ResNote.1
- Zhong, C.Y., Cheng, A.C., Wang, M. S., Zhu, D.K., Luo, Q.H., Chen, S., Zhang, S.H. & Chen, X.Y. (2013). Quantitative real-time PCR study of the expression and regulation of the tetracycline resistance gene in Riemerella anatipestifer. Poultry Science, 92, 1552–1559. 10.3382/ps.2012-02672
- Zhou, Z., Peng, X., Xiao, Y., Wang, X., Guo, Z., Zhu, L., Liu, M., Jin, H., Bi, D., Li, Z. & Sun, M. (2011). Genome sequence of poultry pathogen Riemerella anatipestifer strain RA-YM. Journal of Bacteriology, 193, 1284–1285. 10.1128/JB.01445-10