ABSTRACT
Avian metapneumovirus (aMPV) causes respiratory disease and drops in egg production in chickens, and is routinely controlled by vaccination. However, the host’s immune response to virulent challenge in vaccinated or unvaccinated broiler chickens is poorly characterized. We show that subtype B vaccination offers heterologous (subtype A challenge) and homologous (subtype B challenge) protection. Subtype B challenge caused significantly greater humoral antibody titres in vaccinated and unvaccinated chickens. In turbinate and lung tissues of unvaccinated-challenged chickens, IgA and IgY mRNA transcription was significantly up-regulated after subtype B challenge compared to subtype A. Cellular immunity (CD8-α and CD8-β) gene transcripts were significantly up-regulated during early and later stages of infection from subtype B or subtype A, respectively. Immune gene transcriptional responses (IL-1β, IL-6 and IL-18) were significantly up-regulated after challenge. Gene transcription results showed that mRNA expression levels of CD8-α, CD8-β, TLR3 and IL-6, particularly in turbinate and trachea tissues, are useful parameters to include in future aMPV vaccination-challenge studies.
Introduction
Poultry disease caused by avian metapneumovirus (aMPV) was first described in 1978, and the virus was subsequently isolated and identified in 1986 (Buys, Citation1980; McDougall & Cook, Citation1986). To date, four subtypes have been identified (A, B, C and D) based on both antigenic differences and variations in their genome (Juhasz & Easton, Citation1994; Cook et al., Citation1999; Bäyon-Auboyer et al., Citation2000). However, a recent publication researching wild birds has highlighted the existence of intermediate or novel aMPVs, which do not belong to these four subtypes (Canuti et al., Citation2019). While turkeys are more susceptible to aMPV (Cook, Citation2000; Hartmann et al., Citation2015), infection in chickens remains an issue for producers globally. In the UK, infection in chickens predominantly originates from subtype B (Jones, Citation2010; Ball et al., Citation2018); however, subtype A continues to be globally detected (Chacón et al., Citation2011; Abdel-Azeem et al., Citation2014). Infection from subtype A or B is mostly associated with swollen head syndrome, which produces signs such as periorbital and infraorbital sinus swelling and nasal discharge (Cook, Citation2000) in broiler, layer and breeder chickens. Strains belonging to both subtype A and B have been shown to cause drops in egg production and quality (Hess et al., Citation2004; Sugiyama et al., Citation2006). Disease severity and production losses due to aMPV are controlled by the use of live attenuated vaccines (Marangon & Busani, Citation2007). In turkeys, a degree of cross-protection conferred by subtype A and B vaccines has been demonstrated against heterologous challenge from either subtype A or B (Cook et al., Citation1995; Cook et al., Citation1999; Toquin et al., Citation1999). However, there is limited published information on cross-protection between the subtypes in chickens (Cook et al., Citation1995). A few aMPV strains of turkey origin have been reported not to cause disease in chickens (Gough et al., Citation1988). However, publications from our laboratory and others have demonstrated that both subtype A and subtype B, including the virulent strains used in this paper, are capable of causing disease and an immune response in chickens (Elhafi et al., Citation2004; Htut Aung et al., Citation2008).
Laboratory diagnosis of aMPV has included virus isolation using tracheal organ cultures (McDougall & Cook, Citation1986) or embryonated eggs (Chacón et al., Citation2007; Sun et al., Citation2014), and identification by immunofluorescence (Luo et al., Citation2009) and reverse transcription PCR (RT-PCR) (Cavanagh et al., Citation1999). Multiplex real-time RT-PCR assays have also been developed to allow for differentiation between subtypes A and B in a single reaction (Guionie et al., Citation2007). Along with antigen detection, the host response is another method to diagnose aMPV infection. For antibody detection, a number of commercial ELISA kits are widely available, and specific IgA-ELISA assays have been attempted before (Rautenschlein et al., Citation2011; Śmiałek et al., Citation2016). With the advancement of molecular techniques, a number of studies have reported host (chicken)-agent interactions, including innate and cellular gene transcripts following respiratory virus infection. These include infectious bronchitis virus (IBV) (Kameka et al., Citation2014; Okino et al., Citation2017; Chhabra et al., Citation2018), Newcastle disease virus (NDV) (Schilling et al., Citation2019) and aMPV (Cha et al., Citation2011; Yun et al., Citation2016). For aMPV, interferon gamma (IFN-γ) transcription is up-regulated following infection of turkeys (Cha et al., Citation2011). In chickens, up-regulation of IFN-γ is stimulated by the presence of interleukin 18 (IL-18) (Göbel et al., Citation2003). Activity of the cleavage protease TMPRSS12 was shown to be crucial for aMPV subtype B infectivity in chickens (Yun et al., Citation2016), as it facilitates the cleavage of the aMPV fusion (F) protein, which mediates virion fusion to the host cell (Yun et al., Citation2016). There are currently no previous reports on IL-18 detection in relation to aMPV infection in chickens.
The objectives of this study were to investigate the following: (i) cross-protection conferred by subtype B vaccination against subtype A challenge in 21-days-old broiler chickens, (ii) profiling the immune response in unvaccinated 21-days-old chickens challenged with either virulent subtype A or subtype B strains, and (iii) profiling the immune response of aMPV-vaccinated chickens when challenged with either virulent subtype A or subtype B.
Materials and methods
Chicks
Day-old broiler chicks with no previous vaccinations were obtained from a commercial hatchery. The parent stock had been vaccinated against aMPV. All birds were kept in separate, secure, negative-pressure rooms (University of Liverpool) and reared on deep litter with water and feed provided ad libitum. No antibiotics were included in feed or water throughout the experiment. All experimental procedures were conducted according to UK legislation on the use of animals for experiments, as permitted under the project license PPL 112 40/3723.
Avian metapneumoviruses
Vaccine strain
A commercially available live attenuated aMPV subtype B vaccine (Nemovac®, Boehringer Ingelheim, Lyon, France) was prepared using sterile distilled water, prior to administration. Each chick received 100 µl (>103.0 TCID50/dose) as per the manufacturer’s instructions.
Challenge strains
A subtype A (105.0 TCID50/ml) (Elhafi et al., Citation2004) and a subtype B (104.51 TCID50/ml) (Ganapathy & Jones, Citation2007) virulent aMPV strain were used as challenge virus. Viral titres were confirmed using tracheal organ cultures (TOCs) (Cook et al., Citation1976). Bacteria/mycoplasma culture confirmed the inoculum to be free of bacteria and mycoplasmas (Moscoso et al., Citation2004).
Experimental design
A total of 180 day-old broiler chicks were separated into two groups (90 per group) and kept in separate negative-pressure rooms. At day-old, chicks from Group 1 were inoculated via ocular (50 µl) and nasal (50 µl) routes with the aMPV subtype B vaccine. Chicks from Group 2 were sham-inoculated with sterile distilled water. Birds were observed daily for clinical signs by the lead researchers. At 1, 3, 5, 7, 10, 14 and 21 days post-vaccination (dpv), oropharyngeal (OP) and cloacal (CL) swabs were collected from ten randomly sampled chicks. At 7, 14 and 21 dpv, five randomly sampled chicks were euthanised from each group. Turbinate, trachea (upper) and lung tissue were individually collected from each chick, with one portion immediately homogenized for RT-PCR, and a separate portion stored in RNAlater® (Qiagen, Germantown, MD, USA) for quantitative RT-PCR (qRT-PCR). From all groups, blood was collected from ten chicks before vaccination and at 21 dpv.
After sampling at 21 dpv, chicks from Group 1 (n = 75) were split into Groups A1, B1 and C1, and chicks from Group 2 (n = 75) were split into Groups A2, B2 and C2, with 25 chicks per group. Chicks in Groups A1 and A2 were challenged with 100 µl of virulent aMPV subtype A via oculo-nasal route. Similarly, chicks in Groups B1 and B2 were challenged with 100 µl of virulent aMPV B. Groups C1 and C2 were sham-challenged with sterile TOC medium. Swabs (OP and CL) were collected from five chicks at 3, 6 and 9 days post-challenge (dpc). At the same time-points, five chicks were euthanised, and turbinate, trachea and lung samples were collected from each group (as previously described) and stored in RNAlater®. Blood was collected from ten chicks via the brachial vein at 10 dpc.
Clinical signs
Chicks were observed daily for clinical signs (Naylor et al., Citation1992); 0 = no clinical signs; 1 = clear, unilateral nasal exudate; 2 = turbid, bi-lateral nasal exudate; 3 = nasal exudate with frothy eyes and/or swollen infra-orbital sinus. Birds exceeding the moderate severity limit were immediately euthanised and removed from the study as per the project license.
Measurement of antibody levels
Blood was taken from ten chicks at 0 and 21 dpv, and 10 dpc. A commercial ELISA kit (BioChek BV, Reeuwijk, Netherlands) was used for the detection of aMPV antibodies. Samples with a sample positive (S/P) ratio of over 0.2 were considered positive. Antibody titres were calculated according to the manufacturer’s instructions, with results higher than 1655 indicating positive samples.
aMPV detection by RT-PCR
Oropharyngeal (OP) and cloacal (CL) swabs were collected from ten chicks per group. At each sampling point, swabs were pooled and dipped as a single sample per group in a sterile bijou tube containing 1.5 ml of Eagle's serum-free minimum essential medium with glutamine, streptomycin (50 mg/ml) and penicillin (50 IU/ml), and stored at −70°C until required.
At 7, 14, 21 dpv five trachea, turbinate and lung samples were collected from each group. After challenge, the same sampling occurred at 3, 6 and 9 dpc. Collected tissue samples from each sampled chick were individually ground with sterile sand and 1.5 ml of TOC medium (minimum essential medium, sodium bicarbonate and 0.4% penicillin/streptomycin) using a pestle and mortar. Homogenates were frozen and thawed three times, then clarified by centrifugation at 3000 × g for 15 min. Supernatants were stored at −70°C.
RNA was extracted from both the swab and tissue samples using the phenol chloroform method (Chomczynski & Sacchi, Citation2006) and subjected to RT-PCR for aMPV (Cavanagh et al., Citation1999). Samples were determined as subtype A (268 bp) or subtype B (361 bp) positive based on amplicon size.
Host gene mRNA expression following infection
Extracted RNA from each individual sample was converted to cDNA (Chhabra et al., Citation2018) and analysed by quantitative real-time PCR for mRNA transcript expression of immunoglobulin A (IgA), immunoglobulin Y (IgY), CD8-α, CD8-β, toll-like receptor 3 (TLR3), interferon gamma (IFN-γ), interleukins (IL-1β, IL-6 and IL-18) and TMPRSS12 (Zheng et al., Citation2001; Cha et al., Citation2011; Ndegwa et al., Citation2012; Kuchipudi et al., Citation2014; Yun et al., Citation2016; Okino et al., Citation2017; Chhabra et al., Citation2018). Each individual cDNA sample was tested in triplicate using the LightCycler 480 SYBR Green I Master mix (Roche Diagnostics, Risch-Rotkreuz, Switzerland) and gene specific primers. Data were normalized against the 18S housekeeping gene. The fold change difference in gene expression compared to the control group was calculated using the double delta method (Livak & Schmittgen, Citation2001). Samples with a double delta Ct (ΔΔ Ct) value ≥ 2 or ≤ 0.5 were considered biologically relevant (Zegpi et al., Citation2020).
Statistical analysis
Statistical analysis was carried out using SPSS 25 (IBM, New York, NY, USA). Significance of differences between groups was analysed using univariate ANOVA, alongside the homogeneity of variance test, to confirm statistical differences within the data set. This was followed by post hoc Tukey’s testing to compare between each group. When groups had P < 0.05 for the homogeneity of variance test, Tamhane’s T2 was applied post hoc instead of Tukey’s. All differences between treatment and/or control groups were considered significant at P < 0.05 unless stated.
Results
Clinical signs
No clinical signs were detected after vaccination. Following challenge, mild clinical signs were found in both unvaccinated groups (A2 and B2) comprising nasal exudate. While not significant (P > 0.05), subtype B showed a greater severity of clinical signs compared to subtype A at the peak of presentation. The signs associated with subtype B persisted until 8 dpc, whereas clinical signs associated with subtype A challenge were only present until 6 dpc (). No clinical signs were found in other groups. No birds exceeded the moderate severity limit, and there were no mortalities during the study.
Evaluation of aMPV ELISA antibody titres at 10 dpc
Prior to vaccination, the mean serum aMPV antibody titre at day-old was 9579.18 ± 2341, indicating the presence of aMPV-specific maternally-derived antibodies (MDA). At 21 days old, antibody levels in the unvaccinated chicks had declined (). In contrast, chicks that were vaccinated at day-old, showed a positive aMPV antibody titre of 3074 ± 770. By 10 dpc, only Group C3 (unvaccinated-unchallenged) had an average antibody titre below the detectable limit (329 ± 63) while all other groups had a positive titre. Following aMPV challenge, subtype B induced a significantly greater antibody response in the unvaccinated (16,681 ± 1839) group when compared to subtype A (3697 ± 277). Subtype B challenge also induced a greater response in unvaccinated birds (16,681 ± 1839) compared to the vaccinated birds (12,734 ± 1967). In contrast, with subtype A challenge, higher antibody titre (9847 ± 1786) was found in vaccinated-challenged birds compared with the unvaccinated-challenged birds (3697 ± 277).
Figure 2. Serum ELISA results for all groups at 21 dpv (i.e. 0 dpc) and 10 dpc. Data are presented as the mean antibody titre of 10 birds per group ± SEM, according to the formula provided by the manufacturer. Values over 1655 indicate a positive antibody titre against aMPV (dotted line). Significant differences between groups at the same time-point are indicated by different letters.
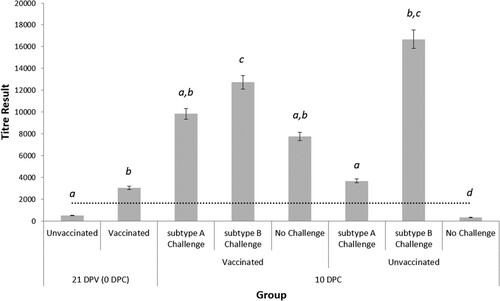
aMPV RNA detection by RT-PCR
Post-vaccination
Subtype B RNA was detected in OP swabs at 1–14 dpv in the vaccinated group. Cloacal swabs were negative at all sampling points, and all swabs from the unvaccinated group were negative. For the tissues, only turbinate samples were positive at 21 dpv from the aMPV-vaccinated group. All trachea and lung samples were negative for aMPV.
Post-challenge swabs
Following subtype A challenge, subtype A viral RNA was detected in OP swabs from both challenged groups (). The vaccinated group was only positive at 3 dpc, with OP swabs positive at both 3 and 6 dpc in the unvaccinated group. Following subtype B challenge, the virulent subtype B strain was detected in OP swabs from the unvaccinated group at 3 and 6 dpc, with all swabs negative in the vaccinated group. The unchallenged groups were aMPV-negative throughout the study.
Table 1. aMPV strain detection by RT-PCR from OP swabs, turbinate, trachea and lung tissue taken at 3, 6 and 9 dpc. (–) denotes a negative sample. Cloacal swabs and unchallenged groups were all negative and are not shown.
Post-challenge tissues
Following subtype A challenge, subtype B RNA was detected in the vaccinated group until 6 dpc from turbinate samples, with subtype A detected at 9 dpc. Both trachea and lung tissues were negative by RT-PCR. For the unvaccinated group, virulent subtype A RNA was detected in the turbinate, trachea and lung at 3 dpc, the trachea and turbinate at 6 dpc, and only the turbinate by 9 dpc.
After subtype B challenge, turbinate and trachea samples in the vaccinated group were subtype B virus positive until 9 and 3 dpc, respectively. All lung samples from vaccinated birds were negative. For the unvaccinated group, aMPV subtype B RNA was detected in all tissues up to 9 dpc. Unchallenged groups remained negative throughout the study.
Mucosal immune responses: IgA and IgY
Turbinate
Challenge from either subtype caused a significant IgA down-regulation in unvaccinated chicks at 6 and 9 dpc; however, subtype B induced a significant up-regulation at 3 dpc. In the vaccinated group, subtype A challenge significantly increased IgA transcription at 3 dpc, whereas subtype B caused a significant down-regulation at all time-points. For IgY, subtype A challenge of unvaccinated chicks caused significantly down-regulated transcription at 3 and 9 dpc (). For vaccinated birds, IgY was significantly up-regulated at the same time-points. After subtype B challenge, IgY was significantly up-regulated at 6 and 9 dpc in unvaccinated birds, whereas no change was noted in the vaccinated group.
Figure 3. Expression profile of IgA and IgY genes in turbinate, trachea and lung tissues in unvaccinated or vaccinated broiler chickens challenged with either subtype A or subtype B aMPV. Data are shown as fold change when compared to the unvaccinated-unchallenged (control) group. Significant differences in fold change between groups are shown with different letters. Samples with a fold change value ≥ 2 or ≤ 0.5 were considered as biologically relevant.
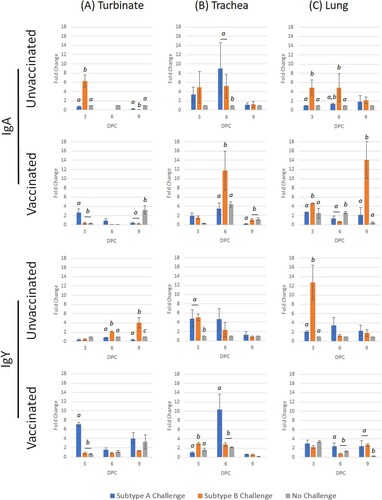
Trachea
For IgA, challenge by either subtype caused significant up-regulation at 3 and 6 dpc in unvaccinated chicks. The only significant up-regulation was seen in subtype B-challenged birds at 6 dpc. After challenge by either subtype A or B, IgY transcription was up-regulated at 3 and 6 dpc in unvaccinated groups (). Only subtype A caused a significant up-regulation in vaccinated birds at 6 dpc.
Lung
Subtype A challenge stimulated an up-regulation of IgA at 3 and 6 dpc, whereas subtype B produced a significant response at all time-points. In the vaccinated birds, subtype B generated a significant up-regulation at 3 dpc, and both virulent subtypes induced significant transcription at 9 dpc. For IgY, subtype A challenge caused a significant up-regulation at all time-points in unvaccinated chicks, whereas subtype B only induced up-regulation at 3 and 6 dpc (). Both subtypes induced a significant up-regulation at 9 dpc in the vaccinated groups.
Cell-mediated immune responses: mRNA expression of CD8-α and CD8-β
Turbinate
Challenge of unvaccinated birds with subtype A stimulated significant transcription of CD8-α at all time-points; however, subtype B only induced a significant up-regulation at 3 dpc, which was then down-regulated by 9 dpc (). In vaccinated birds, no significant differences were noted when compared with the unchallenged group. For CD8-β, subtype A caused significant up-regulation at 3 dpc in vaccinated chicks, whereas transcription was up-regulated at 3 and 6 dpc for subtype B. Significant up-regulation for both subtypes only occurred at 3 dpc in vaccinated birds.
Figure 4. Expression profile of CD8-α and CD8-β genes in turbinate, trachea and lung tissues in unvaccinated or vaccinated broiler chickens challenged with either subtype A or subtype B aMPV. Data are shown as fold change when compared to the unvaccinated-unchallenged (control) group. Significant differences in fold change between groups are shown with different letters. Samples with a fold change value ≥ 2 or ≤ 0.5 were considered as biologically relevant.
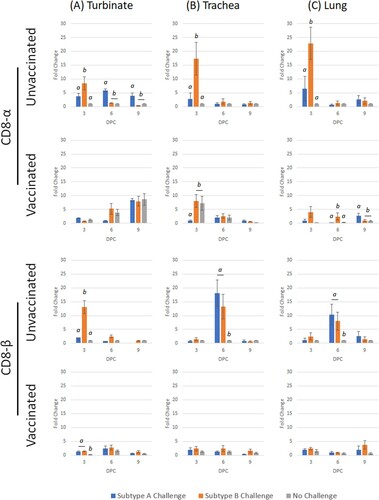
Trachea
In unvaccinated chicks, CD8-α was only significantly up-regulated at 3 dpc for both subtypes (). In contrast, subtype A challenge caused a significant down-regulation in the vaccinated birds at 3 dpc. No other changes were noted. For CD8-β, both subtypes induced significant transcription at 3 and 6 dpc in unvaccinated birds, whereas no change was noted for vaccinated groups.
Lung
Transcription of CD8-α was up-regulated at 3 dpc for both virulent subtypes in unvaccinated chicks, whereas only subtype B stimulated significant transcription at 3 and 6 dpc in vaccinated birds (). Similar to the trachea, both subtypes induced significant CD8-β transcription at 3 and 6 dpc in unvaccinated groups. Subtype B challenge caused significant up-regulation at 9 dpc, with no other changes identified.
Post-aMPV challenge (subtype A or subtype B) host gene expression in unvaccinated chicks
Following subtype A or subtype B virulent aMPV challenge in 21-days-old commercial broiler chicks, the various host gene transcripts in turbinate, trachea and lung tissues were examined at intervals.
Turbinate
Only TLR3, IFN-γ and IL-18 were significantly up-regulated following subtype A challenge ( and ), of which IFN-γ and IL-18 were identified to have biological relevance (> 2 fold change). After subtype B challenge, TLR3, IFN-γ and IL-18 were significantly up-regulated with biological relevance. When compared with subtype A challenge, subtype B challenge induced significantly higher transcription of TLR3, IFN-γ, IL-6 and IL-18 up to 6 dpc.
Figure 5. Expression profile of host genes (TLR3, TMPRSS12 and IFN-γ) in trachea, turbinate and lung tissues in unvaccinated broiler chickens challenged with either subtype A or subtype B aMPV. Data are shown as fold change when compared to the unvaccinated-unchallenged (control) group. Significant differences in fold change between groups are shown with different letters. Samples with a fold change value ≥ 2 or ≤ 0.5 were considered as biologically relevant.
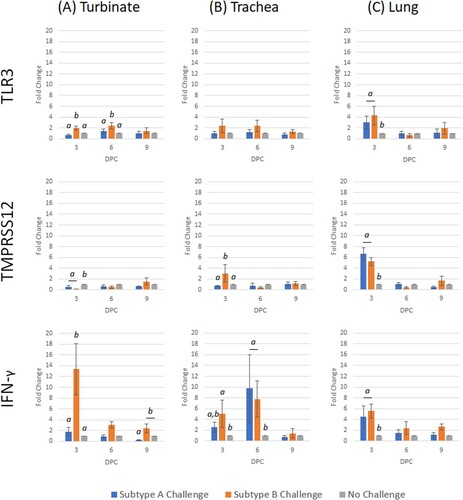
Figure 6. Expression profile of interleukin host genes (IL-1β, IL-6 and IL-18) in trachea, turbinate and lung tissues in unvaccinated broiler chickens challenged with either subtype A or subtype B aMPV. Data are shown as fold change when compared to the unvaccinated-unchallenged (control) group. Significant differences in fold change between groups are shown with different letters. Samples with a fold change value ≥ 2 or ≤ 0.5 were considered as biologically relevant.
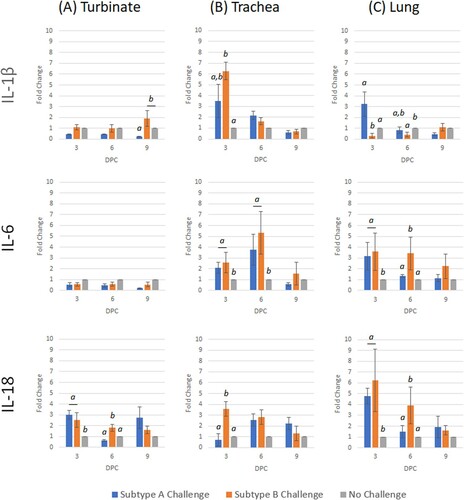
Trachea
Only IL-6 at 3 dpc was significantly up-regulated after subtype A challenge (). Similar to the turbinate, all genes were significantly up-regulated at either 3 or 6 dpc, following subtype B challenge, with the exception of TLR3 ( and ).
Lung
After subtype A challenge, TLR3 and TMPRSS12 were the only genes to be significantly up-regulated (). For the subtype B challenge, TLR3, TMPRSS12, IL-6 and IL-18 were significantly up-regulated, whereas IL-1β was down-regulated at 3 dpc ( and ). By 6 dpc, only IL-6 and IL-18 were up-regulated, while TMPRSS12 and IL-1β were down-regulated. Comparing the two challenge groups, TMPRSS12 and IL-1β were transcribed to a significantly greater level in subtype A compared to B, whereas IL-6 and IL-18 were significantly lower.
Post-aMPV challenge (subtype A or subtype B) host gene expression in subtype-B vaccinated chicks
Chicks that were previously vaccinated with subtype B aMPV at day-old were challenged with either virulent subtype A or subtype B at 21 days of age. Host gene transcripts in turbinate, trachea and lung tissues were evaluated at intervals.
Turbinate
After subtype A challenge, IL-1β was down-regulated at 9 dpc (). The only genes with significant up-regulation after subtype B challenge were IFN-γ (3 dpc) and TMPRSS12 (9 dpc) (). After comparing both challenge groups, TMPRSS12 and IL-1β were significantly lower in subtype A-challenged birds compared to subtype B-challenged birds.
Trachea
The only gene to be up-regulated after subtype A challenge was TMPRSS12 at 9 dpc (). Early responses (3–6 dpc) following subtype B challenge demonstrated an up-regulation of IFN-γ, TMPRSS12 and IL-18 ( and ). Early transcription responses of TMPRSS12 and IL-18 were significantly lower after subtype A challenge when compared to the subtype B-challenged birds.
Figure 7. Expression profile of host genes (TLR3, TMPRSS12 and IFN-γ) in trachea, turbinate and lung tissues in vaccinated broiler chickens challenged with either subtype A or subtype B aMPV. Data are shown as fold change when compared to the unvaccinated-unchallenged (control) group. Significant differences in fold change between groups are shown with different letters. Samples with a fold change value ≥ 2 or ≤ 0.5 were considered as biologically relevant.
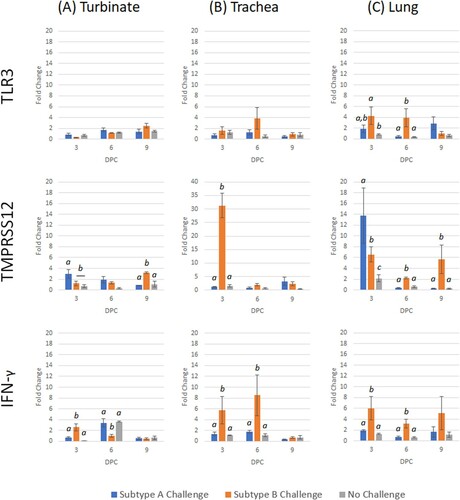
Figure 8. Expression profile of interleukin host genes (IL-1β, IL-6 and IL-18) in trachea, turbinate and lung tissues in vaccinated broiler chickens challenged with either subtype A or subtype B aMPV. Data are shown as fold change when compared to the unvaccinated-unchallenged (control) group. Significant differences in fold change between groups are shown with different letters. Samples with a fold change value ≥ 2 or ≤ 0.5 were considered as biologically relevant.
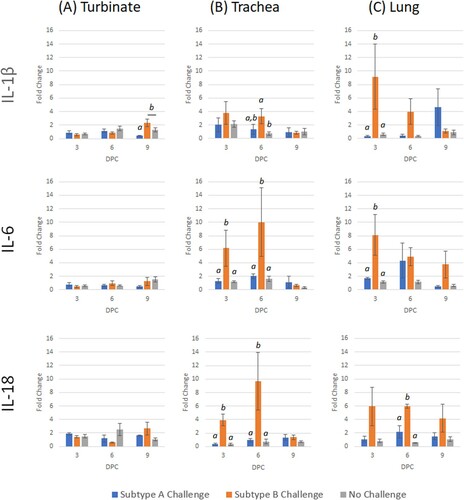
Lung
Up-regulation was demonstrated for TMPRSS12 (3 dpc) and TLRs (both 9 dpc) following subtype A challenge ( and ). Interferon-γ and TMPRSS12 were both down-regulated at 6 dpc. For subtype B challenge, all genes were up-regulated on at least one time-point, with the exception of IL-1β. Transcription of TLR3, IFN-γ, TMPRSS12 and IL-18 were significantly lower for subtype A when compared with subtype B.
Discussion
In this study, three aspects of aMPV in commercial broiler chickens were examined; (i) cross-protection conferred by subtype B vaccination against subtype A challenge, (ii) profiling the immune response in unvaccinated 21-days-old chickens challenged with either virulent subtype A or subtype B strains, (iii) profiling the immune response of aMPV-vaccinated chickens when challenged with either virulent subtype A or subtype B. Vaccination by aMPV subtype B in chickens is routinely carried out in many poultry producing countries to prevent respiratory infection and production losses arising from virulent aMPV infection (Marangon & Busani, Citation2007). To date, there has been limited published information on cross-protection between subtypes A and B in chickens (Cook et al., Citation1995), although previous reports have suggested the presence of such cross-protection in turkeys (Cook et al., Citation1995; Cook et al., Citation1999; Toquin et al., Citation1999). Based on clinical scoring, the current study has demonstrated cross-protection against a subtype A challenge in chickens that received a subtype B vaccination. Homologous protection was also demonstrated through the absence of clinical signs when vaccinated birds were challenged with a virulent subtype B virus. Since the discovery of aMPV infection (initially known as turkey rhinotracheitis) in 1978 (Buys, Citation1980), the degree of protection against aMPV has mainly been assessed by clinical scoring of birds (Naylor et al., Citation1992). Recently, aMPV protection studies have included the detection of viral genomes in swabs (OP and CL) and tissue samples collected following virulent aMPV challenge (Ganapathy & Jones, Citation2007; Ganapathy et al., Citation2010; Jardine et al., Citation2018). In this study, despite the absence of clinical signs, aMPV was detected in the turbinate (both subtypes) and trachea (subtype B only) of vaccinated chickens up to 9 dpc. Unfortunately, the viability of the detected virus is unknown as no isolation was attempted. Previous aMPV challenge studies have made little or no attempt to detect aMPV in the turbinate or lungs of challenged chickens (Hess et al., Citation2004; Ganapathy et al., Citation2010; Rautenschlein et al., Citation2011). In this study, aMPV RNA was detected in lung and turbinate tissues of subtype A- or subtype B-challenged groups. This indicates that alongside the trachea, both of these tissues should be included in vaccination/challenge studies.
In commercial broiler chickens, it was reported that the subtype B vaccine strain can be detected up to 21 dpv (Ganapathy et al., Citation2010). Results obtained from unvaccinated and vaccinated-challenged groups in this study suggest that the aMPV is cleared earlier from trachea and lungs, compared to the nasal turbinate. Liman & Rautenschlein (Citation2007) found turkey turbinate tissue to be virus-free by 21 days post-infection when challenged with either subtypes A or B (Liman & Rautenschlein, Citation2007). In this study, consistent prolonged detection of RNA of either subtype in turbinate tissue from vaccinated-challenged chickens, rather than from OP swabs, raises concerns regarding the current practice of mainly relying on OP swabs for diagnosis of aMPV. Our findings suggest that inclusion of the turbinate tissue alongside swab samples should be considered for improved aMPV detection in immunopathogenesis, diagnostic, epidemiological and vaccination-challenge activities.
Mucosal and innate immunity in the respiratory tract and associated tissues are crucial components of the host defence against chicken respiratory pathogens (Liew et al., Citation1984; Chhabra et al., Citation2015), but there have been limited reports investigating such aspects for aMPV infection in chickens. While chicken mucosal immunity is traditionally measured using monoclonal ELISAs to assay levels of virus-specific antibodies in lachrymal fluid, tracheal washes, or both (Hawkes et al., Citation1983; Ganapathy et al., Citation2005), this study attempted to use molecular methods to measure such parameters. Measurement of immune gene mRNA in different solid tissues has provided an opportunity to evaluate local immunity in the aMPV replication tissues (Chhabra et al., Citation2018). In the turbinate and lungs of unvaccinated-challenged chickens, IgA and IgY mRNA transcription was frequently significantly up-regulated after subtype B challenge compared to subtype A. However, the inverse was true for trachea samples at 6 dpc, with subtype A being significantly raised compared to subtype B. This possibly reflects preferential virus replication and host immune induction, which is inversely dependent on the infecting aMPV subtype – subtype B predominantly in the turbinate and lungs, compared to subtype A mostly in the trachea. For the vaccinated-challenged groups, findings were mixed with no clear pattern for either subtype or tissue type. Following subtype B challenge, significant IgA mRNA expression was found in the trachea and lungs at 3–9 dpc compared to IgY transcription. Meanwhile, following subtype A challenge, a significantly higher expression of IgY mRNA was found in all tissues when compared with IgA expression. With the importance of mucosal immunity (Ganapathy et al., Citation2005; Awad et al., Citation2015), and based on these mRNA transcription profiles, it appears that further studies are needed to ascertain the complex interactions found in this study. Conducting mRNA transcription assays in parallel to other bioassays, such as monoclonal IgA ELISAs of lachrymal fluid and tracheal washes and a detailed proteomic study, may provide further detail to better understand such relationships.
Comprehensive understanding of cellular immunity to aMPV, in addition to innate, mucosal and humoral antibody responses, can further improve understanding of immunopathogenesis and enhance vaccination strategies against this virus. In this study, following either subtype A or B challenge of unvaccinated (naïve) and vaccinated chickens, transcription of certain immune genes in turbinate, trachea and lung tissues was evaluated. An early (3 dpc) differential response was noted in the unvaccinated-challenged groups, where for both CD8-α and CD8-β, significantly higher up-regulation was found for subtype B-compared to subtype A-infected birds. At the later stage of infection (6 and 9 dpc), significantly higher expression was found in the trachea and lungs of birds with subtype A infection (particularly for CD8-β). This reflects an earlier host response to subtype B compared to subtype A, potentially associated with faster replication of subtype B. The importance of cellular immunity for aMPV clearance has been reported before (Liman & Rautenschlein, Citation2007), and it appears that this is likely dependent on the subtype of aMPV virus. In the unvaccinated-challenged groups, significant levels of both CD8 markers were found during early infection (3 dpc) after subtype B challenge; however, by 6 or 9 dpc, the response to subtype A challenge was significantly greater. This reflects the ability of subtype B to induce early cellular immune responses, and that it is possibly associated with higher tissue viral loads, also reflected by earlier and greater clinical signs. In comparing between the unvaccinated and vaccinated challenged groups, it is evident that the marked increase in transcription fold changes of CD8-α and CD8-β at 3 and 6 dpc in turbinate, trachea and lung tissues, could be used as potential quantitative protection markers in aMPV vaccination-challenge studies.
The magnitude and pattern of TLR3, TMPRSS12 and IFN-γ mRNA transcription was variable based on the subtype of the challenge virus, tissue type and days post-challenge. In the unvaccinated-challenged chicks, it appears that aMPV subtype B often had significantly higher levels of mRNA expression for these immune genes compared to subtype A. Subtype A induced significantly higher levels of TMPRRSS12 and IFN-γ at only two sampling points (3 dpc in lungs; 6 dpc in trachea). Overall, the magnitude of up-regulation induced by subtype B was significantly higher than subtype A, potentially reflecting that the subtype B challenge virus used in this study is more virulent that the subtype A strain. In the vaccinated-challenge groups, similar to the unvaccinated-challenge groups, mRNA expression of all genes in all tissues was dominated by subtype B, with the exception of three sampling points (3 dpc – TMPRSS12 in turbinate and trachea; 6 dpc – IFN-γ in turbinate). In comparing the magnitude of mRNA transcription levels between the unvaccinated and vaccinated groups, TMPRSS12 could be suggested as an indicator to distinguish vaccination status. The reasoning is that there was a 2–3 times increase in fold change for the vaccinated chickens compared to the unvaccinated group for either challenge subtype. For the interleukin mRNAs, the IL-1β, IL-6 and IL-18, significantly higher levels of mRNA were frequently detected in the subtype B-challenged groups, independent of vaccination status. This magnitude of expression was particularly higher in the trachea and lungs. In addition, the majority of differential gene transcription in the turbinate and trachea (e.g. TLR3 and IL-6) was noted at 3 and 6 dpc, which corresponded with the peak in clinical score. Overall, cross-comparisons between tissue types and sampling intervals for interleukin expression in the vaccinated-challenged and unvaccinated-challenged groups indicated negligible differences in fold change.
Results of this study suggested that the virulent aMPV subtype B strain appears to have been more virulent and immunogenic in commercial broiler chicks when compared to the subtype A strain. Despite a lower viral titre of virulent subtype B than subtype A, chicks challenged with subtype B produced significantly higher levels of aMPV-specific ELISA antibody titres at 10 dpc. In addition, the overall magnitude of assayed immune gene mRNA expressions was higher in the turbinate, trachea and lungs of chickens that received aMPV subtype B challenge compared to subtype A. Clinically, greater and longer persistence of clinical signs were found in the subtype B infection group. Furthermore, OP swabs demonstrated that the virulent subtype B virus was detected until 9 dpc, whereas subtype A was only detected up to 6 dpc. The increased antibody response and greater duration of clinical signs may be associated with the prolonged detection of the subtype B aMPV (Santos et al., Citation2012). Similar findings have been reported previously (Htut Aung et al., Citation2008; Paudel et al., Citation2016; Brown et al., Citation2019), which confirms that in chickens, subtype B is more virulent than subtype A.
In conclusion, clinical, serological and molecular findings from this study have demonstrated that vaccination of day-old broiler chickens with aMPV subtype B can induce protection against both homologous (subtype B) and heterologous (subtype A) challenge. The host immune response to challenge was dependent on the subtype of aMPV and vaccination status. Results of this study have shown that the turbinate and lungs, in addition to the trachea, should be examined in aMPV vaccination-challenge studies. Based on clinical signs, humoral antibody titres, and early immune responses as measured by mRNA gene transcription, it appears that the subtype B is either more virulent or immunogenic, compared to subtype A. Findings have shown that mRNA expression levels of CD8-α, CD8-β, TLR3 and IL-6, particularly in tissues of turbinate and trachea, could be further studied in aMPV vaccination-challenge studies.
Ethical approval
All experimental procedures were conducted according to UK legislation on the use of animals for experiments, as permitted under the project license PPL 112 40/3723. All procedures were approved by the University of Liverpool ethics committee prior to the study.
Disclosure statement
No potential conflict of interest was reported by the author(s).
Funding
The research was funded by Boehringer Ingelheim Animal Health, Lyon, France.
Additional information
Funding
References
- Abdel-Azeem, A.A., Franzo, G., Dalle Zotte, A., Drigo, M., Catelli, E., Lupini, C., Martini, M. & Cecchinato, M. (2014). First evidence of avian metapneumovirus subtype A infection in turkeys in Egypt. Tropical Animal Health and Production, 46, 1093–1097.
- Awad, F., Forrester, A., Baylis, M., Lemiere, S., Jones, R. & Ganapathy, K. (2015). Immune responses and interactions following simultaneous application of live Newcastle disease, infectious bronchitis and avian metapneumovirus vaccines in specific-pathogen-free chicks. Research in Veterinary Science, 98, 127–133.
- Ball, C., Forrester, A. & Ganapathy, K. (2018). Co-circulation of genetically diverse population of vaccine related and unrelated respiratory mycoplasmas and viruses in UK poultry flocks with health or production problems. Veterinary Microbiology, 225, 132–138.
- Bäyon-Auboyer, M.H., Arnauld, C., Toquin, D. & Eterradossi, N. (2000). Nucleotide sequences of the F, L and G protein genes of two non-A/non-B avian pneumoviruses (APV) reveal a novel APV subgroup. Journal of General Virology, 81, 2723–2733.
- Brown, P., Allée, C., Courtillon, C., Szerman, N., Lemaitre, E., Toquin, D., Mangart, J., Amelot, M. & Eterradossi, N. (2019). Host specificity of avian metapneumoviruses. Avian Pathology, 48, 311–318.
- Buys, S. (1980). A preliminary report on the isolation of a virus causing sinusitis in turkeys in South Africa and attempts to attenuate the virus. Turkeys, 28, 36.
- Canuti, M., Kroyer, A.N., Ojkic, D., Whitney, H.G., Robertson, G.J. & Lang, A.S. (2019). Discovery and characterization of novel RNA viruses in aquatic North American wild birds. Viruses, 11, 768.
- Cavanagh, D., Mawditt, K., Britton, P. & Naylor, C. (1999). Longitudinal field studies of infectious bronchitis virus and avian pneumovirus in broilers using type-specific polymerase chain reactions. Avian Pathology, 28, 593–605.
- Cha, R.M., Khatri, M., Mutnal, M. & Sharma, J.M. (2011). Pathogenic and immunogenic responses in turkeys following in ovo exposure to avian metapneumovirus subtype C. Veterinary Immunology and Immunopathology, 140, 30–36.
- Chacón, J.L., Brandão, P.E., Buim, M., Villarreal, L. & Ferreira, A.J. (2007). Detection by reverse transcriptase-polymerase chain reaction and molecular characterization of subtype B avian metapneumovirus isolated in Brazil. Avian Pathology, 36, 383–387.
- Chacón, J.L., Mizuma, M., Vejarano, M.P., Toquín, D., Eterradossi, N., Patnayak, D.P., Goyal, S.M. & Ferreira, A.J. (2011). Avian metapneumovirus subtypes circulating in Brazilian vaccinated and nonvaccinated chicken and turkey farms. Avian Diseases, 55, 82–89.
- Chhabra, R., Ball, C., Chantrey, J. & Ganapathy, K. (2018). Differential innate immune responses induced by classical and variant infectious bronchitis viruses in specific pathogen free chicks. Developmental & Comparitive Immunology, 87, 16–23.
- Chhabra, R., Forrester, A., Lemiere, S., Awad, F., Chantrey, J. & Ganapathy, K. (2015). Mucosal, cellular, and humoral immune responses induced by different live infectious bronchitis virus vaccination regimes and protection conferred against infectious bronchitis virus Q1 strain. Clinical and Vaccine Immunology, 22, 1050–1059.
- Chomczynski, P. & Sacchi, N. (2006). The single-step method of RNA isolation by acid guanidinium thiocyanate-phenol-chloroform extraction: twenty-something years on. Nature Protocols, 1, 581–585.
- Cook, J.K. (2000). Avian pneumovirus infections of turkeys and chickens. Veterinary Journal, 160, 118–125.
- Cook, J.K., Darbyshire, J. & Peters, R. (1976). The use of chicken tracheal organ cultures for the isolation and assay of avian infectious bronchitis virus. Archives of Virology, 50, 109–118.
- Cook, J.K., Huggins, M.B., Orbell, S.J. & Senne, D.A. (1999). Preliminary antigenic characterization of an avian pneumovirus isolated from commercial turkeys in Colorado, USA. Avian Pathology, 28, 607–617.
- Cook, J.K., Huggins, M.B., Woods, M.A., Orbell, S.J. & Mockett, A.P. (1995). Protection provided by a commercially available vaccine against different strains of turkey rhinotracheitis virus. Veterinary Record, 136, 392–393.
- Elhafi, G., Naylor, C., Savage, C. & Jones, R. (2004). Microwave or autoclave treatments destroy the infectivity of infectious bronchitis virus and avian pneumovirus but allow detection by reverse transcriptase-polymerase chain reaction. Avian Pathology, 33, 303–306.
- Ganapathy, K., Bufton, A., Pearson, A., Lemiere, S. & Jones, R.C. (2010). Vaccination of commercial broiler chicks against avian metapneumovirus infection: a comparison of drinking-water, spray and oculo-oral delivery methods. Vaccine, 28, 3944–3948.
- Ganapathy, K., Cargill, P.W. & Jones, R.C. (2005). A comparison of methods of inducing lachrymation and tear collection in chickens for detection of virus-specific immuoglobulins after infection with infectious bronchitis virus. Avian Pathology, 34, 248–251.
- Ganapathy, K. & Jones, R.C. (2007). Vaccination of chicks with live attenuated subtype B avian metapneumovirus vaccines: protection against challenge and immune responses can be unrelated to vaccine dose. Avian Diseases, 51, 733–737.
- Göbel, T.W., Schneider, K., Schaerer, B., Mejri, I., Puehler, F., Weigend, S., Staeheli, P. & Kaspers, B. (2003). IL-18 stimulates the proliferation and IFN-gamma release of CD4+ T cells in the chicken: conservation of a Th1-like system in a nonmammalian species. Journal of Immunology, 171, 1809–1815.
- Gough, R., Collins, M., Cox, W. & Chettle, N. (1988). Experimental infection of turkeys, chickens, ducks, geese, guinea fowl, pheasants and pigeons with turkey rhinotracheitis virus. Veterinary Record, 123, 58–59.
- Guionie, O., Toquin, D., Sellal, E., Bouley, S., Zwingelstein, F., Allée, C., Bougeard, S., Lemière, S. & Eterradossi, N. (2007). Laboratory evaluation of a quantitative real-time reverse transcription PCR assay for the detection and identification of the four subgroups of avian metapneumovirus. Journal of Virological Methods, 139, 150–158.
- Hartmann, S., Sid, H. & Rautenschlein, S. (2015). Avian metapneumovirus infection of chicken and turkey tracheal organ cultures: comparison of virus-host interactions. Avian Pathology, 44, 480–489.
- Hawkes, R.A., Darbyshire, J.H., Peters, R.W., Mockett, A.P.A. & Cavanagh, D. (1983). Presence of viral antigens and antibody in the trachea of chickens infected with avian infectious bronchitis virus. Avian Pathology, 12, 331–340.
- Hess, M., Huggins, M.B., Mudzamiri, R. & Heincz, U. (2004). Avian metapneumovirus excretion in vaccinated and non-vaccinated specified pathogen free laying chickens. Avian Pathology, 33, 35–40.
- Htut Aung, Y., Liman, M., Neumann, U. & Rautenschlein, S. (2008). Reproducibility of swollen sinuses in broilers by experimental infection with avian metapneumovirus subtypes A and B of turkey origin and their comparative pathogenesis. Avian Pathology, 37, 65–74.
- Jardine, C., Parmley, E., Buchanan, T., Nituch, L. & Ojkic, D. (2018). Avian metapneumovirus subtype C in wild waterfowl in Ontario, Canada. Transboundary and Emerging Diseases, 65, 1098–1102.
- Jones, R.C. (2010). Viral respiratory diseases (ILT, aMPV infections, IB): are they ever under control? British Poultry Science, 51, 1–11.
- Juhasz, K. & Easton, A.J. (1994). Extensive sequence variation in the attachment (G) protein gene of avian pneumovirus: evidence for two distinct subgroups. Journal of General Virology, 75, 2873–2880.
- Kameka, A.M., Haddadi, S., Kim, D.S., Cork, S.C. & Abdul-Careem, M.F. (2014). Induction of innate immune response following infectious bronchitis corona virus infection in the respiratory tract of chickens. Virology, 450, 114–121.
- Kuchipudi, S.V., Tellabati, M., Sebastian, S., Londt, B.Z., Jansen, C., Vervelde, L., Brookes, S.M., Brown, I.H., Dunham, S.P. & Chang, K.C. (2014). Highly pathogenic avian influenza virus infection in chickens but not ducks is associated with elevated host immune and pro-inflammatory responses. Veterinary Research, 45, 118.
- Liew, F.Y., Russell, S.M., Appleyard, G., Brand, C.M. & Beale, J. (1984). Cross-protection in mice infected with influenza A virus by the respiratory route is correlated with local IgA antibody rather than serum antibody or cytotoxic T cell reactivity. European Journal of Immunology, 14, 350–356.
- Liman, M. & Rautenschlein, S. (2007). Induction of local and systemic immune reactions following infection of turkeys with avian metapneumovirus (aMPV) subtypes A and B. Veterinary Immunology & Immunopathology, 115, 273–285.
- Livak, K.J. & Schmittgen, T.D. (2001). Analysis of relative gene expression data using real-time quantitative PCR and the 2-ΔΔCT method. Methods, 25, 402–408.
- Luo, L., Sabara, M.I. & Li, Y. (2009). Analysis of antigenic cross-reactivity between subgroup C avian pneumovirus and human metapneumovirus by using recombinant fusion proteins. Transboundary and Emerging Diseases, 56, 303–310.
- Marangon, S. & Busani, L. (2007). The use of vaccination in poultry production. Revue Scientifique et Technique-Office International des Epizooties, 26, 265–274.
- McDougall, J. & Cook, J.K.A. (1986). Turkey rhinotracheitis: preliminary investigations. Veterinary Record, 118, 206–207.
- Moscoso, H., Thayer, S.G., Hofacre, C.L. & Kleven, S.H. (2004). Inactivation, storage, and PCR detection of mycoplasma on FTA® filter paper. Avian Diseases, 48, 841–850.
- Naylor, C., Al-Ankari, A., Al-Afaleq, A., Bradbury, J. & Jones, R. (1992). Exacerbation of mycoplasma gallisepticum infection in turkeys by rhinotracheitis virus. Avian Pathology, 21, 295–305.
- Ndegwa, E.N., Joiner, K.S., Toro, H., van Ginkel, F.W. & van Santen, V.L. (2012). The proportion of specific viral subpopulations in attenuated Arkansas Delmarva poultry industry infectious bronchitis vaccines influences vaccination outcome. Avian Diseases, 56, 642–653.
- Okino, C.H., Mores, M.A., Trevisol, I.M., Coldebella, A., Montassier, H.J. & Brentano, L. (2017). Early immune responses and development of pathogenesis of avian infectious bronchitis viruses with different virulence profiles. PLoS One, 12, e0172275.
- Paudel, S., Easwaran, M., Jang, H., Jung, H.K., Kim, J.H. & Shin, H.J. (2016). Immunization with avian metapneumovirus harboring chicken Fc induces higher immune responses. Virus Research, 220, 129–135.
- Rautenschlein, S., Aung, Y.H. & Haase, C. (2011). Local and systemic immune responses following infection of broiler-type chickens with avian metapneumovirus subtypes A and B. Veterinary Immunology and Immunopathology, 140, 10–22.
- Santos, M.B.D., Martini, M.C., Ferreira, H.L., da Silva, L.H., Fellipe, P.A., Spilki, F.R. & Arns, C.W. (2012). Brazilian avian metapneumovirus subtypes A and B: experimental infection of broilers and evaluation of vaccine efficacy. Pesquisa Veterinária Brasileira, 32, 1257–1262.
- Schilling, M.A., Memari, S., Cattadori, I.M., Katani, R., Muhairwa, A.P., Buza, J.J. & Kapur, V. (2019). Innate immune genes associated with Newcastle disease virus load in chick embryos from inbred and outbred lines. Frontiers in Microbiology, 10, 1432.
- Śmiałek, M., Tykałowski, B., Pestka, D., Welenc, J., Stenzel, T. & Koncicki, A. (2016). Three-step anti-aMPV IgA expression profile evaluation in turkeys of different immunological status after TRT vaccination. Polish Journal of Veterinary Sciences, 19, 509–518.
- Sugiyama, M., Koimaru, H., Shiba, M., Ono, E., Nagata, T. & Ito, T. (2006). Drop of egg production in chickens by experimental infection with an avian metapneumovirus strain PLE8T1 derived from swollen head syndrome and the application to evaluate vaccine. The Journal of Veterinary Medical Science, 68, 783–787.
- Sun, S., Chen, F., Cao, S., Liu, J., Lei, W., Li, G., Song, Y., Lu, J., Liu, C., Qin, J. & Li, H. (2014). Isolation and characterization of a subtype C avian metapneumovirus circulating in Muscovy ducks in China. Veterinary Research, 45, 74–74.
- Toquin, D., Bäyon-Auboyer, M., Jestin, V. & Eterradossi, N. (1999, March 23–25). Résponse sérologique et protection croisée visa vis de l’infection par une souche non-A non-B du virus de la rhinotrachéite infectieuse de la dinde. Comptes rendus des 3emes Journées de la Recherche Avicole, St Malo, France, 223–224.
- Yun, B., Zhang, Y., Liu, Y., Guan, X., Wang, Y., Qi, X., Cui, H., Liu, C., Zhang, Y., Gao, H., Gao, L., Li, K., Gao, Y. & Wang, X. (2016). TMPRSS12 is an activating protease for subtype B avian metapneumovirus. Journal of Virology, 90, 11231–11246.
- Zegpi, R., Joiner, K., Van Santen, V. & Toro, H. (2020). Infectious bronchitis virus population structure defines immune response and protection. Avian Diseases, 64, 60–68.
- Zheng, W., Izaki, J., Furusawa, S. & Yoshimura, Y. (2001). A sensitive non-radioactive in situ hybridization method for the detection of chicken IgG γ-chain mRNA: a technique suitable for detecting of variety of mRNAs in tissue sections. Biological Procedures Online, 3, 1–7.