ABSTRACT
Field observations indicate that the impact of velogenic Newcastle disease virus (vNDV) is more severe in countries with concomitant circulation of low pathogenicity avian influenza virus, as is the case in the Middle East, in particular in Israel, where H9N2 and NDV are endemic. In our study, we evaluated how the exposure of chickens to an H9N2 challenge either favours or interferes with a subsequent vNDV infection and its transmission to sentinels. For this purpose, single vNDV and sequential H9/NDV challenges were performed with increasing doses of vNDV (101–106 EID50). The H9N2 challenge made birds more susceptible to the vNDV, lowering the minimum dose required to cause an infection, exacerbating the clinical outcome, while delaying the onset of the disease and time of death. Interestingly, the presence and degree of these seemingly contrasting effects were dose-dependent and not mutually exclusive.
Introduction
Avian influenza (AI) and Newcastle disease virus (NDV) are two of the most serious diseases of poultry. In the Middle East, an ongoing epizootic of low pathogenicity avian influenza (LPAI) of the H9N2 subtype has been favouring the occurrence of NDV-AI mixed infections in backyard and commercial farms of layers, breeders, broilers and turkeys, since the year 2000 (Davidson et al., Citation2014). In Israel, vaccination with inactivated H9N2 whole-virus vaccines is voluntary, while immunization against velogenic NDV (vNDV) is mandatory (DG SANCO, Citation2014; Miller et al., Citation2015). Nevertheless, even in the presence of good flock immunity against NDV, vaccinal breaks of vNDV have caused drops in egg production and mortality events often reported in association with H9N2 infections (Banet-Noach et al., Citation2007), suggesting a role of this pathogen in the sustained circulation of NDV in the region.
Although H9N2 viruses alone can cause moderate to severe respiratory infections in chickens, replicating beyond the respiratory and digestive tracts (Gharaibeh, Citation2008; Mosleh et al., Citation2009), it has been speculated that H9N2 viruses can either act as immunosuppressive agents (Xing et al., Citation2009) or cause epithelial damage at the level of the respiratory tract, facilitating secondary bacterial or viral infections (Kishida et al., Citation2004; Haghighat-Jahromi et al., Citation2008).
The investigation of the interplay between NDV and AI viruses under experimental settings offers valuable insights into the impact of co-infection on virulence, infectivity, virus shedding and seroconversion, helping to adjust surveillance and diagnostic activities in the case of co-circulation of these diseases.
Extensive research has been made through both in vitro and in vivo studies in poultry species, on the interaction between mesogenic, velogenic NDV viruses and AI strains of the low and highly pathogenic type (LPAI/HPAI) (Costa-Hurtado et al., Citation2014, Citation2015, Citation2016; Pantin-Jackwood et al., Citation2015), but relatively few data are available on the role of H9N2 viruses as exacerbating or interfering agents on a sequential vNDV infection.
In fact, the existing literature regarding H9N2 co-infection studies only refers to experimental settings in which different combinations of H9N2 and either non-lethal pathogens or immunosuppressive agents are investigated (Kishida et al., Citation2004; Haghighat-Jahromi et al., Citation2008; Kwon et al., Citation2008; Chu et al., Citation2016). Results published so far prove that the clinical picture of co-infected birds is exacerbated, partially accounting for the discrepancy between H9N2 disease outbreaks in the field, and the apparent low pathogenicity of the virus when tested as a pure single agent, under experimental conditions.
Nevertheless, the role of the H9N2 AI virus as either a triggering or an exacerbating agent of a heterologous primary lethal infectious pathogen has never been studied.
Moreover, previous experimental studies did not test isolates that were actually co-circulating in the field. In this study, we opted for a different approach, selecting as challenge viruses H9N2 LPAI and vNDV strains isolated in Israel, during surveillance activities in commercial farms in 2011. To test the hypothesis of a disrupting role of H9N2 on vNDV infections in experimental conditions, we challenged specific-pathogen-free (SPF) chickens with a fixed dose of H9N2 and subsequently inoculated the birds with different doses of vNDV. Introduction of sentinels after the second challenge aimed at evaluating the impact of concurrent infections on the transmissibility of the two viruses.
Materials and methods
Viruses
A LPAI virus of the H9N2 subtype, G1 lineage A/chicken/Israel/1163/2011 and a VII genotype vNDV, APMV1/chicken/Israel/Maaleh-Hachamsha/998/2011 were isolated at the Kimron Veterinary Institute from swabs collected during surveillance activities in Israel. Viral stocks were produced by inoculating 9-to-11-day-old embryonated SPF hen’s eggs via the allantoic cavity and were subsequently titrated according to the Reed and Muench method (Reed & Muench, Citation1938). The stocks were screened to exclude the presence of bacterial agents and common viruses of poultry as previously described (Bonfante et al., Citation2014). To confirm the low pathogenicity of the H9N2 virus we pathotyped the new stock by calculating the intravenous pathogenicity index in accordance with standard protocols (World Organisation for Animal Health (OIE), Citation2015). The intracerebral pathogenicity index of the APMV1 strain is 1.63 as previously reported by Miller et al. (Citation2015).
Birds and experimental infections
For this study, 180 SPF day-old White Leghorn chicks were housed in negative, high efficiency particulate air filtered poultry isolators, at the facilities of the Istituto Zooprofilattico Sperimentale delle Venezie. Birds received feed and water ad libitum.
Animal experiment procedures were conducted in strict accordance with the Decree of the Ministry of Health n. 26 of 4 March 2014 on the protection of animals used for scientific purposes, implementing Directive 2010/63/EU, and approved by the Institute’s Ethics Committee (n. 5/2014).
In the first experiment, six groups of 10 six-week-old chickens were challenged with 100 µl of six batches of allantoic fluid containing increasing infectious doses (from 101 to 106 EID50) of the vNDV challenge virus. Birds were inoculated via the oro-nasal route.
In the second experiment, the same protocol of infection was applied, but three days prior to the vNDV challenge, birds were dosed via the oro-nasal route 106 EID50 of the H9N2 challenge virus.
In both the experiments, two days after the vNDV challenge, five sentinels were introduced into each group and were cohoused with the challenged birds for 18 days. On days 2, 4, 6 and 8 post infection (p.i.) or post contact (p.c.) all birds were sampled for the collection of tracheal and cloacal swabs. Clinical data were recorded daily, and at the end of the trial blood samples were collected to assess seroconversion to both viruses.
Time of decease (number of days from challenge to death) and the onset of NDV tracheal and cloacal shedding (number of days from challenge to detection of viral RNA in swabs) were recorded, to calculate mean death time (MDT) and mean shedding onset time (MSOT), respectively. These parameters were compared to evaluate the degree of interference of the two viruses in terms of timing of infection.
Groups were named after the challenge and NDV dose (i.e. group NDV-102, group H9/NDV-102).
Transmissibility of each virus was calculated as the percentage of birds that shed virus RNA by either the tracheal or the cloacal route, for at least one day.
Virus detection and quantification
Viral RNA was extracted from 50 ml of phosphate-buffered saline containing a swab suspension using the Ambion MagMax-96 AI-ND Viral RNA Isolation kit (Ambion®, Austin, TX, USA) on the MICROLAB® STARlet bench-top liquid handling system (Hamilton Robotics, Reno, NV, USA). Isolated RNA was analysed by quantitative real-time reverse transcription polymerase chain reaction (qRRT-PCR) for the identification and quantification of the influenza A virus matrix gene using the published probes and primers from Spackman et al. (Citation2002). For the identification and quantification of the NDV RNA-dependent RNA polymerase (L) gene a qRRT-PCR was carried out on the same RNA using the fluorescein amidite probe LproMGB and the primer set NDF/NDR. The oligonucleotide sequences were kindly provided by the Animal and Plant Health Agency, UK.
The qRRT-PCRs for AI and NDV RNA detection were performed using the QuantiTec® Multiplex RT-PCR kit (Quiagen, Hilden, Germany) and carried out on Applied Biosystems 7300 Real-Time PCR system (Applied Biosystem, Carlsbad, CA, USA) and Bio-Rad CFX96 Real-time PCR system (Bio-Rad Laboratories, Hercules, CA, USA), respectively. The RT step conditions for both primer sets were 20 min at 50°C and 15 min at 95°C. The cycling conditions for AIV were 40 cycles of 45 s at 94°C, followed by 45 s at 60°C; and for NDV were 40 cycles of 45 s at 94°C, followed by 45 s at 50°C.
Standard curves for virus quantification were generated with RNA extracted from 10-fold dilutions of the titrated challenge viruses and reported as EID50/100 µl equivalents. Each dilution was extracted, and was analysed in triplicate. Virus dilutions and trial samples were extracted in different sessions to avoid contaminations. AIV and NDV standard curves were analysed with the ABI 7300 and Bio-Rad software, respectively. According to the standard curves generated, the linear equations for the qRRT-PCR for AIV and NDV were y = −3.283x + 34.863 and y = −3.3498x + 46.904, respectively. The R2 index values, which represent the correlation coefficient of a standard curve in the linear regression analysis, were 0.998 and 0.984, while the slopes (m) were −3.28 and −3.35 for AIV and NDV, respectively. The efficiencies of the reaction, defined by 10(−1/slope), were 2.02 and 1.99, which is within the acceptable range of 1.7–2.2 (18).
Statistics
Shedding data expressed in EID50/100 µl equivalents were averaged, and compared among groups using a Mann–Whitney test with a two-tailed P-value. Negative samples were assigned a value of 0.00 EID50/100 µl and averaged with positive samples. MDT and MSOT were compared using a two-way ANOVA model corrected for multiple comparison with the Bonferroni test.
Statistical analyses were carried out using the GraphPad Prism 5.0 software (GraphPad Prism, GraphPad Software, La Jolla, CA, USA).
Serology
To evaluate post-infection seroconversion, the haemagglutination inhibition test (HI) was carried out using homologous antigens to the challenge viruses, according to the OIE procedure (World Organisation for Animal Health (OIE), Citation2015).
Results
Clinical data
In both experiments, mortality rates of challenged birds were proportional to the NDV dose as opposed to the sentinels, in which the severity of the disease showed no clear pattern across groups (, Tables S1 and S2). In the H9/NDV and NDV groups, mortality among challenged birds ranged between 0 and 100% and 0 and 70%, respectively. Mortality rates among challenged birds were higher in H9/NDV-103, 104, 105 and 106 groups than in the corresponding NDV groups (, Table S1).
Figure 1. Mortality rates of challenged (a) and sentinel birds (b). Birds were monitored for 21 days after the challenge/contact.
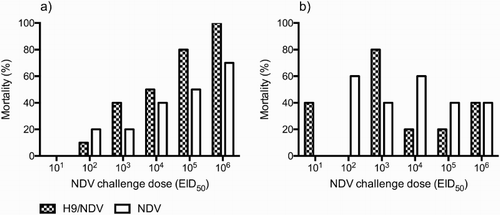
In the NDV-101 group, challenged and sentinel birds did not exhibit signs of infection, and mortality was not recorded. On the other hand, in the H9/NDV-101 group, the challenge elicited a mild respiratory infection such as reddening of the eye mucosa, ruffling of the feathers and inactivity, in both challenged and sentinel birds. In this group, two sentinels became severely sick and died 11 days post contact (p.c.).
Groups challenged with 102 EID50 of the NDV virus differed greatly between the two experiments, as in the single infection, two challenged birds and three sentinels died, while in the H9/NDV challenge, only one challenged bird died and all of the sentinels survived.
In H9/NDV-102–6 groups, MDT was inversely proportional to the NDV challenge dose, ranging between 12.00 and 5.50 dpi (, Table. S1). In NDV-102–4 groups, MDT ranged between 12.00 and 6.25 dpi, and values were inversely proportional to the NDV challenge dose, while in NDV-105–6 groups, the MDT plateaued at a value of approximately 6 dpi, irrespective of the NDV challenge dose.
Figure 2. NDV tracheal mean shedding onset time (MSOT) (a) and mean death time (b) of challenged birds. The NDV tracheal MSOT was calculated by averaging the number of days post infection when NDV was first detected in tracheal swabs by qRRT-PCR. *P < 0.05; ***P < 0.0005.
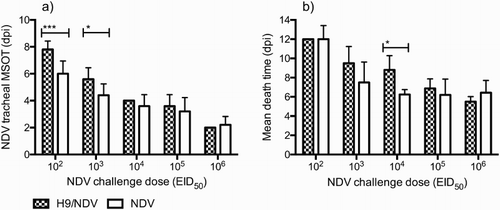
Comparison of MDT between the two experiments recorded higher values among H9/NDV-103–5 compared with the corresponding NDV groups, although statistical significance was observed only for the H9/NDV-104 group (P < 0.05).
Shedding and seroconversion of challenged birds
In agreement with the recorded clinical picture, all of the swabs and sera collected from the NDV-101 group were NDV negative, while all of the birds in the H9/NDV-101 group shed NDV virus RNA by both the tracheal and the cloacal routes, and seroconverted to the NDV antigen.
In all of the remaining groups, each bird shed NDV viral RNA from the trachea and the cloaca and seroconverted to the NDV virus, confirming the occurrence of infection.
In both of the experiments, tracheal and cloacal MSOT were inversely proportional to the administered NDV challenge dose (, Table S1).
In the NDV groups, NDV virus RNA was detected in the trachea, starting on average between 6.00 and 2.20 dpi, while in all of the corresponding H9/NDV groups, the shedding started hours to days later, except for the H9/NDV-106 group (Table S1). Delays resulted in statistical significance for the 102 (P < 0.0005) and 103 (P < 0.05) groups (, Table S1).
Comparison of the cloacal MSOT between the two experiments identified the same trend but lacked statistical significance (Table S1).
In each NDV group, tracheal shedding of NDV peaked on day 4 p.i., reaching mean values in the range of 107–108 EID50/100 µl, while in the H9/NDV groups the peak of shedding was postponed to either the sixth or the eighth day after the challenge ().
Figure 3. NDV tracheal shedding expressed as EID50/0.1 ml equivalents, after transformation of qRRT-PCR Ct values using a calibration curve. Means and standard deviations are shown for each sampling time. *P < 0.05; **P < 0.005; ***P < 0.0005; ****P < 0.00005.
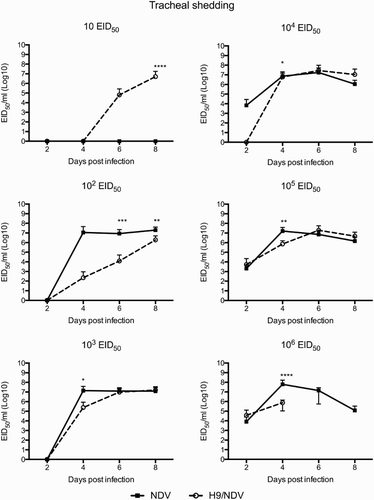
In H9/NDV 103, 104, 105 and 106 groups, mean EID50/100 µl equivalents of NDV virus in tracheal swabs were significantly lower than in the corresponding NDV groups, on day 4 p.i., while for 102 groups the same trend was recorded on both days 6 and 8 p.i. ().
Similarly, mean EID50/100 µl equivalents in cloacal swabs were significantly lower at one sampling time for each H9/NDV group, apart for the H9/NDV-103 group in which shedding was higher than in the corresponding NDV group, on day 8 p.i. ().
Figure 4. NDV cloacal shedding expressed as EID50/0.1 ml equivalents, after transformation of qRRT-PCR Ct values using a calibration curve. Means and standard deviations are shown for each sampling time. *P < 0.05; **P < 0.005; ***P < 0.0005.
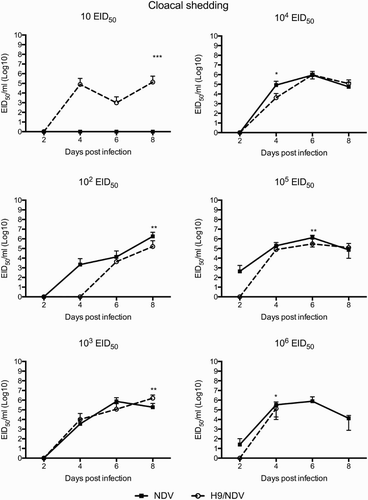
Within each experiment, mean HI titres to the NDV antigen did not differ significantly across the groups.
The comparison of mean NDV HI titres between the two experiments highlighted higher values in the NDV groups than in the H9/NDV groups. In particular, we found statistical support for 104 and 105 groups (P < 0.05) (Table S1).
The H9N2 virus replicated in the trachea of all challenged birds reaching peak values in the range of 103.48–104.15EID50/ml, on day 3 p.i. (Figure S1).
Replication of the H9N2 in the intestines was scarce, as few birds shed virus RNA from the cloaca and the average EID50 equivalents were 2–3 Log10 lower than the ones recorded in the trachea (Figure S1).
Replication dynamics of the H9N2 virus in the trachea were the same across the H9/NDV groups, as the differences in mean H9N2 EID50/100 µl equivalents were not statistically significant, apart for the H9/NDV-101 group, in which shedding on day 3 p.i. was significantly higher (P < 0.05) compared to all other groups (Figure S1). On the other hand, values of cloacal shedding of H9N2, although graphically heterogeneous among groups, were not statistically different.
The comparison of mean HI titres against the H9N2 virus identified the H9/NDV-101 group as the one recording the highest seroconversion, and statistically significant differences were observed with 103 and 104 groups (Table S1).
Shedding and seroconversion of sentinels
Transmission of and seroconversion to the NDV virus occurred in all sentinels of all groups but the NDV-101 group, in which challenged birds did not develop an NDV infection, and hence could not transmit the virus. Comparison of NDV tracheal and cloacal MSOT between H9/NDV and NDV groups recorded no significant difference in the onset of infection, a part for the sentinels in group H9/NDV-102 (Table S2).
Mean HI titres to the NDV virus were comparable to the ones of the challenged birds and did not differ between the sentinels of the H9/NDV and NDV groups (Table S2).
Transmission of and seroconversion to the H9N2 virus occurred in all of the sentinels in groups H9/NDV-101, 102, 103 and 105, while none of the sentinels in the H9/NDV-104 and 106 groups either shed or seroconverted. In the H9/NDV-101 group H9N2 MSOT was significantly higher (P < 0.05) than in the rest of the groups. Differences in geometric mean titer to the H9N2 antigen were not statistically significant among groups (Table S2).
Discussion
The scope of this study was to reproduce and investigate under experimental conditions the incursion of a vNDV strain in an unvaccinated flock of chickens where an H9N2 AI virus is circulating. To evaluate the role of the H9N2 virus on the evolution of the NDV disease, we had to establish two identical experimental settings, in which the only difference was the presence/absence of the AI virus. We focused on clinical, virological and serological data, for both challenged and contact sentinels, to characterize the dynamics of infection and transmission of both pathogens.
In our setup, the H9N2 challenge dose was identical for all groups, while we opted for serial dilutions of the subsequent vNDV challenge, in order to be able to assess changes in the susceptibility of birds to a broad spectrum of vNDV challenge doses.
The virulence of the vNDV virus result was in keeping with the intracerebral pathogenicity index of the isolate (Cattoli et al., Citation2011), as the highest dose failed to elicit a 100% mortality in the NDV-106 group, and the lowest dose (NDV-101) could not establish an infection, as confirmed by both virological and serological data. The intermediate virulence of this strain turned out to be highly informative for our purposes, allowing us to observe changes in the phenotype of infection at both ends of the spectrum.
A prior exposure of the birds to a high dose of the H9N2 virus proved to increase their susceptibility to the vNDV infection, lowering its minimum infectious dose from 102 to 101 EID50/100 µl, as the NDV virus replicated and transmitted in all of the challenged and sentinel birds in the H9/NDV-101 group.
In a previous experiment (manuscript in preparation), we proved that the H9N2 challenge strain did not elicit a deadly infection, as 100% of the SPF birds survived an oro-nasal 106 EID50 challenge, developing only mild clinical signs. Nevertheless, in this study the direct comparison of groups indicated a role of the H9N2 virus as an exacerbating agent of the subsequent vNDV infection, increasing the mortality rates of challenged birds, starting from a challenge dose of 103.
A growing body of data from in vitro and in vivo studies suggests that H9N2 might act as an immunosuppressive agent, hampering vaccination responses, causing degeneration of lymphatic organs (Qiang & Youxiang, Citation2011), leading to apoptotic processes in different types of cells (Xing et al., Citation2011) and suppressing the expression of interferon (IFN) and IFN-inducible genes (Xing et al., Citation2008). If some or all of these conditions were met in our studies, a temporary dysfunction in the host’s immune response might have led to a more severe replication of the vNDV in vital organs, hence increasing mortality.
Moreover, the H9N2 challenge strain showed a marked tropism for the upper respiratory tract, confirming published findings (Iqbal et al., Citation2013; Zhu et al., Citation2015). This evidence, although not supported by pathological examinations in this study, leads us to assume that the robust replication in the trachea might have caused deciliation of the respiratory epithelium, as previously reported in other pathogenesis studies (Barbour et al., Citation2006; Pantin-Jackwood & Swayne, Citation2009), facilitating the colonization of the more severe secondary vNDV infection.
Paradoxically, both the onset of the vNDV replication and the mean time of death among challenged birds were delayed by the pre-existing H9N2 replication, indicating an interference of the latter in the establishment of the vNDV infection. A detailed evaluation of the shedding dynamics confirmed this interpretation, as the H9/NDV groups recorded a delay in the peak of vNDV tracheal and cloacal shedding, while we observed an overall uniformity of viral loads at later time points. Interestingly, in the H9/NDV-102 group, for which the delay in tracheal shedding was the most significant, the loads of shed virus throughout the study were strongly reduced, in particular at the level of the trachea.
A comprehensive analysis of all data sets indicates that the degrees of H9N2 interference and exacerbation varied depending on the dose of the subsequent vNDV challenge.
In fact, at the lowest comparable dose (H9/NDV-102) replication of vNDV was both delayed and reduced at all sampling times, and no exacerbation of clinical disease was recorded. In the H9/NDV-103 group, vNDV replication was delayed and reduced but to a lower extent (up to day 4 p.i.) and with less statistical significance than in the H9/NDV-102 group, while this was the first group in which the mortality rate was increased.
At higher doses of NDV (104 and 105), the reduction in viral loads was similar to the one recorded for the H9/NDV-103 group, but the delays in the onset of tracheal shedding were not statistically significant, while we recorded an increase in mortality for both groups.
At the highest dose (106), we still observed a reduction in the replication of NDV at the tracheal level on day 4 p.i. and a clear exacerbation of the disease associated with the highest mortality rate. Paradoxically, in this group, although in the absence of statistical significance, MSOT and MDT were shorter than in NDV groups, inverting the expected trend in terms of time interference.
In our interpretation, this complex dataset portrays a temporary, local interference of H9N2 for the establishment of the subsequent vNDV infection that is overcome by intermediate-high doses of vNDV, while synergy of the two viruses translates unambiguously into higher susceptibility of animals to vNDV and higher mortality rates. To speculate further on this, we should take into account that the interference we observed at the level of the trachea and the cloaca is not representative of what occurs outside of the respiratory and digestive tracts, where vNDV theoretically replicates in the absence of H9N2.
Since the discovery of the IFN in the 1940–1950s (Henle & Henle, Citation1943; Gale, Citation2015), viral interference has been deeply investigated in the field of both medical and veterinary virology and immunology. Interference relies on different mechanisms, and it is particularly effective when pathogens compete for the same cell receptors, enzymes and replication machinery, and when the superinfecting agent engages with a previously infected tissue expressing sufficient levels of IFN. Both challenge viruses in this study are single-stranded, negative-sense RNA viruses that require enzymatic cleavage of their attachment glycoproteins to establish an infection. Moreover, both viruses have a tropism for the respiratory epithelium, and depend on the presence of N-linked glycoproteins as cell receptors (Ferreira et al., Citation2004).
Upon LPAI infection, chickens express peak levels of IFN-α and IFN-β mRNA at the level of the lungs, on the first day p.i. (Cornelissen et al., Citation2012). Expression of IFN drops from day 2 onwards, probably as a result of virus replication and the associated accumulation of the NS1 protein, a potent IFN-inhibitor (Xing et al., Citation2009).
In light of all of these considerations, we speculate that interference with the vNDV virus, after three days from the H9N2 challenge, is hardly attributable to the anti-viral state induced by the expression of the IFN. On the other hand, considering that the vNDV challenge occurred at the peak of H9N2 replication at the level of the trachea, the most probable cause for the observed interference is competition at the site of infection for receptors, enzymes and host cell machinery. In agreement with this hypothesis, the decreasing replication of the H9N2 on day 4 after the vNDV infection (day 7 after the H9N2 challenge) coincided with the end of interference, as viral loads in the trachea were statistically identical, at later sampling times.
Extensive research has been carried out (Costa-Hurtado et al., Citation2014, Citation2016) in different poultry species, to study through either co-infections or sequential infections several combinations of LPAI/HPAI viruses with lentogenic, mesogenic and velogenic NDV strains. Given the different experimental settings and selection of challenge viruses, a direct comparison of our data with the available literature is not possible. Nevertheless, we can establish a parallelism between our results and those reported in the literature.
Findings from Ge et al. (Citation2012) showed that AIVs have a replicative advantage over NDVs in SPF chicken embryos when co-inoculated, and that this effect is even more evident if AIV is administered 12–24 h before NDV. Interestingly though, the degree of interference was dramatically reduced if the NDV challenge virus was a virulent strain instead of a LaSota virus.
In our studies, tracheal shedding in the range of 103–4 EID50/ml of a LPAI virus consistently interfered with 102–3 EID50/0,1 ml challenge doses of a virulent NDV strain, while higher doses of the NDV virus were less affected. Interestingly, irrespective of the subsequent vNDV challenge dose, the replication of the H9N2 virus was not affected by the virulent superinfection, a finding that supports the previously described replicative advantage of AIV during NDV superinfections.
Transmission of viral diseases is a multifactorial phenomenon mainly depending on the combination of variables such as shedding levels of primary infected birds, number and frequency of functional contacts between birds, viral load in the environment and time of exposure.
In our experimental setup, we observed transmission of the vNDV strain in all sentinels of all groups, while mortality differed greatly and with no relation to the vNDV challenge dose. This is not surprising, considering that many factors varied independently across the groups, eventually resulting in different overall exposure levels of sentinels to the viruses.
Interestingly, H9N2 did not transmit to any sentinel in groups H9/NDV-104 and 106, while in the rest of the groups all birds were infected. A partial explanation for this is that the sentinels were introduced two days after the vNDV challenge (day 5 after the H9N2 challenge), implying that they were exposed to chickens in which the H9N2 infection and shedding were declining, while the vNDV virus was yet to reach its replicative peak.
Co-circulation of H9N2 and vNDV is one of the main burdens of poultry production in the Middle East, in particular in Israel, where both diseases are endemic at the time of writing.
Although vaccination against both viruses has been implemented, together with stamping out, quarantine and movement control measures in response to outbreaks of NDV, reports of new cases have not declined.
Results from this study indicate that in the absence of immunity, H9N2 viruses circulating in the field might have the ability to make birds more susceptible to a superinfection of vNDV, both lowering the minimum dose required to establish an infection, and exacerbating clinical signs.
Moreover, our findings indicate that the presence of H9N2 might delay and decrease the shedding of NDV, extending the period during which the virus replicates in the flock at undetectable levels. Nevertheless, we should bear in mind that these results were generated in a single experiment in SPF birds, under controlled conditions that reproduced a homogeneous superinfection of NDV, three days after an equally homogeneous H9N2 challenge of the flock. In light of this, we should bear in mind that outbreaks in the field are often heterogeneous in terms of both viral load and timing of infections across the flock, and therefore our findings might not be representative of all the complex scenarios in the field.
Concluding, the co-circulation of these viruses has the potential not only to worsen the severity of NDV infections but also to confound and delay the correct diagnosis of the disease, eventually postponing the implementation of effective emergency control measures.
Supplemental data
Download Zip (461.6 KB)Additional information
Funding
References
- Banet-Noach, C., Perk, S., Simanov, L., Grebenyuk, N., Rozenblut, E., Pokamunski, S., Pirak, M., Tendler, Y., & Panshin, A. (2007). H9N2 influenza viruses from Israeli poultry: a five-year outbreak. Avian Diseases, 51, 290–296. doi: 10.1637/7590-040206R1.1
- Barbour, E.K., El-hakim, R.G., Kaadi, M.S., Shaib, H.A., Gerges, D.D., & Nehme, P.A. (2006). Evaluation of the histopathology of the respiratory system in essential oil-treated broilers following a challenge with Mycoplasma gallisepticum and/or H9N2 influenza virus. The International Journal of Applied Research in Veterinary Medicine, 4, 293–300.
- Bonfante, F., Fusaro, A., Zanardello, C., Patrono, L.V., De Nardi, R., Maniero, S., & Terregino, C. (2014). Lethal nephrotropism of an H10N1 avian influenza virus stands out as an atypical pathotype. Veterinary Microbiology, 173, 189–200. doi: 10.1016/j.vetmic.2014.07.023
- Cattoli, G., Susta, L., Terregino, C., & Brown, C. (2011). Newcastle disease: a review of field recognition and current methods of laboratory detection. Journal of Veterinary Diagnostic Investigation, 23, 637–656. doi: 10.1177/1040638711407887
- Chu, J., Zhang, Q., Zhang, T., Han, E., Zhao, P., Khan, A., He, C., & Wu, Y. (2016). Chlamydia psittaci infection increases mortality of avian influenza virus H9N2 by suppressing host immune response. Scientific Reports, 6, 29421. doi: 10.1038/srep29421
- Cornelissen, J.B.W.J., Post, J., Peeters, B., Vervelde, L., & Rebel, J.M.J. (2012). Differential innate responses of chickens and ducks to low-pathogenic avian influenza. Avian Pathology, 41, 519–529. doi: 10.1080/03079457.2012.732691
- Costa-Hurtado, M., Afonso, C.L., Miller, P.J., Shepherd, E., Cha, R.M., Smith, D., Spackman, E., Kapczynski, D.R., Suarez, D.L., Swayne, D.E., & Pantin-Jackwood, M.J. (2015). Previous infection with virulent strains of Newcastle disease virus reduces highly pathogenic avian influenza virus replication, disease, and mortality in chickens. Veterinary Research, 46, 97. doi: 10.1186/s13567-015-0237-5
- Costa-Hurtado, M., Afonso, C.L., Miller, P.J., Shepherd, E., DeJesus, E., Smith, D., & Pantin-Jackwood, M.J. (2016). Effect of infection with a mesogenic strain of Newcastle disease virus on infection with highly pathogenic avian influenza virus in chickens. Avian Diseases, 60, 269–278. doi: 10.1637/11171-051915-Reg
- Costa-Hurtado, M., Afonso, C.L., Miller, P.J., Spackman, E., Kapczynski, D.R., Swayne, D.E., Shepherd, E., Smith, D., Zsak, A., & Pantin-Jackwood, M. (2014). Virus interference between H7N2 low pathogenic avian influenza virus and lentogenic Newcastle disease virus in experimental co-infections in chickens and turkeys. Veterinary Research, 45, 1–11. doi: 10.1186/1297-9716-45-1
- Davidson, I., Fusaro, A., Heidari, A., Monne, I., & Cattoli, G. (2014). Molecular evolution of H9N2 avian influenza viruses in Israel. Virus Genes, 48, 457–463. doi: 10.1007/s11262-014-1037-0
- DG SANCO (2014). Final report of an audit carried out in Israel from 10 to 19 June 2014 in order to evaluate the animal health controls in place for poultry and poultry products intended for export to the European Union. European Commission Retrieved from ec.europa.eu/food/fvo/act_getPDF.cfm?PDF_ID=11338.
- Ferreira, L., Villar, E., & Muñoz-Barroso, I. (2004). Gangliosides and N-glycoproteins function as Newcastle disease virus receptors. International Journal of Biochemistry and Cell Biology, 36, 2344–2356. doi: 10.1016/j.biocel.2004.05.011
- Gale, M. (2015). Interference with virus infection. The Journal of Immunology, 195, 1909–1910. doi: 10.4049/jimmunol.1501575
- Ge, S., Zheng, D., Zhao, Y., Liu, H., Liu, W., Sun, Q., Li, J., Yu, S., Zuo, Y., Han, X., Li, L., Lv, Y., Wang, Y., Liu, X., & Wang, Z. (2012). Evaluating viral interference between influenza virus and Newcastle disease virus using real-time reverse transcription-polymerase chain reaction in chicken eggs. Virology Journal, 9, 128. doi: 10.1186/1743-422X-9-128
- Gharaibeh, S. (2008). Pathogenicity of an avian influenza virus serotype H9N2 in chickens. Avian Diseases, 52, 106–110. doi: 10.1637/8108-090907-Reg
- Haghighat-Jahromi, M., Asasi, K., Nili, H., Dadras, H., & Shooshtari, A.H. (2008). Coinfection of avian influenza virus (H9N2 subtype) with infectious bronchitis live vaccine. Archives of Virology, 153, 651–655. doi: 10.1007/s00705-008-0033-x
- Henle, W., & Henle, G. (1943). Interference of inactive virus with the propagation of virus of influenza. Science, 98, 87–89. doi: 10.1126/science.98.2534.87
- Iqbal, M., Yaqub, T., Mukhtar, N., Shabbir, M.Z., & McCauley, J.W. (2013). Infectivity and transmissibility of H9N2 avian influenza virus in chickens and wild terrestrial birds. Veterinary Research, 44, 100. doi: 10.1186/1297-9716-44-100
- Kishida, N., Sakoda, Y., Eto, M., Sunaga, Y., & Kida, H. (2004). Co-infection of Staphylococcus aureus or Haemophilus paragallinarum exacerbates H9N2 influenza A virus infection in chickens. Archives of Virology, 149, 2095–2104. doi: 10.1007/s00705-004-0372-1
- Kwon, J.-S., Lee, H.-J., Lee, D.-H., Lee, Y.-J., Mo, I.-P., Nahm, S.-S., Kim, M.-J., Lee, J.-B., Park, S.-Y., Choi, I.-S., & Song, C.-S. (2008). Immune responses and pathogenesis in immunocompromised chickens in response to infection with the H9N2 low pathogenic avian influenza virus. Virus Research, 133, 187–194. doi: 10.1016/j.virusres.2007.12.019
- Miller, P.J., Haddas, R., Simanov, L., Lublin, A., Rehmani, S.F., Wajid, A., Bibi, T., Khan, T.A., Yaqub, T., Setiyaningsih, S., & Afonso, C.L. (2015). Identification of new sub-genotypes of virulent Newcastle disease virus with potential panzootic features. Infection, Genetics and Evolution: Journal of Molecular Epidemiology and Evolutionary Genetics in Infectious Diseases, 29, 216–229. doi: 10.1016/j.meegid.2014.10.032
- Mosleh, N., Dadras, H., & Mohammadi, A. (2009). Evaluation of H9N2 avian influenza virus dissemination in various organs of experimentally infected broiler chickens using RT-PCR. Iranian Journal of Veterinary Research, 10, 12–20.
- Pantin-Jackwood, M.J., Costa-Hurtado, M., Miller, P.J., Afonso, C.L., Spackman, E., Kapczynski, D.R., Shepherd, E., Smith, D. & Swayne, D.E. (2015). Experimental co-infections of domestic ducks with a virulent Newcastle disease virus and low or highly pathogenic avian influenza viruses. Veterinary Microbiology, 177, 7–17. doi: 10.1016/j.vetmic.2015.02.008
- Pantin-Jackwood, M.J., & Swayne, D.E. (2009). Pathogenesis and pathobiology of avian influenza virus infection in birds. Revue scientifique et technique (International Office of Epizootics), 28, 113–136.
- Qiang, F., & Youxiang, D. (2011). The effects of H9N2 influenza A on the immune system of broiler chickens in the Shandong Province. Transboundary and Emerging Diseases, 58, 145–151. doi: 10.1111/j.1865-1682.2010.01192.x
- Reed, L.J., & Muench, H. (1938). A simple method of estimating fifty per cent endpoints. American Journal of Epidemiology, 27, 493–497. doi: 10.1093/oxfordjournals.aje.a118408
- Spackman, E., Senne, D.A., Myers, T.J., Bulaga, L.L., Garber, L.P., Perdue, M.L., Lohman, K., Daum, L.T., & Suarez, D.L. (2002). Development of a real-time reverse transcriptase PCR assay for type A influenza virus and the avian H5 and H7 hemagglutinin subtypes. Journal of Clinical Microbiology, 40, 3256–3260. doi: 10.1128/JCM.40.9.3256-3260.2002
- World Organisation for Animal Health (OIE). (2015). Avian influenza. Manual of Diagnostic Tests and Vaccines for Terrestrial Animals 2015, 1, 1–23.
- Xing, Z., Cardona, C.J., Adams, S., Yang, Z., Li, J., Perez, D., & Woolcock, P.R. (2009). Differential regulation of antiviral and proinflammatory cytokines and suppression of Fas-mediated apoptosis by NS1 of H9N2 avian influenza virus in chicken macrophages. Journal of General Virology, 90, 1109–1118. doi: 10.1099/vir.0.007518-0
- Xing, Z., Cardona, C.J., Li, J., Dao, N., Tran, T., & Andrada, J. (2008). Modulation of the immune responses in chickens by low-pathogenicity avian influenza virus H9N2. The Journal of General Virology, 89, 1288–1299. doi: 10.1099/vir.0.83362-0
- Xing, Z., Harper, R., Anunciacion, J., Yang, Z., Gao, W., Qu, B., Guan, Y., & Cardona, C.J. (2011). Host immune and apoptotic responses to avian influenza virus H9N2 in human tracheobronchial epithelial cells. American Journal of Respiratory Cell and Molecular Biology, 44, 24–33. doi: 10.1165/rcmb.2009-0120OC
- Zhu, Y., Yang, Y., Liu, W., Liu, X., Yang, D., Sun, Z., Ju, Y., Chen, S., Peng, D., & Liu, X. (2015). Comparison of biological characteristics of H9N2 avian influenza viruses isolated from different hosts. Archives of Virology, 160, 917–927. doi: 10.1007/s00705-015-2337-y