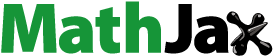
Abstract
Phthalates are a class of compounds that have been extensively used as plasticizers in different applications. Several phthalates have been recognized as substances of very high concern (SVHCs) in the EU, because of their toxicity for reproduction. However, high amounts of other phthalates are still produced and imported in the European Economic Area. In China and the US, recent studies show increasing concentrations of several phthalates in the air and in human urine, respectively. The understanding of phthalate absorption, distribution, metabolism, and elimination (‘pharmacokinetics’) in the organism is still limited. Specifically, phthalate partitioning among tissues is insufficiently understood. Here, we estimate partition coefficient (PC) values for different phthalates by using five algorithms and compare them to experimental (in-vivo and in-vitro) PC values. In addition, we review all pharmacokinetic steps for phthalates in human and rat, based on data from 133 peer-reviewed publications. We analyze the factors that determine phthalate partitioning and pharmacokinetics. Four processes are particularly relevant to phthalate distribution: protein binding, ionization, passive partitioning, and metabolism in different tissues. The interplay of these processes needs to be better represented in methods for determining the PC values of phthalates. The hydrophobicity of phthalates affects all pharmacokinetic steps. The exposure route has an influence on specific steps of phthalate pharmacokinetics but generally does not affect the pattern of metabolites in urine. The age of the organism has an influence on phthalate metabolism. More studies on the protein-bound fraction of phthalates in plasma and pharmacokinetic studies following inhalation and dermal exposure are desirable.
Introduction
Phthalates are esters of phthalic acid that have been widely used as plasticizers. The ingestion of contaminated foodstuffs, dermal application of personal care products, air inhalation, or iatrogenic contact with phthalate-containing materials have been reported as important routes of exposure to these compounds (Buchta et al. Citation2003; Wormuth et al. Citation2006). Due to toxic effects on reproduction, di(2-ethylhexyl) phthalate (DEHP), butylbenzyl phthalate (BBP), dibutyl phthalate (DBP), and diisobutyl phthalate (DIBP) have been included in the Regulation (EC) 1907/2006 on the Registration, Evaluation, Authorisation and Restriction of Chemicals (REACH, annex XIV) as substances of very high concern (SVHCs), and their production and import was forbidden in February 2015 (‘sunset date’), with exemptions for packaging of medicinal products. Certain uses of di-isononyl phthalate (DINP), di-isodecyl phthalate (DIDP), and di-n-octyl phthalate (DnOP) are also restricted under REACH (annex XVII). Conversely, diethyl phthalate (DEP), dimethyl phthalate (DMP), di(2-propylheptyl) phthalate (DPHP), and related plasticizers like di(2-ethylhexyl) terephthalate (DEHT) are not regulated under REACH and high amounts of these compounds are produced. Specifically, more than 103 tonnes/year of DEP and DMP and more than 105 tonnes/year of DPHP and DEHT are manufactured and/or imported in the European Economic Area (ECHA Citation2018). In China, from 2000 to 2010, a strong increase in the concentrations of DEHP and total phthalates in air has been reported (Chen et al. Citation2012). For the same period, in the US, the urinary concentrations of metabolites derived from DEHP, DEP, and BBP decreased by 30–40% while the concentrations of metabolites derived from DIBP and DINP increased by 200 and 150%, respectively (Zota et al. Citation2014). Human biomonitoring (HBM) programs and studies on phthalates conducted in Europe, North America (US and Canada), and Australia have been reviewed by Choi et al. (Citation2017). Across continents, the urinary concentrations (geometric mean, µg/L) of metabolites derived from DEHP, BBP, DBP, and DEP in children and adults were generally lower than the biomonitoring equivalent values (BE, µg/L, or µg/g creatinine) of these compounds, estimated from exposure guidance values by Aylward et al. (Citation2009a, Citation2009b). However, the 95th percentiles of urinary concentrations of monobutyl phthalate (MBP) and mono(2-ethylhexyl) phthalate (MEHP) in children exceeded in some cases the corresponding BE values (Choi et al. Citation2017). In Asia, the median concentrations of metabolites derived from DEHP, BBP, DBP, and DEP in urine from adults by Guo et al. (Citation2011) were on the same order of magnitude as the values reported in other continents by Choi et al. (Citation2017). Importantly, specific population groups are exposed to higher levels of phthalates than the general population. First, in occupationally exposed workers, the median urinary concentrations (µg/g creatinine) of MBP- and MEHP-derived compounds reported by Hines et al. (Citation2009) were in several cases similar or higher than the BE values of these compounds. Second, in general intensive-care-unit patients, all the maximum concentrations (µg/L) of MEHP-derived compounds in urine by Huygh et al. (Citation2015) were higher than the maximum BE values of these compounds.
Concerning phthalate pharmacokinetics, an important gap exists in the literature. The steady-state partitioning of these compounds in the organism is still unclear and major differences in partition coefficient (PC) values are observed between different estimation methods. A starting point for the analysis of phthalate PC values is provided by the physiologically-based pharmacokinetic (PBPK) models for di-n-butyl phthalate (DnBP) and DEHP developed by Keys et al. (Citation1999, Citation2000). These authors estimated the PC values between tissues and blood for phthalates either by means of the algorithm of Poulin and Krishnan (Citation1995b) or by in-vitro measurements. In fat, the PC values estimated algorithmically for the monoester metabolites were between 2 and 3 orders of magnitude higher than the in-vitro measurements for these compounds. No further information concerning phthalate partitioning has been found in relatively recent PBPK models for phthalates (Clewell et al. Citation2008; Gentry et al. Citation2011; Sharma et al. Citation2018). Moreover, in the complex PBPK models for phthalates by Clewell et al. (Citation2008) and Gentry et al. (Citation2011), some PC values were used as fitting parameters in a fit of the model to measured concentrations, together with a high number of additional fitting parameters (>30). There is substantial uncertainty associated with this approach, since the fitted values are influenced by the model structure and the choice of experimental studies used for fitting. Moreover, the PBPK models from Clewell et al. (Citation2008) and Gentry et al. (Citation2011), similarly to those from Keys et al. (Citation1999, Citation2000), did not explicitly represent the binding of each parent phthalate and its metabolites to plasma proteins (this process was included for MEHP in the recent PBPK model from Sharma et al. (Citation2018)). Protein binding may have an impact on the fitted PC values. In addition to fitting, there are other methods for estimating PC values: (1) estimation algorithms, (2) in-vitro, or (3) in-vivo measurements, but there is no consensus about the best method for estimating phthalate PC values.
Several reviews of experimental studies on phthalate pharmacokinetics have been published (Albro, Corbett, et al. Citation1982; Kluwe Citation1982; Albro and Lavenhar Citation1989; Fay et al. Citation1999; Kavlock Citation2002a–2002g; Frederiksen et al. Citation2007). In short, these reviews agreed on several conclusions: high metabolism of phthalate diesters in the gastrointestinal tract and absorption principally as monoesters, high binding of DEHP and MEHP to plasma proteins, generally slight qualitative differences in the metabolic pathways among species, existence of biliary excretion and enterohepatic recycling of some phthalates, and high elimination in urine. We have not found any review which analyzed the steady-state partitioning of phthalates in the organism. Additional gaps have been indicated, including the possible influence of the exposure route, the age, and the physiological stage (weeks of pregnancy) on phthalate pharmacokinetics (Frederiksen et al. Citation2007).
Here, we analyze the steady-state partitioning of phthalates and compare different methods of PC estimation. First, we estimate PC values for phthalates by using five different algorithms, including that from Poulin and Krishnan (Citation1995b), used in PBPK models for these compounds. Second, by applying strict selection criteria, we compile available experimental (in-vivo and in-vitro) data on steady-state PC values for phthalates. Third, we compare the results from both methods. To summarize, we show that four main processes, binding, ionization, passive partitioning, and metabolism of phthalates in different tissues, determine the distribution of these compounds and explain the variability in PC values between estimation methods. Based on the lack of information available in the literature regarding the relative importance of these processes for different phthalates, we recommend the use of in-vitro measurements for PC estimation. This analysis of phthalate partitioning is conducted as the main objective of this work and is part of a broader overview of all pharmacokinetic steps for these compounds in human and rat. To this end, we review available data on absorption, distribution, metabolism, and elimination of different phthalates. Pharmacokinetic studies for related plasticizers, including DEHT, bis(2-ethylhexyl) tetrabromophthalate (BEH-TEBP), and diisononyl cyclohexane-1,2-dicarboxylate (DINCH) are also reviewed.
Material and methods
Literature search and data compilation
The literature search was based on the Web of Science. Several combinations of keywords were used (Supplemental material, Table S1). The flow diagram of literature research and data compilation, based on PRISMA (Liberati et al. Citation2009), is shown in Figure S1. The compiled data for human and rat analyzed in this publication are shown in the Supplementary material (Tables S2–S51).
We focused on experimental pharmacokinetic (in-vivo dosing, in-vitro) and PBPK modeling studies in adult humans and rats that neither provoked nor focused on toxicity. Additional studies on algorithms for estimation of the partition coefficients, on physico-chemical properties of phthalates, and on HBM for more than one population group (notably, different age groups) were selected. The criteria for selection and exclusion of papers during the title and abstract screening and during assessment for eligibility of full articles are detailed in the Supplementary material (literature search and data compilation, Table S1). Data from 133 publications were compiled and analyzed, including (1) experimental studies in rat and human (N = 85), (2) PBPK models in rat and human (N = 4), and (3) development of algorithms for PC estimation (N = 5). Additional criteria for data compilation included:
To estimate experimental PC of phthalates, we selected in-vitro data at steady-state or pharmacokinetic in-vivo data reported after extended exposure (for example, intravenous infusion maintained for several hours, oral dosing for several days), followed by sampling within a few hours (2 h) after the last dosing, with tissue distribution results expressed in units equivalent to mg compound/g tissue.
The experimental t1/2 values were calculated in the reviewed publications by considering either one-phase depuration kinetics or just the terminal phase in the case of two-phase depuration kinetics.
In the case of exposure to a radiolabelled phthalate and report of results expressed in % of dose, which was generally the case for data of elimination in feces and urine and biliary excretion, we considered that the results included the parent compound and its metabolites. The data from a diester phthalate and its metabolites (i.e. DEHP + MEHP) were in this case pooled before analysis.
The number of experimental studies on phthalate pharmacokinetics published per decade was relatively stable in the 1970s and the 1980s, decreased in the 1990s, and has increased since the 2000s. These experiments consisted generally of in-vivo studies of the pharmacokinetics of DEHP and DBP in rat. In humans, in-vivo studies consisted generally of the administration of a parent phthalate and analysis of the concentration of its metabolites in urine and blood. In our database, each value was listed together with the following information: reference, type of study (in-vivo or in-vitro experiments, PBPK models, development of algorithms for estimation of PC values), species, administered and/or measured compound, route of exposure, duration of exposure, dose, matrix (tissue), units, and results.
Selection of algorithms for PC estimation
To estimate algorithmic PC values we selected five algorithms considered suitable for organic substances (Table S2). To our knowledge, no previous study on phthalate distribution compared several algorithms to estimate PC values for these compounds. Specifically, in agreement with the classification of Peyret et al. (Citation2010), we selected the mechanistic algorithms of Poulin and Krishnan (Citation1995a, Citation1995b). Based on the results of the comparison of algorithms performed by Payne and Kenny (Citation2002), we also selected the algorithms from Fiserova-Bergerova and Diaz (Citation1986) and Balaz and Lukacova (Citation1999). These two last algorithms as well as that from DeJongh et al. (Citation1997) can be considered as semi-empirical, in which some parameters were mechanistic while other ones were fitted to experimental data (Payne and Kenny Citation2002). The algorithms were developed by their authors for compounds with log octanol/water partition coefficient (log Kow) values from −0.8 to 4.8 (applicability domain of hydrophobicity). These algorithms were in general developed for volatile and in some cases non-volatile, non-amphiphilic, and non-ionizable organic compounds (Balaz and Lukacova Citation1999) that neither presented a significant binding nor were highly metabolized in the studied tissues. Finally, we compiled also several algorithmic PC values used in published PBPK models for phthalates.
Classification in ‘phthalate groups’
Following our study of metabolic pathways for phthalates and considering the physico-chemical properties (log Kow) of the parent compounds, we were able to assign most of the studied phthalates to four ‘phthalate groups:’ ‘group A’, lead compound DEHP; ‘B’, lead compounds BBP and DBP; ‘C’, lead compounds DEP and DMP; ‘D’, lead compounds DIDP, DINP, DnOP, and DIOP (Table S3). Each ‘phthalate group’ includes (1) either one or several parent phthalates that follow similar metabolic pathways (see Supplementary material Section 10.1) and present similar hydrophobicity (log Kow) and (2) the corresponding metabolites.
Calculations
Algorithmic and experimental PC values
The algorithmic estimation of PC values required the specification of physico-chemical information about the compound as well as physiological data about the species studied (Table S2). The physico-chemical properties of phthalates used in these estimations are shown in . Each algorithm was applied only to compounds with log Kow values within the applicability domain of hydrophobicity of the algorithm. The physiological information for the estimation of PC values was taken from the same publication in which the algorithm was developed.
Table 1. Physico-chemical properties of phthalates considered for algorithmic estimation of partition coefficient (PC) values.
The estimation of experimental in-vivo PC values was made by dividing, at steady state, the concentration of the compound in a tissue by its concentration in plasma (Kim et al. Citation2015). The experimental in-vitro PC values were measured by Keys et al. (Citation1999, Citation2000) by applying the vial-equilibration method developed by Murphy et al. (Citation1995) (see Supplementary material Section 5.1).
Other calculations
In order to analyze the influence of physiology (children vs. adults; weeks of pregnancy) on metabolism, we compiled data on the median concentrations of parent phthalates and their metabolites in human urine from biomonitoring studies in which the data for different population groups were presented. We calculated the ratios of (1) the concentration of each metabolite to the concentration of parent compound and (2) the concentration of each oxidized metabolite to the concentration of the simple monoester. Within each study, we compared these ratios between population groups (see Supplementary material section 10.4).
Results
Distribution
Overview
The ‘early distribution’ of phthalates in the organism has been reported by several studies. We use the term ‘early distribution’ to differentiate it from ‘steady-state partitioning’. These studies conducted either a single or repeated exposure to a radiolabelled parent phthalate in rat and reported the levels of total radioactivity (% of dose) in the tissues at different sampling times.
The data show a strong influence of the exposure route on the early distribution of phthalates in the body. Specifically, after oral exposure to radiolabelled butylglycolylbutyl phthalate (BGBP), DBP, DEHP, MEHP, or DIOP the radioactivity recovery (% of dose) in the tissues was low at all sampling times. For example, the sum of radioactivity in liver, blood, and fat remained in general below 5% between 2 and 4 h and 24 h after exposure (Tanaka et al. Citation1975; Williams and Blanchfield Citation1975; Chu et al. Citation1978; Ikeda et al. Citation1978; Citation1980). In contrast, after intravenous exposure to radiolabelled-DEHP or MEHP, a high radioactivity recovery was reported in some tissues. For example, in the liver the radioactivity reached a peak (40–60%) within 2 h, in liver and lungs the radioactivity remained generally high within 24 h (14–30% in each tissue (Jaeger and Rubin Citation1973; Daniel and Bratt Citation1974; Tanaka et al. Citation1975; Chu et al. Citation1978)).
Partition coefficients
The number of compiled PC values (all phthalates) is 52 in fat (), 50 in liver, and between 10 and 42 in other tissues (Figures S2–S6 and Tables S4–S11). A majority of these values were algorithmically estimated in this review. For instance, in fat, the compiled values consisted of values we estimated algorithmically (N = 41), values estimated algorithmically in published PBPK models for phthalates (N = 6), and experimental values (in-vivo or in-vitro, N = 5). The irrigation matrix was in general the blood.
Figure 1. Partition coefficients (PC) of phthalates between fat and blood/plasma, in rat and human, estimated by different methods. The compounds are organized by increasing log Kow values of the nonionized compounds. Full triangles, raw data; empty triangles, median value. Number of values (N) for human (H) and rat (R) per compound: MMP to MEP (H, 3; R, 2); DEP (H, 3; R, 1); MBP (H, 3; R, 2); MnBP (R, 3); MBzP (R, 1); DIBP and BGBP (H, 2; R, 1); BBP (H, 2; R, 2); DnBP and DBP (H, 2; R, 1); MEHP (H, 2; R, 4); DEHP (H, 1; R, 1). All abbreviations are defined in Supplementary material.
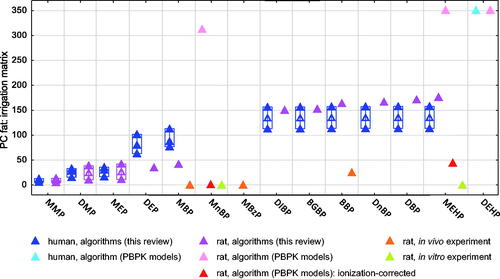
The experimental PC values of all phthalates in all tissues (N = 22) were lower than 5, with the exception of the in-vivo PC value of BBP in fat (25.0, Kim et al. Citation2015). For MBP and MnBP, the PC values measured in-vivo using plasma as irrigation matrix (Kim et al. Citation2015) compared to those measured in-vitro on the basis of blood (Keys et al. Citation2000) were very close in fat and liver (within a factor of 2) and close in testis (within a factor of 8). Thus, PC values measured by different experimental approaches (in-vivo and in-vitro) using either blood or plasma as irrigation matrices were consistent.
Measured (experimental) and algorithmically estimated PC values in rat show strong and systematic differences (, S2, and S3). Notably, in fat the algorithmic PC values for MEHP, MnBP, and MBP are between 2 and 3 orders of magnitude higher than the experimental PC values, see . In liver and muscle, the differences were around 1 order of magnitude. Our algorithmically estimated PC values were closer to the experimental values than algorithmic PC values used in PBPK models in fat. This is explained by the fact that, in our review, each algorithm was used only for compounds comprised within its applicability domain of hydrophobicity. Importantly, the differences between algorithmic and experimental values reported by Keys et al. (Citation1999, Citation2000) were drastically reduced when these authors corrected the algorithmic PC values by considering the ionization of MnBP and MEHP at physiological pH (, S2, and S3).
An important factor influencing many of the algorithmic PC values is hydrophobicity (higher PC values for more hydrophobic phthalates). Similarly, the experimental in-vivo PC values for compounds within the ‘phthalate group B’ (i.e. from MBP or MBzP to BBP in and S5) increase with increasing hydrophobicity of these compounds in fat and brain. Conversely, in liver and kidney the opposite trend was observed (Figures S2 and S4), which may be related to the capacity of liver and kidney to metabolize phthalate diesters to their monoesters (Tables S47 and S49).
Binding to plasma proteins
The binding of phthalates to proteins in blood/plasma and in tissues determines the fraction of these compounds that remains free (unbound) in these matrices and, by consequence, available for passive transfer between each tissue and the blood/plasma (partitioning). In human blood, DEHP accumulates mainly in plasma (approx. 85% of total recovery; Jaeger and Rubin Citation1973), where almost all DEHP (100%) and MEHP (97%) are bound to plasma proteins (Shintani Citation2000) (see Supplementary material section 6.1). The mechanisms of phthalate binding in serum have been studied recently (Xie et al. Citation2011; Yuan et al. Citation2014; Yue et al. Citation2014; Wang and Zhang Citation2015) (see Supplementary material section 6.1). The binding of phthalates in other tissues has also been confirmed (see Supplementary material section 6.2). However, data on the total bound/unbound fractions of different phthalates in plasma/blood and in other tissues are scarce.
Half-life
A total of 131 half-life values (t1/2, h) of phthalates, their metabolites, and DINCH metabolites were compiled. Most of these values were measured in blood, plasma, or serum (N = 50 in rat, N = 7 in human) and urine (N = 40 in human, N = 13 in rat) (). The t1/2 values measured in other tissues in rat (N = 21) are shown in Figure S8.
Figure 2. Half-life values of phthalates and DINCH derivatives in blood/plasma and urine in human and rat. The compounds are presented per ‘phthalate groups’ (cf. Table S3). Full triangles, raw data; empty triangles, median value. Number of values (N) in human blood/plasma (HB), human urine (HU), rat blood/plasma (RB) and rat urine (RU) per compound: 2-cx-MMHP and 5-cx-MEPP (HB, 1; HU, 2); 5-OH-MEHP and 5-oxo-MEHP (HB, 1; HU, 4); DEHP (HB, 1; RB, 13; RU, 2); MEHP (HB, 2; HU, 4; RB, 10); 2-OH-MiBP to 3-OH-MiBP (HU, 1); 3-OH-MnBP and 4-OH-MnBP (HU, 2); BBP (RB, 2); DBP (RB, 1); DnBP (RB, 2); MBP (RB, 12); MBP-G (RB, 7); MBzP (RB, 1); MiBP (HU, 1); MnBP (HU, 2); BBP monoesters (RB, 1); MCPP (HU, 1; RU, 1); DINP (RB, 1); MCHpP to MOOP (RU, 1); cx-MPHxP to oxo-MPHP (HU, 1); cx-MINCH to CHDA (HU, 2). All abbreviations are defined in Supplementary material.
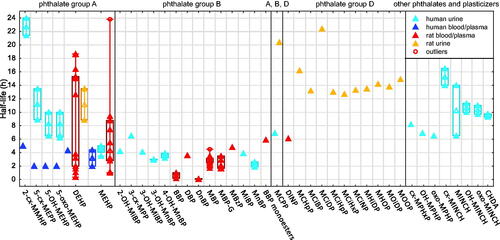
As shown in , all t1/2 values in human and rat blood/plasma and urine are lower than 24 h.
The t1/2 values vary between the ‘phthalate groups’. Specifically, for compounds within the ‘group B’, the t1/2 values measured in human urine (N = 10) and rat blood/plasma (N = 26), were slightly lower (< 8 h) than in other groups. The data also suggest an influence of other factors on the t1/2 values of phthalates, including the matrices for sampling (urine > blood/plasma) and possibly the species (rat > human) ( and Supplementary material section 7.1). More data would be needed to confirm the influence of these factors. Neither the factors mentioned (i.e. ‘phthalate groups’, matrix, and species) nor additional factors including the route of exposure and the dose explained the high variability of the t1/2 values of DEHP and MEHP in rat blood/plasma, with values that ranged from 1 h to more than 18 h for both compounds ( and S7).
Similarly to the differences in t1/2 values in blood/plasma and urine between ‘phthalate groups’, the t1/2 values in fat and other matrices in rat for compounds within the ‘group B’ were generally low (<12 h, N = 6), while the t1/2 values in fat of DEHP and MEHP were 126 ± 42 h (N = 2) and 68 h (N = 1), respectively (Figure S8).
Elimination
In general, the compiled data on phthalate elimination came from experiments in which humans or rats were exposed to radiolabelled phthalates by oral, intravenous, or dermal routes, the urine and feces were collected for a period (cumulative elimination) of 24–72 h (oral, intravenous exposure) or 5–7 d (dermal exposure), and the radioactivity (% of dose) was measured.
We compiled and analyzed 82 values of elimination of phthalates, DEHT, and BEH-TEBP in rat urine and feces and in human urine. Since we observed an influence of the dose on the elimination of phthalates in urine and feces, we present here the results at doses below 1000 mg/kg (number of values, N in rat: N = 33 in urine, N = 29 in feces, N = 7 in urine + feces; human: N = 5 in urine). The results at high doses in rat (N = 8) are shown in the Supplementary material (see Section 8.2).
The data in rat show an influence of the exposure route and the physico-chemical properties of parent phthalates (hydrophobicity) on the elimination of these compounds in urine and feces ().
Figure 3. Cumulative elimination of phthalates in feces and urine (% of dose) in rat, at doses < 1000 mg/kg, per exposure route. The compounds are organized from lower to higher log Kow of the parent compounds. Full triangles, raw data; empty triangles, median value. Number of values in rat urine (U), feces (F), and urine + feces (T) after oral (po), intravenous (IV), or dermal (D) exposure per compound: DMP to DiBP (T-D, 1); BGBP (F-po, 1; U-po, 1); DnBP (F-IV, 2; U-IV, 2); DBP (F-po, 2; U-po, 2; T-D, 1); BBP (F-po, 3; F-IV, 1; U-po, 3; U-IV, 1; T-D, 1); nBBP (U-po, 3); DEHP + MEHP (F-po, 8; F-IV, 2; U-po, 9; U-IV, 2; T-D, 1); DIOP (F-po, 1; U-po, 1); DINP (F-po, 5; U-po, 5); DIDP (T-D, 1); DEHT (F-po, 1; U-po, 1) and BEH-TEBP (F-po, 2; F-IV, 1; U-po, 2; U-IV, 1). All abbreviations are defined in Supplementary material.
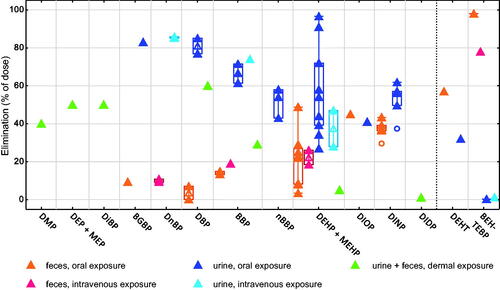
Regarding the exposure route, the total elimination of different phthalates in urine plus feces after dermal exposure was low (60% or less of the dose applied), compared to the total elimination (sum of the median eliminations in urine and feces) of these compounds after oral or intravenous exposure (generally higher than 80%).
Regarding the physico-chemical properties (hydrophobicity) of the parent phthalates, the findings depend on the exposure route. First, after dermal exposure, the total elimination of phthalates in urine plus feces diminished with increasing hydrophobicity of the compounds (30–60% of the dose applied for less hydrophobic phthalates, 1–5% for the more hydrophobic DEHP and DIDP (Elsisi et al. Citation1989)). The relative elimination in urine vs. feces after dermal exposure was not reported. Second, after intravenous and oral exposure, the hydrophobicity did not have an influence on the total elimination (urine plus feces) of phthalates, but it had an influence on the relative elimination of these compounds in urine vs. feces. Specifically, after oral and dermal exposure, phthalates with relatively low hydrophobicity (in , from DMP to nBBP, log Kow of the parent compounds below 5) were eliminated mainly in urine (median elimination in urine between 54 and 86% of the dose), with a minor elimination in feces (<20%). For more hydrophobic phthalates and related plasticizers (in , from DEHP + MEHP to DEHT, log Kow of the parent compounds between 7 and 10, approximately), the median elimination in feces was higher, compared to less hydrophobic compounds. The highly hydrophobic brominated substance BEH-TEBP (log Kow around 12.0), was eliminated mostly in feces (78–98% of the dose).
The data in humans after oral exposure (Figure S10, molar % of the dose) were generally coherent with the results reported in rats after oral and intravenous exposure and indicate a higher elimination in urine of less hydrophobic phthalates, compared to more hydrophobic ones. Specifically, the elimination in urine was high (approximately 90% of the molar dose) for phthalates like DIBP and DnBP (log Kow < 5), and it was slightly lower (68%) for the more hydrophobic DEHP. The relatively low elimination in human urine of DINCH derivatives (36%) was similar to the results for the highly hydrophobic DEHT in rat.
Bioavailability, absorption, bioaccessibility, and first-pass metabolism
‘Bioavailability’ refers to the amount of dose that is absorbed, by-passes first-pass metabolism, and reaches the systemic circulation (Buxton and Benet Citation2011). The ‘inhalation bioaccessibility’ in artificial lung fluids refers to the fraction of compound dissolved in these fluids and potentially bioavailable in the respiratory system (Kastury et al. Citation2017).
Phthalate bioavailability, similarly to phthalate elimination, is affected by the route of exposure and the hydrophobicity of these compounds.
For instance, after dermal exposure, the total elimination of phthalates in urine plus feces within 5–7 d reported by Elsisi et al. (Citation1989) directly reflects a dermal bioavailability of at least 30–60% for less hydrophobic phthalates vs. 1–5% for more hydrophobic ones. Similarly, McKee et al. (Citation2002) reported that 2–4% of a dose of the highly hydrophobic DINP was dermally absorbed in rat within 7 d. These results are in agreement with a decrease of in-vitro dermal absorption of phthalates in human and rat skin with increasing hydrophobicity of these compounds (Scott et al. Citation1987). These results may be explained by a low permeation of more hydrophobic diester phthalates across the skin (Hopf et al. Citation2014; Sugino et al. Citation2017).
After oral exposure, White et al. (Citation1980) reported a higher absorption rate (µmol/h) for less hydrophobic phthalate monoesters than for their corresponding diesters (MMP > DMP, MnBP > DnBP, and MEHP > DEHP) in small intestine preparations from rat. Within each class of compounds (monoesters and diesters), a trend for lower absorption rates with increasing hydrophobicity was shown. Accordingly, the absorption rate is influenced by the type of compound (monoester metabolite > parent diester) and by the hydrophobicity (in general, lower absorption rate for more hydrophobic compounds).
After inhalation, a lower bioaccessibility and bioavailability of more hydrophobic compounds is also observed. For instance, the inhalation bioaccessibility was higher for DMP and DEP (>75%) > DIBP and DnBP (10–15%) > DEHP, DINP, DEHT, and DINCH (<5%) (Kademoglou et al. Citation2018). In addition, in-vivo estimations considered a higher fraction of inhaled particles which deposited and were available for absorption in the respiratory tract for DEP than for DEHP (Andersen et al. Citation2018; Krais et al. Citation2018).
Concerning first-pass metabolism of diester phthalates, the data suggest that this process is important after oral and dermal exposure. As a result, after exposure to phthalate diesters by these routes, these compounds are mainly bioavailable as monoesters. Specifically, after oral exposure, DMP, DnBP, and DEHP were highly metabolized to monoester derivatives in the intestinal wall and absorbed principally (>80%) as monoesters (White et al. Citation1980). After dermal exposure, a high first-pass metabolism of DBP and BBP in human and rat skin (Sugino et al. Citation2017) and of absorbed DEHP in human skin (Hopf et al. Citation2014) were reported.
Biliary excretion and enterohepatic recycling
Data on cumulative excretion of phthalates in bile (% of dose) in rat were scarce, but showed consistent differences between exposure routes (Figure S12). Specifically, a higher biliary excretion was reported for DEHP + MEHP after intravenous (39 ± 14%, N = 3) than after oral (7 ± 5%, N = 6) exposure. Similarly, the biliary excretion of DnBP (Payan et al. Citation2001) and BEH-TEBP (Knudsen et al. Citation2017) was higher after intravenous than after oral or dermal exposure (N = 1 per compound and exposure route).
Following biliary excretion, an enterohepatic recycling of some phthalates was reported. The data suggest a higher enterohepatic recycling for less hydrophobic phthalates than for more hydrophobic ones. Specifically, after intravenous exposure to DnBP in rats, around 75–80% of DnBP-derived compounds excreted in bile were reabsorbed in the gut and later eliminated in urine (Payan et al. Citation2001), with similar results reported after intravenous exposure to BBP (Eigenberg et al. Citation1986). Conversely, a low enterohepatic recycling of the highly hydrophobic BEH-TEBP is suggested, since this compound was almost exclusively eliminated in feces both after oral or intravenous exposure (Knudsen et al. Citation2017). The observed influence of hydrophobicity on enterohepatic recycling is coherent with the influence of hydrophobicity on gastrointestinal absorption and bioavailability of these compounds.
Metabolism
Overview
Phthalates are metabolized in different tissues in human and rat, including intestine, liver, and plasma (see Supplementary material section 10.2) (Shintani Citation2000). The pathways of metabolism of phthalates in human and rat are explained in the Supplementary material (see section 10.1, Tables S37–S46). These pathways were previously reviewed by Frederiksen et al. (Citation2007). Briefly, the diester phthalates are firstly hydrolyzed to monoesters, which are generally further glucuronidated and/or oxidated. The metabolic pathways depend on the physico-chemical properties of phthalates and are simpler for less hydrophobic phthalates with lower molecular weight (i.e. DEP) than for more hydrophobic ones. Between human and rat, the differences in metabolic pathways are quantitative, rather than qualitative.
Influence of the exposure route and the dose
Concerning the exposure route, the data compiled show that this factor generally does not have an important influence on the pattern of phthalate metabolites in urine. Specifically, no important differences in the pattern of metabolites (% of measured metabolites) and even in total elimination (% of dose) in urine were observed after intravenous and oral exposure to DEHP in human and rat (Schulz and Rubin Citation1973; Koch, Bolt, Preuss, Angerer Citation2005; Koch, Bolt, Preuss, Eckstein, et al. Citation2005, Tables S37 and S38). Similarly, the pattern of metabolites in urine (% of measured compounds) was relatively close between intravenous and dermal exposure to DnBP (Payan et al. Citation2001, Table S44) and between oral and subcutaneous exposure to DINCH (Silva et al. Citation2012) in rat.
Conversely, the dose has an influence on the pattern of phthalate metabolites in urine (see Supplementary material section 10.3). Briefly, a saturation of the metabolism of monoester phthalates at high doses (above 200 mg/kg) is suggested (Eigenberg et al. Citation1986; Kessler et al. Citation2004). Accordingly, we suggest that the differences in the pattern of metabolites in urine after oral (162 mg/kg) and intravenous (0.07 mg/kg) exposure to DEHP in rats reported by Albro, Hass, et al. (Citation1982) may have been related to the dose, rather than the exposure route.
Influence of physiology
The data compiled show a higher oxidative metabolism of phthalates in children than in adults, more prominent in younger children. Specifically, for DEHP, the ratio of the urinary molar concentration of each oxidized metabolite (5-OH-MEHP, 5-oxo-MEHP, and 5-cx-MEPP) to the molar concentration of MEHP is approximately twice in children compared to adults, while the opposite is observed for the ratio of MEHP molar concentration to DEHP molar concentration (Koch et al. Citation2004; Kasper-Sonnenberg et al. Citation2012; Song et al. Citation2013). Similar differences between age groups are observed when younger children (3–5 year-old) are compared to teenagers (12–14 year-old, Becker et al. Citation2009) or for babies and toddlers (1 month to 2 year-old) compared to children from 2 to 6 year-old (Song et al. Citation2013). Similar age differences are observed for DnBP and DIDP and, to a lower degree, for DINP (Becker et al. Citation2009; Kasper-Sonnenberg et al. Citation2012). The reported age differences are highly coherent between studies.
Other physiological factors have been studied (see Supplementary material section 10.4) and suggest a slight increase in oxidative metabolism of DEHP at later weeks of pregnancy (Johns et al. Citation2016; Zhao et al. Citation2018). More data would be needed to determine the possible influence of these additional factors.
Discussion
Partition coefficients
The PC values for phthalates are highly influenced by the method of estimation (algorithmic estimation > experimental measurements) in several tissues, especially in fat.
For experimental PC values for phthalates, only few values were found. Our selection criteria for studies reporting experimental PC values were not fulfilled by a majority of the experimental studies on phthalate distribution. Specifically, the reasons for which experimental studies were excluded from the estimation of experimental PC values included one or more of the following: (1) application of a single dose and by consequence, no reach of the steady-state, (2) sampling of blood/plasma and tissues conducted 24 h after exposure, while the t1/2 values of phthalates in blood/plasma are lower than 24 h, (3) results presented as percentages of the dose in different tissues, instead of concentration units, or (4) no information on the phthalate concentrations in blood/plasma and in other tissues available from the same experimental treatment. To our knowledge, no previous review considered these criteria to analyze phthalate distribution at steady state. In our database, the values measured by different experimental approaches (in-vivo vs. in-vitro) were mutually coherent.
Regarding algorithmic PC values, we estimated them by using five algorithms, including that from Poulin and Krishnan (Citation1995b), used in PBPK models for phthalates (Keys et al. Citation1999, Citation2000; Clewell et al. Citation2008; Gentry et al. Citation2011). The selected algorithms estimate PC values based on the hydrophobicity of the compounds (passive partitioning). For this reason, we used the algorithms only for phthalates within the applicability domain of hydrophobicity of each algorithm. The algorithms were developed for organic substances that did not present a significant binding, ionization, or metabolism in the studied tissues. However, as shown in this review, a high binding of certain phthalates to plasma proteins and phthalate metabolism in different tissues, including plasma, have been reported. Here, we analyze how these processes (binding, metabolism) as well as ionization and passive partitioning of phthalates determine the distribution of these compounds. The presence of these processes, which contribute to the measured (experimental) PC values but were not considered in the algorithmic estimations, explains the important influence of the estimation method (algorithm vs. experiment) on the PC values for these compounds.
First, binding to plasma proteins is a major process to consider in phthalate distribution. Specifically, the high binding of some phthalates to plasma proteins explains a high retention of these compounds in plasma and a low transfer to tissues in experimental PC values. As a consequence, the experimental PC values are low (generally < 5) for all phthalates in the studied tissues and considerably lower than the algorithmic values. In the literature, a very high protein-bound fraction in plasma has been reported for DEHP and MEHP, while the protein-bound fraction in plasma is not known yet for a majority of phthalates. In principle, if we assume a direct relationship between protein-bound fraction in plasma and hydrophobicity (Poulin et al. Citation2001), we can suggest a high protein-bound fraction in plasma for a majority of phthalate diesters, with log Kow values above 4. More data on bound/unbound fractions of different phthalates (including also less hydrophobic parent phthalates and monoesters) in plasma/blood will be needed to confirm and quantify the influence of binding in the distribution of different phthalates.
Second, a high influence of ionization at physiological pH on phthalate distribution was shown by Keys et al. (Citation1999, Citation2000) for the monoesters MEHP and MnBP. Specifically, these authors estimated the PC values for these compounds both experimentally (in-vitro measurements) and by means of the algorithm of Poulin and Krishnan (Citation1995b). Additionally, they estimated algorithmic PC values that they corrected by considering the ionization of these monoesters at physiological pH. Specifically, this correction was made by considering a lower Kow value of these compounds at physiological pH, which was later used for ionization-corrected-algorithmic estimation. The algorithmic PC values corrected by ionization were much closer to the in-vitro values than the algorithmic PC values not corrected by ionization (). Specifically, in fat, after correction of the algorithmic PC values of MnBP and MEHP for ionization of these monoesters, the resulting values were respectively 1 order and 2 orders of magnitude higher than the in-vitro PC values (compared to 3 orders of magnitude without ionization-correction of the algorithmic values). The remaining differences between ionization-corrected-algorithmic and in-vitro PC values may be explained by additional factors. Specifically, Keys et al. (Citation1999, Citation2000) suggested that octanol would not be a reliable surrogate for biological lipids for relatively hydrophilic compounds. In this sense, Poulin and Krishnan (Citation1995b) and Payne and Kenny (Citation2002) showed that algorithmic and experimental PC values in fat were closer to each other for most compounds studied when the Kow was replaced in the algorithms by the partition coefficient for vegetable oil-to-saline (0.9% sodium chloride, Murphy et al. Citation1995). But the differences in the PC values in fat from using Kow vs. Kvegetable oil:saline remained within 1 order of magnitude (Poulin and Krishnan Citation1995b). Rather, as explained above, we suggest that the high binding to plasma proteins may explain the differences between ionization-corrected PC values and in-vitro PC values for MEHP and possibly MnBP.
Concerning phthalate passive partitioning and metabolism in the tissues, these processes may explain the differences in experimental PC values between phthalates. This effect is visible in the in-vivo PC values for compounds within the ‘phthalate group B’, i.e. from less hydrophobic metabolites MBP or MBzP to the more hydrophobic parent phthalate BBP, reported by Kim et al. (Citation2015) (, S2, S4, and S5, orange markers). First, passive partitioning may explain the experimental PC values for these compounds in fat and brain, since the PC values of the more hydrophobic BBP were clearly higher than for the less hydrophobic MBP and MBzP, with higher differences between parent BBP and its metabolites in fat (2 orders of magnitude) than in brain (5-fold). Second, the metabolism of phthalates in liver and kidney may explain the experimental PC values in these tissues, which were lower for the parent BBP than for its metabolites, notably in liver (1 order of magnitude). More experimental PC values for a given parent phthalate and its metabolites in different matrices (e.g. fat and liver) would be needed to compare the influence of passive partitioning and metabolism on the PC values for different phthalates.
Exposure route
The exposure route has an influence on phthalate bioavailability and elimination (intravenous and oral > dermal), first-pass metabolism (oral and dermal), early distribution (intravenous > oral), and biliary excretion (intravenous > oral and dermal). The high first-pass metabolism and probably delayed absorption of phthalates following oral and dermal exposure, which does not occur after intravenous exposure, may be at the origin of these results. In fact, after oral or dermal exposure, the parent phthalate stays for several hours in the gastrointestinal tract or skin, respectively, where it becomes bioavailable progressively, mainly as monoesters. For example, after oral exposure to radiolabelled DEHP or DIOP in rat, 65–85 and 40% of derived-radioactivity remained in the gastrointestinal tract (with contents) 8 h after exposure (Ikeda et al. Citation1978; Citation1980) and 12 h after exposure (Tanaka et al. Citation1975), respectively. Similarly, after dermal exposure to phthalates, a progressive absorption for more than 24 h was shown (Elsisi et al. Citation1989; Sugino et al. Citation2017). The monoesters become gradually bioavailable and can be progressively eliminated in urine and feces, without a high accumulation in the body. Conversely, after intravenous exposure, the intact diester directly reaches the systemic circulation and internal organs, including the liver. In this case, the parent compound stays for several hours in the liver and other tissues, where it is metabolized and may be partly excreted as metabolites in bile, urine, and feces. This may also explain why the biliary excretion of phthalates is higher after intravenous than after oral or dermal exposure.
In opposition to the influence of the exposure route on several pharmacokinetic steps, the pattern of metabolites in urine is generally not affected by the exposure route. This may be the consequence of the capacity of several tissues to metabolize phthalates, including skin (dermal exposure), gastrointestinal tract (oral exposure), liver, plasma, and other tissues (all exposure routes). These results suggest that, after intravenous exposure, when no first-pass metabolism occurs and the parent phthalate directly reaches the blood, phthalate metabolism in internal tissues can compensate for the lack of first-pass metabolism.
Physico-chemical properties (hydrophobicity)
The physico-chemical properties (hydrophobicity) of phthalates have a global influence on phthalate pharmacokinetics. First, as indicated above, phthalate hydrophobicity leads the passive partitioning of phthalates, which is one among four main processes which determine phthalate distribution. Second, less hydrophobic phthalate diesters generally have a higher absorption after oral and dermal exposure than more hydrophobic phthalates. Finally, less hydrophobic phthalates generally have slightly lower t1/2 values, simpler metabolism, higher enterohepatic recycling (related to a higher oral absorption), and higher urinary elimination than more hydrophobic ones.
Conclusions
To conclude, we explain how binding, ionization, passive partitioning, and metabolism influence phthalate distribution. The algorithms studied in this review for PC estimation were generally based only on passive partitioning, which explains the observed differences between experimental and algorithmic values reported here. Other algorithms exist, some of which include the processes of binding and ionization (Poulin and Theil Citation2000; Schmitt Citation2008; Peyret et al. Citation2010). However, these alternative algorithms require some physico-chemical properties, among others the unbound fractions of phthalates in plasma and/or other tissues, which are generally unavailable or scarce in the literature for most phthalates. More experimental data on bound fractions of different phthalates to proteins in blood/plasma are needed to quantify the influence of binding on the distribution of different phthalates. In opposition to the algorithms, the experimental measurements of PC values present a major advantage for phthalates, as these measurements include all main processes (i.e. binding, ionization, passive partitioning, and metabolism) that determine phthalate distribution. Therefore, we recommend to use a more general term for these ‘experimental PC values’, for example ‘distribution factors’, to indicate that several processes beyond passive partitioning determine these values. More experimental measurements of phthalate ‘distribution factors’ are needed, if possible for a given parent phthalate and its metabolites in different matrices (e.g. fat and liver) in order to compare the influence of metabolism and passive partitioning between phthalates. We recommend the interesting and relatively simple in-vitro approach applied by Murphy et al. (Citation1995) and Keys et al. (Citation1999, Citation2000). Also, in-vivo studies on phthalate partitioning (at steady state) in rat are rare and additional data would be useful to validate some in-vitro measurements.
Between routes of exposure, a majority of the phthalate pharmacokinetic data in the literature are for oral and intravenous exposure, whereas data for dermal exposure and especially after inhalation exposure are scarce. More experiments to compare (ideally within the same study) the pattern of metabolites in urine and total urinary elimination fractions between two routes of exposure, including either inhalation or dermal routes vs. either oral or intravenous routes, would be useful.
The results from this review provide a basis for the design of new PBPK models for these compounds. Additionally, the description of the influence of physico-chemical properties of phthalates on pharmacokinetics of these compounds and the classification of phthalates in ‘phthalate groups’ (Table S3) may be useful for further extrapolation of a PBPK model among phthalates and to comprehend the pharmacokinetics of a phthalate for which few data are available, when more is known about the pharmacokinetics of other compounds within the same group. For example, a PBPK model can be more easily extrapolated between parent compounds included within the same phthalate group. More broadly, on the basis of the physico-chemical properties, we can also make extrapolations between similar groups of compounds. For instance, many pharmacokinetic data are available for compounds within the groups A (DEHP) and B (BBP and DBP), whereas fewer data are generally available for other phthalate groups, including the groups C (DEP and DMP) and D (DIDP, DINP, DnOP, and DIOP). In principle, we can consider that the pharmacokinetics of compounds within group C may be similar to that of phthalates within group B, since both groups are among the less hydrophobic phthalates studied. Similarly, for more hydrophobic phthalates, in principle, we can consider the pharmacokinetics of compounds within group D to be similar to that of phthalates within group A. In all the cases, we would recommend that future experimental research on phthalate pharmacokinetics focus especially on the groups C and D, since these groups represent extremes in hydrophobicity among the studied groups.
SUPPLEMENTAL_MATERIAL_revised.pdf
Download PDF (2.1 MB)Acknowledgments
We thank Agnès Fournier (UR AFPA, Vandoeuvre-lès-Nancy, France), Klára Komprdová (RECETOX, Brno, Czech Republic), and David Konecny (RECETOX, Brno, Czech Republic) for their valuable comments on this manuscript.
Disclosure statement
The authors report no conflict of interest.
Additional information
Funding
References
- Abraham MH, Acree WE. 2015. Physicochemical and biochemical properties for the dialkyl phthalates. Chemosphere. 119:871–880.
- Albro PW, Corbett JT, Schroeder JL, Jordan S, Matthews HB. 1982. Pharmacokinetics, interactions with macromolecules and species-differences in metabolism of DEHP. Environ Health Perspect. 45:19–25.
- Albro PW, Hass JR, Peck CC, Jordan ST, Corbett JT, Schroeder J. 1982. Applications of isotope differentiation for metabolic studies with di-(2-ethylhexyl) phthalate. J Environ Sci Health. 17:701–714.
- Albro PW, Lavenhar SR. 1989. Metabolism of di(2-ethylhexyl)phthalate. Drug Metab Rev. 21:13–34.
- Alzaga R, Peña A, Bayona JM. 2003. Determination of phthalic monoesters in aqueous and urine samples by solid-phase microextraction-diazomethane on-fibre derivatization-gas chromatography-mass spectrometry. J Sep Sci. 26:87–96.
- Andersen C, Krais AM, Eriksson AC, Jakobsson J, Londahl J, Nielsen J, Lindh CH, Pagels J, Gudmundsson A, Wierzbicka A. 2018. Inhalation and dermal uptake of particle and gas-phase phthalates-A human exposure study. Environ Sci Technol. 52:12792–12800.
- Aylward LL, Hays SM, Gagne M, Krishnan K. 2009a. Derivation of biomonitoring equivalents for di(2-ethylhexyl)phthalate (CAS No. 117-81-7). Regul Toxicol Pharmacol. 55:249–258.
- Aylward LL, Hays SM, Gagne M, Krishnan K. 2009b. Derivation of biomonitoring equivalents for di-n-butyl phthalate (DBP), benzylbutyl phthalate (BzBP), and diethyl phthalate (DEP). Regul Toxicol Pharmacol. 55:259–267.
- Balaz S, Lukacova V. 1999. A model-based dependence of the human tissue/blood partition coefficients of chemicals on lipophilicity and tissue composition. Quant Struct Activity Relat. 18:361–368.
- Becker K, Goen T, Seiwert M, Conrad A, Pick-Fuss H, Muller J, Wittassek M, Schulz C, Kolossa-Gehring M. 2009. GerES IV: phthalate metabolites and bisphenol A in urine of German children. Int J Hyg Environ Health. 212:685–692.
- Buchta C, Bittner C, Hocker P, Macher M, Schmid R, Seger C, Dettke M. 2003. Donor exposure to the plasticizer di(2-ethylhexyl)phthalate during plateletpheresis. Transfusion. 43:1115–1120.
- Buxton ILO, Benet LZ. 2011. Pharmacokinetics: the dynamics of drug absorption, distribution, metabolism, and elimination, Goodman & Gilman’s the pharmacological basis of therapeutics. 12th ed. New York (NY): McGraw-Hill; p. 17–40.
- Chen L, Zhao Y, Li LX, Chen BH, Zhang YH. 2012. Exposure assessment of phthalates in non-occupational populations in China. Sci Total Environ. 427:60–69.
- Choi J, Knudsen LE, Mizrak S, Joas A. 2017. Identification of exposure to environmental chemicals in children and older adults using human biomonitoring data sorted by age: results from a literature review. Int J Hyg Environ Health. 220:282–298.
- Chu I, Villeneuve DC, Secours V, Franklin C, Rock G, Viau A. 1978. Metabolism and tissue distribution of mono-2-ethylhexyl phthalate in the rat. Drug Metab Dispos. 6:146–149.
- Clewell RA, Kremer JJ, Williams CC, Campbell JL, Andersen ME, Borghoff SJ. 2008. Tissue exposures to free and glucuronidated monobutylyphthalate in the pregnant and fetal rat following exposure to di-n-butylphthalate: evaluation with a PBPK model. Toxicol Sci. 103:241–259.
- Cousins I, Mackay D. 2000. Correlating the physical-chemical properties of phthalate esters using the ‘three solubility’ approach. Chemosphere. 41:1389–1399.
- Daniel JW, Bratt H. 1974. The absorption, metabolism and tissue distribution of di(2-ethylhexyl)phthalate in rats. Toxicology. 2:51–65.
- DeJongh J, Verhaar HJM, Hermens J. 1997. A quantitative property-property relationship (QPPR) approach to estimate in vitro tissue-blood partition coefficients of organic chemicals in rats and humans. Arch Toxicol. 72:17–25.
- [ECHA] European Chemicals Agency. 2018. Information on chemicals. Helsinki, Finland: European Chemicals Agency. [accessed 2018 Nov 6]. https://echa.europa.eu/information-on-chemicals.
- Eigenberg DA, Bozigian HP, Carter DE, Sipes IG. 1986. Distribution, excretion, and metabolism of butylbenzyl phthalate in the rat. J Toxicol Environ Health. 17:445–456.
- Elsisi AE, Carter DE, Sipes IG. 1989. Dermal absorption of phthalate diesters in rats. Fundam Appl Toxicol. 12:70–77.
- [EPA] United States Environmental Protection Agency. 2012. Estimation programs interface suite™ for microsoft® windows, v 4.11. Washington (DC): United States Environmental Protection Agency. [accessed 2018 Aug 22]. https://www.epa.gov/tsca-screening-tools/epi-suitetm-estimation-program-interface.
- Fay M, Donohue JM, De Rosa C. 1999. ATSDR evaluation of health effects of chemicals. VI. Di(2-ethylhexyl)phthalate. Agency for toxic substances and disease registry. Toxicol Ind Health. 15:651–746.
- Fiserova-Bergerova V, Diaz ML. 1986. Determination and prediction of tissue-gas partition coefficients. Int Arch Occup Environ Health. 58:75–87.
- Frederiksen H, Skakkebaek NE, Andersson AM. 2007. Metabolism of phthalates in humans. Mol Nutr Food Res. 51:899–911.
- Gentry PR, Clewell HJ, Clewell R, Campbell J, Van Landingham C, Shipp AM. 2011. Challenges in the application of quantitative approaches in risk assessment: a case study with di-(2-ethylhexyl)phthalate. Crit Rev Toxicol. 41:1–72.
- Guo Y, Alomirah H, Cho HS, Minh TB, Mohd MA, Nakata H, Kannan K. 2011. Occurrence of phthalate metabolites in human urine from several Asian countries. Environ Sci Technol. 45:3138–3144.
- Hines CJ, Hopf NBN, Deddens JA, Calafat AM, Silva MJ, Grote AA, Sammons DL. 2009. Urinary phthalate metabolite concentrations among workers in selected industries: a pilot biomonitoring study. Ann Occup Hyg. 53:1–17.
- Hopf NB, Berthet A, Vernez D, Langard E, Spring P, Gaudin R. 2014. Skin permeation and metabolism of di(2-ethylhexyl) phthalate (DEHP). Toxicol Lett. 224:47–53.
- Huygh J, Clotman K, Malarvannan G, Covaci A, Schepens T, Verbrugghe W, Dirinck E, Van Gaal L, Jorens PG. 2015. Considerable exposure to the endocrine disrupting chemicals phthalates and bisphenol-A in intensive care unit (ICU) patients. Environ Int. 81:64–72.
- Ikeda GJ, Sapienza PP, Couvillion JL, Farber TM, Smith CP, Inskeep PB, Marks EM, Cerra FE, van Loon EJ. 1978. Distribution and excretion of two phthalate esters in rats, dogs and miniature pigs. Food Cosmet Toxicol. 16:409–413.
- Ikeda GJ, Sapienza PP, Couvillion JL, Farber TM, Vanloon EJ. 1980. Comparative distribution, excretion and metabolism of di-(2-ethylhexyl) phthalate in rats, dogs and miniature pigs. Food Cosmet Toxicol. 18:637–642.
- Jaeger RJ, Rubin RJ. 1973. Extraction, localization, and metabolism of Di-2-ethylhexyl phthalate from PVC plastic medical devices. Environ Health Perspect. 3:95–102.
- Johns LE, Ferguson KK, McElrath TF, Mukherjee B, Meeker JD. 2016. Associations between repeated measures of maternal urinary phthalate metabolites and thyroid hormone parameters during pregnancy. Environ Health Perspect. 124:1808–1815.
- Kademoglou K, Giovanoulis G, Palm-Cousins A, Padilla-Sanchez JA, Magner J, de Wit CA, Collin CD. 2018. In vitro inhalation bioaccessibility of phthalate esters and alternative plasticizers present in indoor dust using artificial lung fluids. Environ Sci Technol Lett. 5:329–334.
- Kasper-Sonnenberg M, Koch HM, Wittsiepe J, Wilhelm M. 2012. Levels of phthalate metabolites in urine among mother-child-pairs - Results from the Duisburg birth cohort study, Germany. Int J Hyg Environ Health. 215:373–382.
- Kastury F, Smith E, Juhasz AL. 2017. A critical review of approaches and limitations of inhalation bioavailability and bioaccessibility of metal(loid)s from ambient particulate matter or dust. Sci Total Environ. 574:1054–1074.
- Kavlock R, Boekelheide K, Chapin R, Cunningham M, Faustman E, Foster P, Golub M, Henderson R, Hinberg I, Little R, et al. 2002a. NTP center for the evaluation of risks to human reproduction: phthalates expert panel report on the reproductive and developmental toxicity of butyl benzyl phthalate. Reprod Toxicol. 16:453–487.
- Kavlock R, Boekelheide K, Chapin R, Cunningham M, Faustman E, Foster P, Golub M, Henderson R, Hinberg I, Little R, et al. 2002b. NTP center for the evaluation of risks to human reproduction: phthalates expert panel report on the reproductive and developmental toxicity of di(2-ethylhexyl) phthalate. Reprod Toxicol. 16:529–653.
- Kavlock R, Boekelheide K, Chapin R, Cunningham M, Faustman E, Foster P, Golub M, Henderson R, Hinberg I, Little R, et al. 2002c. NTP center for the evaluation of risks to human reproduction: phthalates expert panel report on the reproductive and developmental toxicity of di-isononyl phthalate. Reprod Toxicol. 16:679–708.
- Kavlock R, Boekelheide K, Chapin R, Cunningham M, Faustman E, Foster P, Golub M, Henderson R, Hinberg I, Little R, et al. 2002d. NTP center for the evaluation of risks to human reproduction: phthalates expert panel report on the reproductive and developmental toxicity of di-n-butyl phthalate. Reprod Toxicol. 16:489–527.
- Kavlock R, Boekelheide K, Chapin R, Cunningham M, Faustman E, Foster P, Golub M, Henderson R, Hinberg I, Little R, et al. 2002e. NTP center for the evaluation of risks to human reproduction: phthalates expert panel report on the reproductive and developmental toxicity of di-n-hexyl phthalate. Reprod Toxicol. 16:709–719.
- Kavlock R, Boekelheide K, Chapin R, Cunningham M, Faustman E, Foster P, Golub M, Henderson R, Hinberg I, Little R, et al. 2002f. NTP center for the evaluation of risks to human reproduction: phthalates expert panel report on the reproductive and developmental toxicity of di-n-octyl phthalate. Reprod Toxicol. 16:721–734.
- Kavlock R, Boekelheide K, Chapin R, Cunningham M, Faustman E, Foster P, Golub M, Henderson R, Hinberg I, Little R, et al. 2002g. NTP center for the evaluation of risks to human reproduction: phthalates expert panel report on the reproductive and developmental toxicity of di-isodecyl phthalate. Reprod Toxicol. 16:655–678.
- Kessler W, Numtip W, Grote K, Csanady GA, Chahoud I, Filser JG. 2004. Blood burden of di(2-ethylhexyl) phthalate and its primary metabolite mono(2-ethylhexyl) phthalate in pregnant and nonpregnant rats and marmosets. Toxicol Appl Pharmacol. 195:142–153.
- Keys DA, Wallace DG, Kepler TB, Conolly RB. 1999. Quantitative evaluation of alternative mechanisms of blood and testes disposition of di(2-ethylhexyl) phthalate and mono(2-ethylhexyl) phthalate in rats. Toxicol Sci. 49:172–185.
- Keys DA, Wallace DG, Kepler TB, Conolly RB. 2000. Quantitative evaluation of alternative mechanisms of blood disposition of di(n-butyl) phthalate and mono(n-butyl) phthalate in rats. Toxicol Sci. 53:173–184.
- Kim MG, Kim TH, Shin BS, Lee YB, Lee JB, Choi HG, Lee Y, Yoo SD. 2015. Sensitive liquid chromatography-tandem mass spectrometry method for the simultaneous determination of benzyl butyl phthalate and its metabolites, monobenzyl phthalate and monobutyl phthalate, in rat plasma, urine, and various tissues collected from a toxicokinetic study. Anal Bioanal Chem. 407:7391–7400.
- Kim S, Thiessen PA, Bolton EE, Chen J, Fu G, Gindulyte A, Han LY, He JE, He SQ, Shoemaker BA, et al. 2016. PubChem substance and compound databases. Nucleic Acids Res. 44:D1202–D1213.
- Kluwe WM. 1982. Overview of phthalate ester pharmacokinetics in mammalian species. Environ Health Perspect. 45:3–10.
- Knudsen GA, Sanders JM, Birnbaum LS. 2017. Disposition of the emerging brominated flame retardant, bis(2-ethylhexyl) tetrabromophthalate, in female Sprague Dawley rats: effects of dose, route and repeated administration. Xenobiotica. 47:245–254.
- Koch HM, Bolt HM, Preuss R, Angerer J. 2005. New metabolites of di(2-ethylhexyl)phthalate (DEHP) in human urine and serum after single oral doses of deuterium-labelled DEHP. Arch Toxicol. 79:367–376.
- Koch HM, Bolt HM, Preuss R, Eckstein R, Weisbach V, Angerer J. 2005. Intravenous exposure to di(2-ethylhexyl)phthalate (DEHP): metabolites of DEHP in urine after a voluntary platelet donation. Arch Toxicol. 79:689–693.
- Koch HM, Drexler H, Angerer J. 2004. Internal exposure of nursery-school children and their parents and teachers to di(2-ethylhexyl)phthalate (DEHP)). Int J Hyg Environ Health. 207:15–22.
- Krais AM, Andersen C, Eriksson AC, Johnsson E, Nielsen J, Pagels J, Gudmundsson A, Lindh CH, Wierzbicka A. 2018. Excretion of urinary metabolites of the phthalate esters DEP and DEHP in 16 volunteers after inhalation and dermal exposure. Int J Environ Res Public Health. 15: E2514.
- Liberati A, Altman DG, Tetzlaff J, Mulrow C, Gotzsche PC, Ioannidis JPA, Clarke M, Devereaux PJ, Kleijnen J, Moher D. 2009. The PRISMA statement for reporting systematic reviews and meta-analyses of studies that evaluate health care interventions: explanation and elaboration. Ann Int Med. 151:W65–W94.
- Lu CH. 2009. Prediction of environmental properties in water-soil-air systems for phthalates. Bull Environ Contam Toxicol. 83:168–173.
- McKee RH, El-Hawari M, Stoltz M, Pallas F, Lington AW. 2002. Absorption, disposition and metabolism of di-isononyl phthalate (DINP) in F-344 rats. J Appl Toxicol. 22:293–302.
- Mose T, Mortensen GK, Hedegaard M, Knudsen LE. 2007. Phthalate monoesters in perfusate from a dual placenta perfusion system, the placenta tissue and umbilical cord blood. Reprod Toxicol. 23:83–91.
- Murphy JE, Janszen DB, Gargas ML. 1995. An in-vitro method for determination of tissue partition-coefficients of nonvolatile chemicals such as 2,3,7,8-tetrachlorodibenzo-p-dioxin and estradiol. J Appl Toxicol. 15:147–152.
- [NIH] National Institutes of Health. 2018. US national library of medicine, TOXNET (toxicology data network), ChemIDplus. Bethesda (MD): National Institutes of Health. [accessed 2018 Aug 22]. https://www.ncbi.nlm.nih.gov/pccompound.
- Payan JP, Marty JP, Fabry JP, Beydon D, Boudry I, Ferrari E, Canel F, Grandclaude MC, Vincent CM. 2001. In vivo and in vitro percutaneous absorption of [(14)C]di-N-butylphthalate in rat. Drug Metab Dispos. 29:843–854.
- Payne MP, Kenny LC. 2002. Comparison of models for the estimation of biological partition coefficients. J Toxicol Environ Health Part A. 65:897–931.
- Peyret T, Poulin P, Krishnan K. 2010. A unified algorithm for predicting partition coefficients for PBPK modeling of drugs and environmental chemicals. Toxicol Appl Pharmacol. 249:197–207.
- Poulin P, Krishnan K. 1995a. A biologically-based algorithm for predicting human tissue – blood partition coefficients of organic chemicals. Hum Exp Toxicol. 14:273–280.
- Poulin P, Krishnan K. 1995b. An algorithm for predicting tissue:blood partition coefficients of organic chemicals from n-octanol:water partition coefficient data. J Toxicol Environ Health. 46:117–129.
- Poulin P, Schoenlein K, Theil FP. 2001. Prediction of adipose tissue: plasma partition coefficients for structurally unrelated drugs. J Pharm Sci. 90:436–447.
- Poulin P, Theil FP. 2000. A Priori prediction of tissue: plasma partition coefficients of drugs to facilitate the use of physiologically-based pharmacokinetic models in drug discovery. J Pharm Sci. 89:16–35.
- Salim CJ, Liu H, Kennedy JF. 2010. Comparative study of the adsorption on chitosan beads of phthalate esters and their degradation products. Carbohydr Polym. 81:640–644.
- Schmitt W. 2008. General approach for the calculation of tissue to plasma partition coefficients. Toxicol in Vitro. 22:457–467.
- Schulz CO, Rubin RJ. 1973. Distribution, metabolism, and excretion of Di-2-ethylhexyl phthalate in the rat. Environ Health Perspect. 3:123–129.
- Scott RC, Dugard PH, Ramsey JD, Rhodes C. 1987. In vitro absorption of some ortho-phthalate diesters through human and rat skin. Environ Health Perspect. 74:223–227.
- Sharma RP, Schuhmacher M, Kumar V. 2018. Development of a human physiologically based pharmacokinetic (PBPK) model for phthalate (DEHP) and its metabolites: a bottom up modeling approach. Toxicol Lett. 296:152–162.
- Shintani H. 2000. Pretreatment and chromatographic analysis of phthalate esters, and their biochemical behavior in blood products. Chromatographia. 52:721–726.
- Silva MJ, Furr J, Preau JL, Samandar E, Gray LE, Calafat AM. 2012. Identification of potential biomarkers of exposure to di(isononyl)cyclohexane-1,2-dicarboxylate (DINCH), an alternative for phthalate plasticizers. J Expo Sci Environ Epidemiol. 22:204–211.
- Song NR, On JW, Lee J, Park JD, Kwon HJ, Yoon HJ, Pyo H. 2013. Biomonitoring of urinary di(2-ethylhexyl) phthalate metabolites of mother and child pairs in South Korea. Environ Int. 54:65–73.
- Sugino M, Hatanaka T, Todo H, Mashimo Y, Suzuki T, Kobayashi M, Hosoya O, Jinno H, Juni K, Sugibayashi K. 2017. Safety evaluation of dermal exposure to phthalates: metabolism-dependent percutaneous absorption. Toxicol Appl Pharmacol. 328:10–17.
- Tanaka A, Adachi T, Takahashi T, Yamaha T. 1975. Biochemical studies on phthalic esters. 1. Elimination, distribution and metabolism of di-(2-ethylhexyl)phthalate in rats. Toxicology. 4:253–264.
- Wang YP, Zhang GW. 2015. Spectroscopic and molecular simulation studies on the interaction of di-(2-ethylhexyl) phthalate and human serum albumin. Luminescence. 30:198–206.
- White RD, Carter DE, Earnest D, Mueller J. 1980. Absorption and metabolism of three phthalate diesters by the rat small intestine. Food Cosmet Toxicol. 18:383–386.
- Williams DT, Blanchfield BJ. 1975. The retention, distribution, excretion, and metabolism of dibutyl phthalate-7-14 C in the rat. J Agric Food Chem. 23:854–858.
- Wormuth M, Scheringer M, Vollenweider M, Hungerbuhler K. 2006. What are the sources of exposure to eight frequently used phthalic acid esters in Europeans? Risk Anal. 26:803–824.
- Xie XY, Wang ZW, Zhou XM, Wang XR, Chen XG. 2011. Study on the interaction of phthalate esters to human serum albumin by steady-state and time-resolved fluorescence and circular dichroism spectroscopy. J Hazard Mater. 192:1291–1298.
- Yuan D, Shen ZL, Liu RT, Wei PH, Gao CZ. 2014. Toxic interaction between dibutyl phthalate and human serum albumin: spectroscopic and molecular modeling investigations. J Chin Chem Soc. 61:255–262.
- Yue YY, Liu JM, Liu R, Sun YY, Li XG, Fan J. 2014. The binding affinity of phthalate plasticizers-protein revealed by spectroscopic techniques and molecular modeling. Food Chem Toxicol. 71:244–253.
- Zhao HZ, Li JF, Zhou YQ, Zhu L, Zheng YY, Xia W, Li YY, Xiang L, Chen W, Xu SQ, et al. 2018. Investigation on metabolism of di(2-ethylhexyl) phthalate in different trimesters of pregnant women. Environ Sci Technol. 52:12851–12858.
- Zota AR, Calafat AM, Woodruff TJ. 2014. Temporal trends in phthalate exposures: findings from the national health and nutrition examination survey, 2001–2010. Environ Health Perspect. 122:235–241.