ABSTRACT
Several phylogenetic clusters of duck Tembusu virus (DTMUV) that caused outbreaks in ducks in Asia have been identified since its emergence in 2010, highlighting the need for an efficient host system that can support isolation of all circulating phylogenetic clusters of DTMUV. In this study, various host systems, including different avian embryonated eggs (duck and chicken) and cell cultures (primary duck embryo fibroblast (DEF), primary chicken embryo fibroblast (CEF), baby hamster kidney (BHK-21), African green monkey kidney (Vero) and Aedes albopictus clone C6/36 (C6/36) cells), were evaluated and compared for their ability to support DTMUV isolation and propagation. Our results showed that all host systems were susceptible to DTMUV infection; however, BHK-21 and primary DEF cells supported more efficient replication of DTMUV compared to the other host systems. BHK-21 cells had the highest DTMUV isolation rate when tested with experimental and field clinical samples. All circulating phylogenetic clusters of DTMUV, including clusters 1, 2 and 3, were successfully isolated from duck clinical samples using BHK-21 cells. In conclusion, our findings supported the use of BHK-21 cells as a host system for primary isolation of all circulating phylogenetic clusters of DTMUV from duck clinical samples. This study highlights the importance of selecting the most appropriate host system for efficient isolation and propagation of DTMUV from duck clinical samples.
RESEARCH HIGHLIGHTS
DTMUV replicated more efficiently in BHK-21 and primary DEF cells than in other host systems tested.
BHK-21 cells had the highest DTMUV isolation rate.
All DTMUV phylogenetic clusters were successfully isolated from the samples using BHK-21 cells.
BHK-21 cells were the most efficient host system for DTMUV isolation.
Introduction
Duck Tembusu virus (DTMUV), a mosquito-borne flavivirus, has been identified as a causative agent of a newly emerging disease in ducks and other avian species, including chickens and geese (Su et al., Citation2011). This disease is primarily characterized by a significant decrease in egg production and severe neurological disorders, including ataxia and paralysis (Su et al., Citation2011). DTMUV is widely distributed and is now endemic in duck populations in Asia, causing significant economic losses to the duck producing industry. DTMUV is a positive-sense single-stranded RNA virus belonging to the genus Flavivirus in the family Flaviviridae (Cao et al., Citation2011; Su et al., Citation2011). Like other single-stranded RNA viruses, DTMUV has a high rate of genetic mutation, resulting in a large degree of genetic diversity (Dai et al., Citation2015; Ninvilai et al., Citation2018, Citation2019). Since its emergence in 2010, several phylogenetic clusters of DTMUV, including cluster 1, cluster 2 and cluster 3, have been identified (Ninvilai et al., Citation2019). This highlights the need for effective diagnostic methods including efficient host systems for virus isolation, which can broadly detect all circulating phylogenetic clusters of DTMUV.
Currently, virus isolation in host systems and/or reverse transcription polymerase chain reaction (RT–PCR) are the primary methods widely used to detect DTMUV in clinical samples (Su et al., Citation2011; Homonnay et al., Citation2014; Thontiravong et al., Citation2015). Besides virus isolation, efficient host systems are also necessary to study various aspects of the DTMUV life cycle, host–pathogen interaction, development of diagnostic tests and vaccines. Therefore, the establishment of an efficient host system for isolation and propagation of all circulating phylogenetic clusters of DTMUV is essential. Several host systems, including different avian embryonated eggs and cell cultures, have been used for DTMUV isolation and propagation (Cao et al., Citation2011; Su et al., Citation2011; O'Guinn et al., Citation2013; Li et al., Citation2014; Tang et al., Citation2015; Thontiravong et al., Citation2015; Zhang et al., Citation2017). However, differences in their ability to support the replication of DTMUV have never been evaluated and the most efficient host system for DTMUV isolation remains unknown. Here, various host systems, including different avian embryonated eggs (duck and chicken) and cell cultures (primary duck embryo fibroblast (DEF), primary chicken embryo fibroblast (CEF), baby hamster kidney (BHK-21), African green monkey kidney (Vero) and Aedes albopictus clone C6/36 (C6/36) cells), were evaluated and compared for their ability to support DTMUV isolation and propagation. The infectivity and replication kinetics, as well as the isolation rate of DTMUV from duck experimental and clinical samples, were examined in these host systems to identify the most suitable host system for isolation of all circulating DTMUV phylogenetic clusters.
Materials and methods
Virus, cells and eggs
The DTMUV strain designated DK/TH/CU-1 was used as a representative strain for evaluating the infectivity and replication kinetics of DTMUV in different host systems. This virus was isolated from DTMUV-infected ducks in Thailand and was genetically classified as DTMUV cluster 2, which is the predominant cluster of DTMUV circulating in duck populations in Asia (Thontiravong et al., Citation2015; Ninvilai et al., Citation2019). Five cell cultures including primary DEF, primary CEF, baby hamster kidney (BHK-21), African green monkey kidney (Vero) and Aedes albopictus clone C6/36 (C6/36) cells were used for evaluation. These cell cultures were chosen because they are often used for isolation and propagation of flaviviruses, including DTMUV (Su et al., Citation2011; O'Guinn et al., Citation2013; Li et al., Citation2014; Tang et al., Citation2015; Zhang et al., Citation2020). Primary DEF and CEF cells were prepared from 10-day-old duck and chicken embryos, respectively. Primary DEF, CEF and Vero cells were maintained in minimal essential medium (MEM) (Invitrogen, Carlsbad, CA, USA) containing 10% foetal bovine serum (FBS) (Invitrogen). BHK-21 cells and C6/36 cells were cultured in Dulbecco's Modified Eagle's Medium (DMEM) (Invitrogen) containing 10% FBS. Primary DEF, primary CEF, BHK-21 and Vero cells were grown and maintained at 37°C, and C6/36 cells at 28°C, with 5% CO2 using standard cell culture procedure. Nine-day-old specific pathogen-free embryonated duck and chicken eggs were used to evaluate the infectivity and replication kinetics as well as the isolation rate of DTMUV from duck clinical samples.
Infectious virus titration in different avian embryonated eggs and cell cultures
The infectivity of DTMUV in different host systems was evaluated by virus titration in various avian embryonated eggs (duck and chicken) and cell cultures (primary DEF, primary CEF, BHK-21, Vero and C6/36 cells) in triplicate. To determine the infectious titre of DTMUV in different cell cultures, 100 µl of 10-fold serial dilution (10−1–10−10) of DK/TH/CU-1 was inoculated into 96-well plates containing each cell culture. The plates were incubated at 37°C or 28°C with 5% CO2 for 5 days and examined microscopically for the presence of cytopathic effect (CPE) (Wang et al., Citation2016). Virus presence was confirmed by DTMUV E gene-specific RT–PCR and by immunocytochemistry (ICC) staining as described previously (Thontiravong et al., Citation2015; Ninvilai et al., Citation2018, Citation2020). For ICC staining, the cells were fixed with 4% paraformaldehyde in phosphate buffered saline (PBS). The fixed cells were incubated with an anti-flavivirus monoclonal antibody (clone D1-4G2-4-15, Merck Millipore, Billerica, MA) as a primary antibody followed by rabbit horseradish peroxidase (HRP)-conjugated anti-mouse IgG (Dako Cytomation, Carpinteria, CA, USA) as a secondary antibody. The cells were then stained with 3-amino-9-ethylcarbazole (AEC) (Sigma-Aldrich, St. Louis, MO, USA) and checked microscopically for the presence of virus. Virus titres were expressed as log10 50% tissue culture infectious dose (TCID50)/ml calculated by the Reed and Muench method (Reed & Muench, Citation1938). To determine the infectious titre of DTMUV in embryonated eggs, six 9-day-old embryonated chicken or duck eggs were inoculated via the allantoic cavity with 100 µl of the same 10-fold serial dilution (10−1–10−10) of DK/TH/CU-1 that was inoculated into the cell cultures. Embryos were examined twice a day for 5 days and allantoic fluid was harvested at 5 days post-inoculation or upon embryo death. DTMUV E gene-specific RT–PCR was performed to confirm the presence of DTMUV in embryonated eggs as described previously (Thontiravong et al., Citation2015). Virus titres were expressed as log10 50% embryo infectious dose (EID50)/ml calculated by the Reed and Muench method (Reed & Muench, Citation1938). Infectious doses (ID50)/ml of DTMUV in different host systems were compared to evaluate their ability to support DTMUV infection and replication (Jahangir et al., Citation2010; Moresco et al., Citation2010).
Replication kinetics of DTMUV in different avian embryonated eggs and cell cultures
The replication ability of DTMUV in different host systems was evaluated by virus replication kinetics in different avian embryonated eggs (duck and chicken) and cell cultures (primary DEF, primary CEF, BHK-21, Vero and C6/36 cells). To determine virus replication kinetics in cell cultures, monolayers of each cell culture seeded in six-well plates were inoculated in triplicate with DK/TH/CU-1 at multiplicity of infection (MOI) of 0.01 at 37°C or 28°C. After 1 h of inoculation, cell monolayers were washed and overlaid with MEM or DMEM supplemented with 10% FBS and then maintained at 37°C or 28°C. To determine virus replication kinetics in embryonated eggs, 9-day-old embryonated chicken or duck eggs were inoculated in triplicate with the same titre of DK/TH/CU-1 that was used in cell cultures (103 TCID50/ml). The eggs were then incubated at 37°C. Cell culture supernatants and allantoic fluids were harvested at 0, 6, 12, 24, 48, 72, 96 and 120 h post-inoculation (hpi). Virus yields at each time point in different host systems were determined by DTMUV E gene-specific quantitative real-time RT–PCR (qRT-PCR) as described previously (Yan et al., Citation2011; Ninvilai et al., Citation2020; Peng et al., Citation2020). Briefly, viral RNA extracted from cell culture supernatants and allantoic fluids was quantified by qRT-PCR using DTMUV E gene-specific primers and probe. The standard curve was generated from serial 10-fold dilutions of the recombinant plasmid containing the DTMUV E gene. The level of viral RNA was calculated using the linear regression equation from the standard curve (log10 copies of E gene vs cycle threshold (Ct) value) from the recombinant plasmid and expressed as log10 viral RNA copies/µl. All experiments were performed in triplicate.
Isolation of DTMUV from experimental and field clinical samples in different avian embryonated eggs and cell cultures
Isolation rates of DTMUV from experimental and field clinical samples in different host systems were compared to evaluate the efficiency of DTMUV isolation. Virus isolation was initially performed on known DTMUV-positive tissue samples (brain and spleen; n = 10) of experimentally DTMUV-infected ducks obtained from our previous study (Ninvilai et al., Citation2020). Virus isolation was also performed on 54 RT–PCR-positive tissue samples (four DTMUV cluster 1, 48 DTMUV cluster 2, and two DTMUV cluster 3) obtained from DTMUV suspected cases in Thailand between 2007 and 2017 as described previously (Thontiravong et al., Citation2015; Ninvilai et al., Citation2018, Citation2019). Briefly, tissue samples including brain, spinal cord, spleen and ovary were homogenized and then filtered through 0.45 µm filters. Tissue suspensions were subsequently inoculated into three 9-day-old embryonated duck or chicken eggs and three wells of a 24-well plate containing each cell culture (primary DEF, primary CEF, BHK-21, Vero and C6/36 cells). Inoculated embryos and cell cultures were incubated at 37°C or 28°C for up to 5 days until CPE was evident or the embryo died. Cell culture supernatants and allantoic fluids were harvested at 5 days post-inoculation or upon embryo death. The presence of DTMUV was confirmed by ICC staining (for cell culture), DTMUV E gene-specific RT–PCR and DNA sequencing (for cell culture and avian embryonated eggs) as described previously (Thontiravong et al., Citation2015; Ninvilai et al., Citation2018, Citation2019, Citation2020).
Statistical analysis
Data obtained from virus replication kinetic and virus isolation studies were expressed as mean ± standard deviation and DTMUV isolation rate (%), respectively. Differences in virus yields and DTMUV isolation rates among different host systems were evaluated by analysis of variance (ANOVA) or chi-square test, respectively, using GraphPad Prism 5.0 software (GraphPad Software Inc., La Jolla, CA, USA). All P values < 0.05 were considered statistically significant.
Ethical considerations
This study was conducted in accordance with the guidelines and approved by the Institutional Animal Care and Use Committee (IACUC) of Chulalongkorn University (Approval number 2031003).
Results
Susceptibility of different avian embryonated eggs and cell cultures to DTMUV infection
To assess which host system was the most suitable for DTMUV isolation and propagation, the infectivity of DTMUV in different host systems was first determined. DK/TH/CU-1 was titrated in various avian embryonated eggs and cell cultures. The EID50 results from avian embryonated eggs inoculated with DK/TH/CU-1 were compared to TCID50 from cell cultures inoculated with the same virus. Results showed that DK/TH/CU-1 infected all host systems tested, indicating their susceptibility to DTMUV infection ( and ). The highest infectious titre of DTMUV was obtained from primary DEF (7 log10 ID50/ml) and BHK-21 (7 log10 ID50/ml) cells, followed by embryonated duck eggs (6 log10 ID50/ml), Vero cells (5.5 log10 ID50/ml), C6/36 cells (5 log10 ID50/ml), embryonated chicken eggs (4.5 log10 ID50/ml) and primary CEF (4 log10 ID50/ml) cells ( and ). In cell cultures, the typical CPE for DTMUV infection was characterized by cell rounding and focal detachment (). CPE was observed in primary DEF, CEF and BHK-21 cells (48 hpi) earlier than in Vero and C6/36 cells (72 hpi) (). CPE observed in primary DEF and BHK-21 cells was more prominent than in other cell cultures tested (). DTMUV infection in different cell cultures and avian embryonated eggs was confirmed by ICC staining and DTMUV E gene-specific RT–PCR. All these host systems were positive for DTMUV by ICC staining (for cell cultures) and DTMUV E gene-specific RT–PCR (for cell cultures and avian embryonated eggs) (). Our results also showed that duck embryos infected with DK/TH/CU-1 died earlier (72 hpi) than chicken embryos infected with the same virus (96 hpi) (). Taken together, these results indicated that all the host systems tested, particularly primary DEF, BHK-21 cells and embryonated duck eggs, were susceptible to DTMUV infection.
Figure 1. Susceptibility of different cell cultures to DTMUV infection. The cells were infected with DTMUV at MOI of 0.01 and observed for the development of cytopathic effect (CPE) for 5 days. CPE with cell rounding and focal detachment ((b), (e), (h), (k) and (n)) and flavivirus antigen stained red brown ((c), (f), (i), (l) and (o)) were observed in DTMUV-infected cells at 72–96 h post-inoculation (hpi). Scale bar, 100 µm.
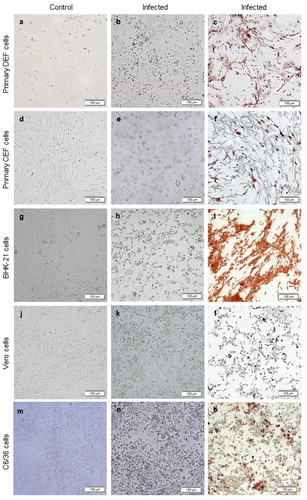
Table 1. Comparison of the infectivity of DTMUV in different avian embryonated eggs and cell cultures.
Replication kinetics of DTMUV in different avian embryonated eggs and cell cultures
To evaluate the replication kinetics of DTMUV in different host systems, various avian embryonated eggs and cell cultures were infected with DK/TH/CU-1. The allantoic fluids or cell culture supernatants were then harvested at different timepoints. In general, DK/TH/CU-1 replicated efficiently in all host systems tested with peak viral RNA levels ranging from 5.16 to 9.89 log10 copies/µl within 72–120 hpi (). BHK-21 cells had the highest ability to support the replication of DTMUV among the host systems tested, with the highest maximum viral RNA level (). Maximum viral RNA level in BHK-21 cells (9.89 log10 copies/µl) was significantly higher than in embryonated chicken eggs (8.72 log10 copies/µl), primary CEF (7.14 log10 copies/µl) and C6/36 (5.16 log10 copies/µl) cells (P < 0.05) but did not differ significantly from embryonated duck eggs (9.12 log10 copies/µl), primary DEF (9.53 log10 copies/µl) and Vero (9.83 log10 copies/µl) cells (). Our results demonstrated that DK/TH/CU-1 reached maximum viral RNA level in embryonated duck eggs and primary DEF cells within 72 hpi, earlier than in other host systems (). Collectively, these findings demonstrated that DTMUV replicated more efficiently in BHK-21 cells, albeit slightly slower than in embryonated duck eggs and primary DEF cells.
Figure 2. Replication kinetics of DTMUV in different avian embryonated eggs and cell cultures. Cells and eggs were infected with DTMUV at MOI of 0.01 or 103 TCID50/ml, respectively. The level of viral RNA was determined by qRT-PCR at the indicated timepoints. Each data point represents mean ± standard deviation (SD) of three independent experiments. Asterisks indicate the comparison of maximum viral RNA levels among different host systems tested (P < 0.05, one-way ANOVA). The numbers at data points show the maximum viral RNA level ± SD of each host system.
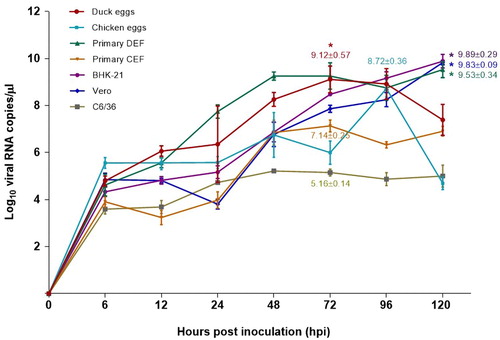
Isolation of DTMUV from duck experimental and field clinical samples in different avian embryonated eggs and cell cultures
To evaluate the efficiency of DTMUV isolation in different host systems, known DTMUV-positive tissue samples (n = 10) of experimentally DTMUV-infected ducks obtained from our previous study were first inoculated into various avian embryonated eggs and cell cultures (Ninvilai et al., Citation2020). The results showed that embryonated duck eggs, primary DEF, BHK-21, Vero and C6/36 cells gave a higher DTMUV isolation rate (100%) than embryonated chicken eggs and primary CEF cells (70%) when tested with known DTMUV-positive tissue samples (). A total of 54 RT–PCR-positive clinical samples obtained from DTMUV suspected cases in Thailand between 2007 and 2017 were also tested by virus isolation in different host systems. Of the 54 RT–PCR-positive clinical samples tested, 26/54 (48.15%), 21/54 (38.89%), 28/54 (51.85%), 25/54 (46.30%), 36/54 (66.67%), 27/54 (50.00%) and 26/54 (48.15%) were positive for DTMUV by virus isolation in embryonated duck and chicken eggs, primary DEF, primary CEF, BHK-21, Vero and C6/36 cells, respectively (). The typical CPE for DTMUV infection was observed in all cell cultures inoculated with virus isolation (VI)-positive samples. In addition, all embryos inoculated with VI-positive samples showed severe cutaneous haemorrhages and died within 3–5 days post inoculation. All VI-positive samples were also confirmed to be DTMUV-positive by ICC staining (for cell cultures) and DTMUV E gene-specific RT–PCR and DNA sequencing (for cell cultures and avian embryonated eggs) (data not shown). Among the host systems tested, BHK-21 cells had the highest DTMUV isolation rate even though the differences were not statistically significant (). Notably, although DTMUV clusters 1 and 2 were successfully recovered from all host systems with different isolation efficiencies, DTMUV cluster 3 was isolated only from BHK-21 and C6/36 cells (). Taken together, these findings indicated that BHK-21 cells were the most efficient host system for isolation of all circulating DTMUV phylogenetic clusters from duck clinical samples.
Table 2. DTMUV isolation rates from duck experimental and clinical samples in different avian embryonated eggs and cell cultures.
Discussion
Since its emergence in 2010, several phylogenetic clusters of DTMUV have been identified and caused outbreaks in Asia, resulting in significant economic losses to the duck-producing industry (Su et al., Citation2011). Early detection and control of this emerging disease require the urgent establishment of an efficient host system for isolation and propagation of all circulating phylogenetic clusters of DTMUV. Although several host systems have been used for isolation of DTMUV (Cao et al., Citation2011; Su et al., Citation2011; O'Guinn et al., Citation2013; Li et al., Citation2014; Tang et al., Citation2015; Thontiravong et al., Citation2015; Zhang et al., Citation2017), the most suitable has never been reported. In this study, various host systems, including different avian embryonated eggs and cell cultures, were evaluated and compared for their ability to support DTMUV isolation. Our results showed that all the host systems tested were susceptible to DTMUV infection, particularly primary DEF, BHK-21 cells and embryonated duck eggs. However, BHK-21 and primary DEF cells allowed more efficient replication of DTMUV compared to the other host systems tested. BHK-21 cells also had the highest DTMUV isolation rate when tested with experimental and field clinical samples. Notably, all circulating phylogenetic clusters of DTMUV were successfully recovered from BHK-21 cells. Collectively, our findings revealed that BHK-21 cells were the most efficient host system for isolation of all circulating phylogenetic clusters of DTMUV from duck clinical samples. To the best of our knowledge, this is the first report on the evaluation of host systems for efficient isolation and propagation of DTMUV.
In this study, all the host systems tested were found to be susceptible to DTMUV infection, consistent with previous studies showing successful isolation of DTMUV using these host systems (Cao et al., Citation2011; Su et al., Citation2011; O'Guinn et al., Citation2013; Li et al., Citation2014; Tang et al., Citation2015; Thontiravong et al., Citation2015; Zhang et al., Citation2017). However, DTMUV yielded higher titres in primary DEF, BHK-21 cells and embryonated duck eggs than in the other host systems tested. This corresponds with recent studies reporting the presence of DTMUV-specific receptors in primary DEF and BHK-21 cells (Zhao et al., Citation2018; Wu et al., Citation2019). In addition, this finding indicates that host systems derived from the same host species from which the virus was isolated displayed high susceptibility to infection (Li et al., Citation2015). Our results demonstrated that CPE was observed in primary DEF, CEF and BHK-21 cells within 48 hpi, earlier than that from the previous studies showing the presence of CPE of Chinese TMUV in BHK-21 cells after 72 hpi (Cao et al., Citation2011; Lei et al., Citation2017). DK/TH/CU-1 replicated efficiently in all host systems tested, corresponding to previous studies reporting the ability of mosquito-borne flaviviruses, including DTMUV, to infect and replicate in a variety of cell lines (Blitvich & Firth, Citation2015; Wang et al., Citation2016). However, among the host systems tested, DK/TH/CU-1 reached the highest maximum viral RNA level in BHK-21 cells, indicating the preference of DTMUV to grow in BHK-21 cells. This finding is consistent with a previous study reporting that Chinese DTMUV reached the highest peak titre in BHK-21 cells compared to other cell cultures tested, including Vero cells and CEF cells (Wang et al., Citation2016). This suggested that DTMUV replicated more efficiently in BHK-21 cells, even though it grew slightly slower than in embryonated duck eggs and primary DEF cells. In addition, CPEs observed in BHK-21 and primary DEF cells were easily detected when compared to other cell cultures, indicating the advantage of using these cell cultures for DTMUV isolation. Taken together, these findings suggested that primary DEF, BHK-21 cells and embryonated duck eggs were highly susceptible to DTMUV infection and allowed DTMUV replication at high efficiency.
Corresponding to the findings from virus infectivity and replication kinetic studies, the isolation rate of DTMUV from duck clinical samples in BHK-21 cells was higher than in the other host systems. Notably, although the number of DTMUV cluster 1 and 3 positive tissue samples evaluated in this study was relatively small due to limited outbreaks associated with DTMUV clusters 1 and 3 in ducks (Ninvilai et al., Citation2018, Citation2019), all circulating phylogenetic clusters of DTMUV were successfully recovered from BHK-21 cells. These findings suggested that BHK-21 cells were the most efficient host system for isolation of all DTMUV phylogenetic clusters from duck clinical samples. This highlights the importance of selecting suitable host systems for isolation of different phylogenetic clusters of DTMUV. BHK-21 is a widely used cell line, which has several advantages over embryonated eggs and primary cell cultures for virus isolation; it is convenient, less time-consuming, allows high-volume sample processing and is cost-effective (Leland & Ginocchio, Citation2007; Zhang et al., Citation2017). Low isolation rates of DTMUV from both experimental and clinical samples were observed in primary CEF cells and embryonated chicken eggs, indicating that these chicken-derived host systems had relatively low sensitivity for isolation of DTMUV from duck clinical samples. However, these findings might not hold true for all strains of DTMUV circulating in other host species. Additional studies are required to evaluate host systems for efficient isolation of other strains of DTMUV. Furthermore, although BHK-21 cells showed better results for isolation of DTMUV than the other host systems, the use of alternative host systems, especially primary DEF cells, should be considered if BHK-21 cells fail to isolate DTMUV from RT–PCR-positive samples.
In conclusion, our data collectively support the use of BHK-21 cells as a host system for the primary isolation of all circulating phylogenetic clusters of DTMUV from duck clinical samples. These findings will be useful for DTMUV research and possibly also for vaccine production. This study highlights the importance of selecting the most appropriate host system for efficient isolation and propagation of DTMUV from duck clinical samples. However, regarding the rapid evolution of DTMUV (Dai et al., Citation2015; Ninvilai et al., Citation2019), continued validation of the virus isolation protocol with recent DTMUV strains is required to ensure optimal sensitivity and accuracy of the results.
Acknowledgements
We would like to acknowledge Assoc. Prof. Sonthaya Tiawsirisup and Assoc. Prof. Padet Siriyasatien for their contribution of C6/36 cells. We would also like to thank Chulalongkorn University for its financial support to the Center of Excellence for Emerging and Re-emerging Infectious Diseases in Animals, the Animal Vector-Borne Disease Research Unit, Chulalongkorn Academic Advancement Into Its 2nd Century Project and the Animal Virome and Diagnostic Development Research Group, and the Thailand Research Fund for its financial support to the TRF Senior Scholar to AA (RTA6080012).
Disclosure statement
No potential conflict of interest was reported by the authors.
Additional information
Funding
References
- Blitvich, B.J. & Firth, A.E. (2015). Insect-specific flaviviruses: a systematic review of their discovery, host range, mode of transmission, superinfection exclusion potential and genomic organization. Viruses, 7, 1927–1959.
- Cao, Z., Zhang, C., Liu, Y., Ye, W., Han, J., Ma, G., Zhang, D., Xu, F., Gao, X. & Tang, Y. (2011). Tembusu virus in ducks, China. Emerging Infectious Diseases, 17, 1873.
- Dai, L., Li, Z. & Tao, P. (2015). Evolutionary analysis of Tembusu virus: evidence for the emergence of a dominant genotype. Infection, Genetics and Evolution, 32, 124–129.
- Homonnay, Z.G., Kovács, E.W., Bányai, K., Albert, M., Fehér, E., Mató, T., Tatár-Kis, T. & Palya, V. (2014). Tembusu-like flavivirus (Perak virus) as the cause of neurological disease outbreaks in young Pekin ducks. Avian Pathology, 43, 552–560.
- Jahangir, A., Ruenphet, S., Hara, K., Shoham, D., Sultana, N., Okamura, M., Nakamura, M. & Takehara, K. (2010). Evaluation of human intestinal epithelial differentiated cells (Caco-2) for replication, plaque formation and isolation of avian influenza viruses. Journal of Virological Methods, 169, 232–238.
- Lei, W., Guo, X., Fu, S., Feng, Y., Tao, X., Gao, X., Song, J., Yang, Z., Zhou, H. & Liang, G. (2017). The genetic characteristics and evolution of Tembusu virus. Veterinary Microbiology, 201, 32–41.
- Leland, D.S. & Ginocchio, C.C. (2007). Role of cell culture for virus detection in the age of technology. Clinical Microbiology Reviews, 20, 49–78.
- Li, G., Gao, X., Xiao, Y., Liu, S., Peng, S., Li, X., Shi, Y., Zhang, Y., Yu, L. & Wu, X. (2014). Development of a live attenuated vaccine candidate against duck Tembusu viral disease. Virology, 450-451, 233–242.
- Li, N., Wang, Y., Li, R., Liu, J., Zhang, J., Cai, Y., Liu, S., Chai, T. & Wei, L. (2015). Immune responses of ducks infected with duck Tembusu virus. Frontiers in Microbiology, 6, 1–7.
- Moresco, K.A., Stallknecht, D.E. & Swayne, D.E. (2010). Evaluation and attempted optimization of avian embryos and cell culture methods for efficient isolation and propagation of low pathogenicity avian influenza viruses. Avian Diseases, 54, 622–626.
- Ninvilai, P., Limcharoen, B., Tunterak, W., Prakairungnamthip, D., Oraveerakul, K., Banlunara, W. & Thontiravong, A. (2020). Pathogenesis of Thai duck Tembusu virus in Cherry Valley ducks: the effect of age on susceptibility to infection. Veterinary Microbiology, 243, 108636.
- Ninvilai, P., Nonthabenjawan, N., Limcharoen, B., Tunterak, W., Oraveerakul, K., Banlunara, W., Amonsin, A. & Thontiravong, A. (2018). The presence of duck Tembusu virus in Thailand since 2007: a retrospective study. Transboundary and Emerging Diseases, 65, 1208–1216.
- Ninvilai, P., Tunterak, W., Oraveerakul, K., Amonsin, A. & Thontiravong, A. (2019). Genetic characterization of duck Tembusu virus in Thailand, 2015–2017: identification of a novel cluster. Transboundary and Emerging Diseases, 66, 1982–1992.
- O'Guinn, M.L., Turell, M.J., Kengluecha, A., Jaichapor, B., Kankaew, P., Miller, R.S., Endy, T.P., Jones, J.W., Coleman, R.E. & Lee, J.S. (2013). Field detection of Tembusu virus in western Thailand by rt-PCR and vector competence determination of select culex mosquitoes for transmission of the virus. American Journal of Tropical Medicine and Hygiene, 89, 1023–1028.
- Peng, S.H., Su, C.L., Chang, M.C., Hu, H.C., Yang, S.L. & Shu, P.Y. (2020). Genome analysis of a novel Tembusu virus in Taiwan. Viruses, 12, 1–19.
- Reed, L.J. & Muench, H. (1938). A simple method of estimating fifty percent endpoints. American Journal of Epidemiology, 27, 493–497.
- Su, J., Li, S., Hu, X., Yu, X., Wang, Y., Liu, P., Lu, X., Zhang, G., Hu, X. & Liu, D. (2011). Duck egg-drop syndrome caused by BYD virus, a new Tembusu-related flavivirus. PLoS ONE, 6, e18106.
- Tang, Y., Diao, Y., Chen, H., Ou, Q., Liu, X., Gao, X., Yu, C. & Wang, L. (2015). Isolation and genetic characterization of a Tembusu virus strain isolated from mosquitoes in Shandong, China. Transboundary and Emerging Diseases, 62, 209–216.
- Thontiravong, A., Ninvilai, P., Tunterak, W., Nonthabenjawan, N., Chaiyavong, S., Angkabkingkaew, K., Mungkundar, C., Phuengpho, W., Oraveerakul, K. & Amonsin, A. (2015). Tembusu-related flavivirus in ducks, Thailand. Emerging Infectious Diseases, 21, 2164–2167.
- Wang, H.-J., Li, X.-F., Liu, L., Xu, Y.-P., Ye, Q., Deng, Y.-Q., Huang, X.-Y., Zhao, H., Qin, E.-D. & Shi, P.-Y. (2016). The emerging duck flavivirus is not pathogenic for primates and is highly sensitive to mammalian interferon antiviral signaling. Journal of Virology, 90, 6538–6548.
- Wu, S., Wu, Z., Wu, Y., Wang, T., Wang, M., Jia, R., Zhu, D., Liu, M., Zhao, X., Yang, Q., Wu, Y., Zhang, S., Liu, Y., Zhang, L., Yu, Y., Pan, L., Chen, S. & Cheng, A. (2019). Heparin sulfate is the attachment factor of duck Tembus virus on both BHK21 and DEF cells. Virology Journal, 16, 134.
- Yan, L., Yan, P., Zhou, J., Teng, Q. & Li, Z. (2011). Establishing a TaqMan-based real-time PCR assay for the rapid detection and quantification of the newly emerged duck Tembusu virus. Virology Journal, 8, 464.
- Zhang, L., Li, Z., Zhang, Q., Sun, M., Li, S., Su, W., Hu, X., He, W. & Su, J. (2017). Efficacy assessment of an inactivated Tembusu virus vaccine candidate in ducks. Research in Veterinary Science, 110, 72–78.
- Zhang, L., Sun, M., Zhang, Q., Wang, J., Cao, Y., Cui, S. & Su, J. (2020). Long-term passage of duck Tembusu virus in BHK-21 cells generates a completely attenuated and immunogenic population with increased genetic diversity. Vaccine, 38, 933–941.
- Zhao, D., Han, K., Zhang, L., Wang, H., Tian, Y., Huang, X., Liu, Q., Yang, J., Liu, Y. & Li, Y. (2018). Identification and immunogenic evaluation of T cell epitopes based on Tembusu virus envelope protein in ducks. Virus Research, 257, 74–81.