Abstract
The aim of this study was the evaluation of the influence of bile salts and fatty acids, important components of intestinal fluids, on physical characteristics of self-emulsifying drug delivery systems (SEDDS) such as size, polydispersity (PDI), zeta potential (Zp), turbidity (T%), cloud point temperature (CPT) and drug release. At this purpose, nonionic (ni-SEDDS) and cationic (c-SEDDS) were emulsified in aqueous media containing increasing concentrations of bile salts (BS) and decanoate (Dec). Zp of ni-SEDDS and c-SEDDS became highly negative at 15 mM BS and Dec. Size of ni-SEDDS decreased of 112 nm and of 76 nm at 15 mM BS and Dec, respectively. Size of c-SEDDS decreased of 53 nm at 15 mM BS, but it was not affected by 15 mM Dec. PDI and T% of ni- and c-SEDDS were lowered as well. CPT of ni-SEDDS increased from 70 °C to 97 °C and 84 °C at 15 mM BS and Dec. CPT of c-SEDDS decreased from above 100 °C to 80 °C and to 85 °C at 1.5 mM BS and at 5 mM Dec, respectively. Generally, BS had a more pronounced effect on SEDDS Zp, size, PDI, T %, and CPT than Dec. The release of the model drug quinine was accelerated by BS and Dec. As BS and fatty acids affect the physical characteristics and drug release behavior of SEDDS, their impact should be addressed during the development process.
Introduction
Self-emulsifying drug delivery systems (SEDDS) are innovative lipid-based formulations consisting of isotropic mixtures of oils, surfactants, and co-solvents forming fine, and stable oil-in-water o/w emulsions upon contact with aqueous media such as gastrointestinal fluid. Due to their composition SEDDS belong to type III of lipid classification system [Citation1]. SEDDS offer the advantage to be easy to produce and scale up. Furthermore, SEDDS preconcentrates can be loaded in capsules and administered orally. In the last years, SEDDS are gaining more and more attention as carriers for delivery of hydrophobic ion pairs (HIPs) formed between drugs and lipophilic counter ions [Citation2,Citation3]. Numerous recent publications reporting in vivo data demonstrate that SEDDS are particularly effective in improving oral bioavailability of HIPs [Citation4–6]. Exenatide ion paired with sodium docusate and loaded in SEDDS showed a relative bioavailability of 14% [Citation7]. The oral bioavailability of drugs, in particular peptides, is promoted on the one hand by the protective effect of SEDDS toward a presystemic metabolism and on the other hand by the dispersion of the drug in fine oily droplets that can easily permeate the mucus layer covering the intestinal mucosa reaching the absorption site [Citation8–10]. In particular, SEDDS characterized by small size (<200nm) and negative zeta potential permeates the mucus more efficiently due to reduced interaction with negatively charged mucins of the mucus gel layer [Citation11,Citation12]. As SEDDS are rapidly degraded by lipases in the intestine [Citation3,Citation5] their stability toward other components of the intestinal fluid was so far not investigated. More recently, SEDDS stable toward lipases were developed [Citation13,Citation14] showing a comparatively higher protective effect for orally delivered peptide drugs. Key properties such as size, zeta potential, or polydispersity, however, might be also substantially altered by other components of the intestinal fluid such as bile salts and fatty acids. Indeed, previous studies demonstrated that the structure of other lipophilic formulations such as liposomes, lipophilic nanoparticles, and emulsions can be altered by bile salts [Citation15–17]. Such altered physical properties have in turn a substantial impact on the in vivo performance of drug delivery systems. The extraction of emulsifiers and lipids from liposomes, for instance, caused formulation instability, dose dumping, and a decrease in bioavailability [Citation15–18]. In another study, an enhanced intestinal absorption of lipophilic drug-loaded nanocarriers was shown in the presence of bile salts [Citation19].
As the impact of bile salts and fatty acids on SEDDS has so far not been evaluated, it was the aim of our study to evaluate their effect on SEDDS properties. At this purpose, a variety of nonionic and cationic SEDDS were prepared, emulsified in dissolution media containing bile salts and sodium decanoate and characterized regarding zeta potential, size, stability, polydispersity, transmittance, cloud point temperature and drug release.
Materials and methods
Materials
Bile salts (sodium cholate, sodium deoxycholate 1:1), sodium cholate, sodium taurocholate, sodium decanoate, Tween 80, DMSO, Cremophor EL, glycerol 85%, Transcutol, ethyl oleate, dioctadecyldimethylammonium chloride (DDAC) were purchased from Sigma-Aldrich (Vienna, Austria). Palmitamidopropyltrimonium chloride (PPTC) was bought from Evonik (Hamburg, Germany). Peceol was a gift from Gattefossé (Lyon, France), whereas Capmul PG8, Labrafil M 1944 CS, Capmul MCM and Captex 355 were a gift from Abitec (St Janesville, WI, USA). Miglyol 840 was bought from Sasol (Hamburg, Germany).
Methods
Preparation of bile salts and medium-chain fatty acid media
The bile salts sodium cholate, sodium deoxycholate, and sodium taurocholate (1:1:1 molar ratio) were dissolved in demineralized water at a final concentration of 1.5, 5, 9, and 15 mM. Sodium decanoate was dissolved in demineralized water at a concentration of 0.1, 0.5, 5, 15, and 25 mM. 10 mM of NaCl were added to each bile salt and decanoate solution and pH was adjusted to 6.8 using 0.01 M HCl or 0.01 M NaOH, respectively. Sodium cholate and sodium deoxycholate were chosen as representatives of the class of unconjugated bile salts [Citation20] while sodium taurocholate was chosen as conjugated bile salt [Citation16]. Out of medium-chain fatty acids sodium decanoate was chosen because of its pronounced hydrophilic character still dissolving in aqueous media.
Preparation of SEDDS
Nonionic SEDDS preconcentrates (ni-SEDDS) were prepared by mixing oils, surfactants and co-solvents as listed in [Citation5,Citation21,Citation22]. Cationic SEDDS preconcentrates (c-SEDDS) were prepared by dissolving the cationic surfactants dioctadecyldimethylammonium chloride (DDAC) and palmitamidopropylammonium chloride (PPTC) in the most lipophilic excipients of each formulation (Peceol, Captex 355, Miglyol 840, Capmul MCM and ethyl oleate) at 70 °C and by mixing them with the remaining excipients as reported in . Ni- and c-SEDDS preconcentrates were emulsified in demineralized water, bile salts and decanoate solutions at a concentration of 0.5% (m/v). Solutions containing both bile salts and sodium decanoate were excluded because of high conductivity interfering with zeta potential measurements.
Table 1. Composition (w/w %) of nonionic formulations.
Table 2. Composition (w/w %) of cationic SEDDS.
Zeta potential, size and polydispersity determination
Zeta potential of formulations was measured at 37 °C in triplicate using a disposable cell (DTSI070, Malvern). Droplet size and polydispersity (PDI) was determined via dynamic light scattering technique at a fixed scattering angle of 173° (zetasizer ZPS, Malvern). The measurements were performed at 37 °C in triplicate. Droplet size is expressed as Z-average, namely the mean hydrodynamic diameter of the particle calculated from the cumulative analysis of the intensity of the scattered light. Formulation stability was evaluated via droplet size and zeta potential measurement after 4 h incubation at 37 °C under shaking at 350 rpm.
Transmittance determination
Transmittance (%) of ni- and c-SEDDS was measured using a UV-vis spectrophotometer at a wavelength of 600 nm (Shimadzu). The measurements were performed at room temperature using a 700 µl quartz cuvette. Solutions containing bile salts and decanoate were considered as 100%.
Cloud point temperature determination
Ni- and c-SEDDS were heated up to 100 °C while shaking at 350 rpm on a thermomixer (Eppendorf, Germany). Cloud point temperature of SEDDS formulations was evaluated visually and recorded when the formulation turned white. Cloud point temperature higher than 100 °C were not determined experimentally [Citation23].
Release study
To investigate drug release from SEDDS in the presence of bile salts and decanoate, we employed a biphasic release setup [Citation24] with some modifications to ensure sink conditions without an extensive dilution of SEDDS. Quinine, a lipophilic drug with a logP value of 2.9 (octanol/water) was used as a model drug. It was loaded into SEDDS at 10% w/w. Subsequently, quinine loaded SEDDS formulations were diluted 1:100 in water and in aqueous solution containing 15 mM bile salts and 25 mM decanoate. The pH of the solution was adjusted to 7.4 with 1 M HCl. One mL of each dilution was added to a 5ml test tube followed by the addition of 2 ml of 1-decanol. The system consisted of two phases: decanol as the upper one and diluted SEDDS as the lower one. Drug was continuously released from SEDDS and diffused to decanol phase until reaching equilibrium. The solubility of quinine in decanol was above 5 mg/mL ensuring sink conditions. Test tubes were gently shaken at 400 rpm and 37 °C on a Thermomixer (Thermomixer C, Eppendorf, Germany). At predetermined time points (15, 30, 60, 120, 180, and 240 min) aliquots of 20 µL were withdrawn from the decanol phase and substituted by the same volume of fresh decanol. Withdrawn samples were diluted 1:5 with decanol and quinine content was quantified via fluorescence measurements at the excitation/emission wavelength of 350/455 nm (Tecan Spark, Austria) using a standard curve of quinine in the range of 0-125 µg/mL.
Statistical analysis
The statistical data analysis was performed using the software GraphPad Prism 5. The means were compared using the analysis of variance (ANOVA) (α = 0.05) with Bonferroni's test as post hoc multiple comparison test. Level of p ≤ 0.05 was set for significant (*), p ≤0.01 for very significant (**) and p ≤0.001 (***) for highly significant. The results were expressed as the mean of at least three experiments ± standard deviation (SD).
Results and discussion
Sedds preparation
To evaluate the effect of bile salts and sodium decanoate on SEDDS a variety of formulations were prepared. According to classification of lipid delivery systems described by Pouton et al., formulations reported in and can be classified as type III [Citation25]. Formulations emulsified in water at a concentration of 0.5% m/v displayed a zeta potential from −7 to +30 mV, a size ranging from 50 nm to 220 nm and a PDI from 0.05–0.5. All formulations were stable in water over 4 h at 37 °C. The amount of cationic surfactants (3–6% m/m) used to prepare SEDDS was tailored to achieve comparable zeta potential among the formulations and to obtain narrow zeta potential distributions. Both chosen surfactants dioctadecyldimethylammonium chloride (DDAC) and palmitamidopropylammonium chloride (PPTC) being quaternary ammonium salts conferred SEDDS with a pH-independent charge. However, charge of SEDDS does not directly correlate to the concentration of these surfactants. This might be on the one hand due to different SEDDS compositions and on the other hand related to the structure of the two cationic surfactants (). SEDDS presented in this study are all consisting of a variety of PEG-ylated excipients, which might partially stealth the charges of the surfactants. The magnitude of stealth effect of PEG-ylated excipients on positive charges of DDAC and PPTC might be influenced by the arrangement of the cationic surfactants on SEDDS surface, which in turn depends on their structure and hydrophobicity.
Table 3. Chemical structure of cationic surfactants incorporated in SEDDS.
Zeta potential, size and polydispersity determination in presence of bile salts
Bile salts (BS) represent 25–35% of bile and are surface active molecules involved in the intestinal metabolism and absorption of lipids. Bile salts differ from commonly intended surfactants due to their cholesterol-based structure. Cholesterol structure is planar, whereas sterol rings of bile salts are bent. Moreover, bile salts are characterized by a bi-planar structure with hydrophobic moieties (CH3) on one face and the hydrophilic one (OH) on the opposite face of the sterol skeleton. This particular disposition of hydrophobic and hydrophilic groups is responsible for the amphiphilic nature of bile salts and consequently for their tendency to form micelles. To evaluate bile salts influence on SEDDS zeta potential, size and PDI, aqueous solutions containing increasing concentrations of bile salts covering the physiological range in the small intestine from 5 mM at fasted state to 15 mM at fed state were tested [Citation26]. As illustrated in () bile salts had a profound impact on zeta potential of both ni- and c-SEDDS. At increasing concentrations of bile salts, zeta potential of ni- and c-SEDDS decreased dramatically. A significant decrease in zeta potential of both sets of formulations was observed already at a bile salts concentration of 1.5 mM (p < 0.01), which is even below the intestinal bile salts concentration at fasted state. At simulated fasted state (5 mM BS) ni-SEDDS displayed an average decrease in zeta potential from −5 ± 2 mV to −18 ± 5 mV whereas the average zeta potential of c-SEDDS decreased from +22 ± 5 mV to −16 ± 4 mV. At simulated fed state (15 mM BS) ni-SEDDS F3 and F5 did not display a significant additional decrease in zeta potential with exception of formulation F1, F2 and F4 whose zeta potential further decreased from −24 ± 1 mV at 5 mM BS to −29 ± 1 mV at 15 mM BS (p < 0.01), from −18.9 ± 1 mV at 5 mM BS to −23.8 ± 1 mV at 15 mM BS (p < 0.01) and from −14 ± 1 mV at 5 mM BS to −20 ± 1 mV at 15 mM BS, respectively (p < 0.001). On the contrary, c-SEDDS displayed all a significant further decrease in zeta potential at fed state. Indeed, the average zeta potential of c-SEDDS decreased from −16 ± 4 mV at 5 mM BS to −28 ± 2 mV at 15 mM BS (p < 0.001). The observed decrease in zeta potential in presence of bile salts was likely caused by their integration in the surface of SEDDS increasing the negative charge. The measured zeta potential was stable over 4 h (data not shown). Decrease in zeta potential of lipid-based formulations in presence of bile salts was also reported in previous studies [Citation27–29]. Even though it is well known that in vivo bile salts absorb to lipids, the mechanism of absorption is still not fully understood. Some studies support the theory that due to their flat and rigid structure, bile salts absorb at the interface oil/water with the face carrying the hydrophobic moieties directed toward the oil phase and the hydrophilic face interacting with the water phase via hydrogen bonds. This theory is supported by calculations provided by Tiss et al. regarding the area occupied by bile salt molecule at the phospholipids/water interface that suggests a flat orientation of bile salts at the interface [Citation30]. However, some other studies provide data for a possible disposition of bile salts perpendicularly at the interface. Vadnere and Lindenbaum studied the partitioning of bile salts between water and n-octanol. Their results suggest that bile salts associate at the interface adopting a perpendicular orientation [Citation31,Citation32]. Other studies support the theory that the orientation of bile salts depends on the degree of order of the hydrophobic phase. Posă et al. studied the orientation of bile salts in mixed micelles consisting of bile salts and Tween 80 showing that bile salts can orientate tangentially at the surface of the mixed micelles with the hydrophobic face turned to the hydrophobic core and the hydrophilic face interacting with the hydrophilic head of Tween 80 or adopt a radial orientation in form of dimers [Citation33]. In case of SEDDS stabilized by hydrophilic surfactants such as Tween 80 or Cremophor EL, it is probable that bile salts adopt either of these two orientations. Size and polydispersity index (PDI) of ni-SEDDS in absence and presence of bile salts are reported in and Citation3(A) (). Depending on SEDDS composition the effect of bile salts on size and PDI was more or less pronounced. Size of ni-SEDDS F2, F3 and F4 decreased by just few nanometers in case of all tested bile salt concentrations (p > 0.05). Even though size of these SEDDS did not change significantly in presence of bile salts, at simulated fasted state (BS 5 mM) and fed state (BS 15 mM) F3 and F4 displayed a significantly lower PDI than in water (p < 0.001). F2 displayed a significant decrease in PDI only at bile salts concentration of 15 mM (p < 0.001). On the contrary, size and PDI of ni-SEDDS F1 and F5 were both affected by bile salts. Size of formulation F1 decreased from 225 ± 10 nm to 165 ± 1 nm at BS 5 mM and to 115 ± 5 nm at BS 15 mM (p < 0.001 BS 5 mM vs BS 15 mM) with a corresponding decrease in PDI from 0.40 ± 0.07 to 0.33 ± 0.01 at BS 5 mM and to 0.27 ± 0.01 at BS 15 mM. As illustrated in formulation F5 exhibiting a monomodal size distribution when emulsified in water with a Z-average of 160 ± 4 nm and PDI of 0.3 ± 0.01, displayed a size distribution with two distinct populations with diameter of 20 nm (3% intensity) and 140 nm (9% intensity) (PDI = 0.6 ± 0.01) at bile salts concentration of 5 mM. At BS 15 mM size distribution of formulation F5 became again monomodal with a Z-average of 13 ± 1 nm (17% intensity) and PDI of 0.09 ± 0.001. This remarkable change in size might be due to rearrangement of SEDDS emulsion excipients in small mixed micelles. Even though SEDDS are easy to prepare they are considered quite complex systems whose properties have not yet been fully disclosed and understood. Small changes in their composition might have a substantial impact on their emulsifying properties, stability and fate in the GI-tract. Therefore, it is quite difficult to anticipate which SEDDS might behave like F5. Among c-SEDDS only F6 was significantly affected by bile salts in terms of both size and PDI ( and , ). Size of formulation F6 decreased from 170 ± 8 nm to 130 ± 1 nm at BS 5 mM and to 115 ± 5 nm at BS 15 mM with a corresponding decrease in PDI from 0.5 ± 0.01 to 0.3 ± 0.001 at BS 5 mM and to 0.28 ± 0.001 at BS 15 mM. F7, F8 and F10 displayed a constant size and PDI in absence and presence of bile salts. However, F9 faced a decrease in PDI without a decrease in size. Size and PDI of ni- and c-SEDDS emulsified in bile salts remained unchanged over 4 h (data not shown).
Figure 1. (A–D) Zeta potential (mV) of nonionic (A) and cationic (B) SEDDS (0.5% m/v) emulsified in water containing indicated concentrations of bile salts; Zeta potential of nonionic (C) and cationic (D) SEDDS (0.5% m/v) emulsified in water containing indicated concentrations of sodium decanoate. Indicated values are means ± SD (n = 3). (Two way ANOVA vs water, * p < 0.05, ** p < 0.01, *** p < 0.001).
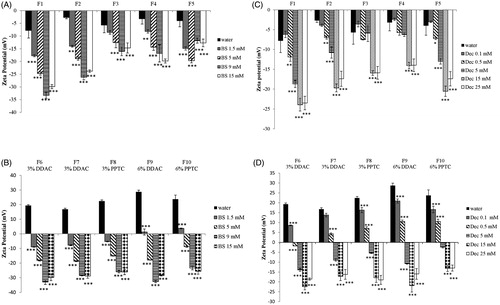
Figure 2. (A–D) Size (nm) of nonionic (A) and cationic (B) SEDDS (0.5% m/v) emulsified in water containing indicated concentrations of bile salts; Size (nm) of nonionic (C) and cationic (D) SEDDS (0.5% m/v) emulsified in water containing indicated concentrations of sodium decanoate. Indicated values are means ± SD (n = 3). (Two way ANOVA vs water, * p < 0.05, ** p < 0.01, *** p < 0.001).
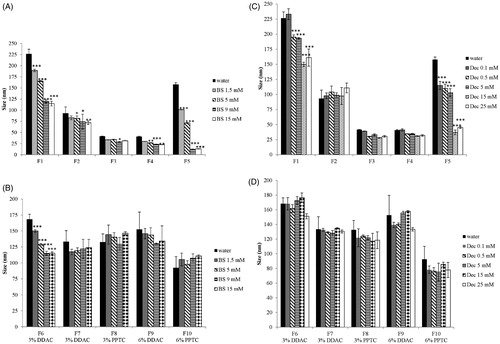
Figure 3. (A –D) PDI of nonionic (A) and cationic (B) SEDDS (0.5% m/v) emulsified in water containing indicated concentrations of bile salts; PDI of nonionic (C) and cationic (D) SEDDS (0.5% m/v) emulsified in water containing indicated concentrations of sodium decanoate. Indicated values are means ± SD (n = 3). (Two way ANOVA vs water, * p < 0.05, ** p < 0.01, *** p < 0.001).
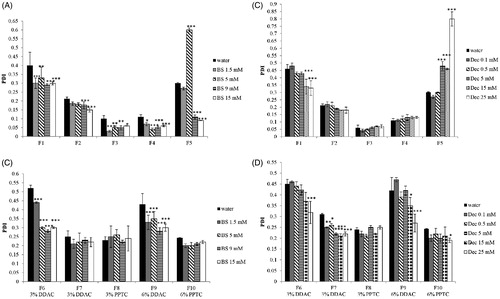
Zeta potential, size and polydispersity determination in presence of sodium decanoate
Intestinal lumen is characterized by variable amounts of fatty acids. Free fatty acids can reach the intestine as component of bile or can derive from the metabolism of ingested triglycerides. Medium-chain fatty acids (C8-12) such as decanoate are recognized for their detergent properties and used as additives to promote drug absorption [Citation34]. According to the literature, a concentration of fatty acids in the range of 0.1–15 mM corresponds to the physiological concentration at the fasted state [Citation35,Citation36]. The concentration of fatty acids at fed state varies depending on the daily fat intake. In this study 25 mM decanoate was used to simulate the fed state [Citation35]. Due to their amphiphilic nature fatty acids are surface active and can adhere to oil/water interface of lipid formulations adapting a perpendicular orientation [Citation37–39]. To evaluate the effect of a medium-chain fatty acid on SEDDS zeta potential, size and polydispersity, SEDDS were incubated in media containing increasing concentrations of decanoate. As illustrated in () zeta potential of all ni- and c-SEDDS decreased with increasing concentrations of decanoate. Zeta potential of F1, F2 and F5 became significantly more negative already at a decanoate concentration of 0.5 mM (p < 0.05) in comparison to SEDDS that were emulsified just in water, whereas F3 and F4 exhibited a shift in zeta potential to more negative values only when emulsified in 15 mM of decanoate (p < 0.001). Due to the addition of 15 mM decanoate, the average zeta potential of ni-SEDDS decreased from −5 ± 2 mV to −18 ± 4 mV. In case of c-SEDDS, 0.1 mM Dec was sufficient to decrease significantly the magnitude of zeta potential of all formulations (p < 0.05). At decanoate concentration of 0.5 mM, most of c-SEDDS exhibited still a positive zeta potential, whereas at concentrations equal and above 5 mM all c-SEDDS became anionic. At 25 mM a further decrease in zeta potential of both ni-SEDDS and c-SEDDS was not observed. Zeta potential of the formulations remained constant over 4 h. Size and polydispersity of ni-SEDDS in presence of decanoate are reported in and Citation3(C) (). Size and polydispersity of F2, F3 and F4 were not influenced by decanoate, whereas size and polydispersity of F1 and F5 were affected by decanoate. Size of F1 decreased significantly already at decanoate concentrations ≥ 0.5 mM (p < 0.05). The most pronounced change in size was observed when F1 was emulsified in decanoate concentrations ≥ 15 mM, with a decrease from 225 ± 10 nm to 150 ± 4 nm at 15 mM decanoate. Under these conditions polydispersity of F1 was also lowered with a reduction in PDI from 0.40 ± 0.07 to 0.34 ± 0.05 at 15 mM decanoate. Size distribution of F5 underwent similar changes observed in presence of bile salts. Size of F5 started to decrease significantly from decanoate concentration of 0.1 mM. At this concentration, F5 displayed a still monomodal size distribution. As illustrated in at a concentration of 5 mM decanoate, size distribution of F5 started to become bimodal with two distinct populations with diameter of 21 nm (3% intensity) and 140 nm (10% intensity) and a PDI of 0.48 ± 0.03. At a decanoate concentration of 15 mM size distribution of F5 showed two populations with a diameter of 15 nm (11% intensity) and 140 nm (6% intensity) and a PDI of 0.5 ± 0.05. At 25 mM decanoate the size distribution remained bimodal with a PDI of 0.8 ± 0.04. Size of c-SEDDS was not affected by decanoate (), whereas PDI of most SEDDS significantly decreased when emulsified in decanoate concentrations ≥ 15 mM (). As observed in presence of bile salts, SEDDS characterized by high polydispersity (PDI > 0.3) were the most subjected to size and PDI reduction. Thus, it appears that bile salts and decanoate improved the overall size distribution of polydispersed formulations. Generally, a narrow size distribution is preferred over a broad distribution because oily droplets of different size could permeate the mucus and be taken up from the intestinal epithelium differently causing an unequal absorption of the formulation. Compared to bile salts, decanoate had a more limited effect on SEDDS. This might be due to the superior emulsifying properties of bile salts, which play a fundamental role in the emulsification of fat in the intestine.
Transmittance
Transmittance provides information on emulsion appearance. It is used as index of formulation turbidity and correlates with formulation size, distribution and concentration. The smaller size and polydispersity are, the higher the percentage of transmittance becomes [Citation40,Citation41]. Results of transmittance measurements of ni- and c-SEDDS in bile salts are reported in (), respectively. Ni-SEDDS F1 and F5 showed a significant increase in transmittance when emulsified in bile salts indicating a progressive reduction of size and polydispersity of the oily droplets. The increase in transmittance was observed in both cases starting from a bile salts concentration of 1.5 mM (p < 0.001). F2 showed only a minor increase in transmittance whereas transmittance of F3 and F4 remained constant. Among c-SEDDS only F6 and F9 showed an increase in transmittance. Formulations F6 and F9 displayed an increased transmittance starting from bile salts concentration of 1.5 mM. Ni- and c-SEDDS emulsified in decanoate displayed a behavior similar as in bile salts ().
Figure 5. (A–D): Transmittance % of nonionic (A) and cationic (B) SEDDS (0.5% m/v) emulsified in water containing indicated concentrations of bile salts; Transmittance % of nonionic (C) and cationic (D) SEDDS (0.5% m/v) emulsified in water containing indicated concentrations of sodium decanoate. Indicated values are means ± SD (n = 3). (Two way ANOVA vs water, * p < 0.05, ** p < 0.01, *** p < 0.001).
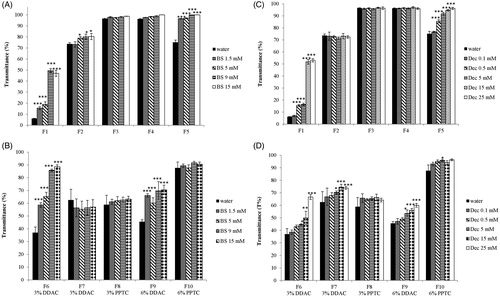
Cloud point temperature determination
Nonionic surfactants such as Tween and Cremophor EL are hydrophilic at room temperature and become more hydrophobic at higher temperatures. This behavior has been ascribed to dehydration of the hydrophilic groups at higher temperatures. The temperature at which this phenomenon occurs is called cloud point [Citation42]. At this temperature micellar solutions of nonionic surfactants become cloudy due to micellar aggregation. The cloud point of surfactants composing a o/w microemulsion affects the cloud point temperature (CPT) of the microemulsion [Citation23]. On the contrary, ionic surfactants such as DODAB and PPTC became more hydrophilic at higher temperatures due to increased degree of dissociation of the counter ion [Citation43,Citation44]. The incorporation of cationic surfactants in o/w microemulsions resulted therefore in an increased cloud point temperature (CPT). As shown in ,C) (), CPT of ni-SEDDS increased with the increase in the concentration of bile salts or decanoate. The results confirm the incorporation of bile salts and decanoate in SEDDS during the emulsification process. As ni-SEDDS F2 displayed a CPT above 100 °C the impact of bile salts and decanoate on CPT could not be determined. C-SEDDS were also characterized by CPT above 100 °C. Due to the addition of bile salts and decanoate, CPT decreased below 100 °C (). This can be explained by a reciprocal neutralization effect between the cationic surfactants and the anionic bile salts and decanoate, which is favored by dehydration of the polar heads at higher temperatures with consequential decrease of their hydrophilicity and therefore cloud point [Citation45]. The most pronounced decrease in CPT was where zeta potential of cationic formulations was negligible. In contrast, at a higher bile salts and decanoate concentration of 15 mM, SEDDS exhibited already a pronounced negative zeta potential due to prevalence of anionic surfactants on the surface of SEDDS causing a higher CPT. These results further confirm that bile salts and decanoate strongly interact with SEDDS droplets.
Figure 6. (A–D) Cloud point temperature (CPT) of nonionic (A) and cationic (B) SEDDS (0.5% m/v) emulsified in water containing indicated concentrations of bile salts; (CPT) of nonionic (C) and cationic (D) SEDDS (0.5% m/v) emulsified in water containing indicated concentrations of sodium decanoate. Indicated values are means ± SD (n = 3). (Two way ANOVA vs water, * p < 0.05, ** p < 0.01, *** p < 0.001. In case of formulations having a cloud point temperature above 100 °C, (CPT) was not experimentally determined, therefore statistical analysis was performed assuming a (CPT) equal to 100 °C).
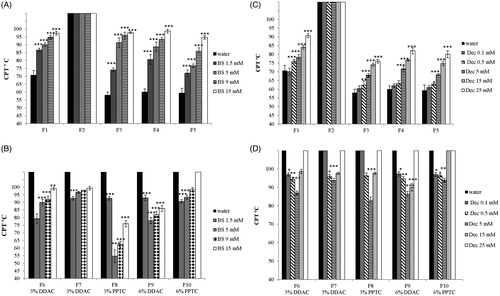
Release study
Quinine was chosen as model drug to study the impact of bile salts and decanoate on drug release. Bile salts and decanoate were tested at fed concentrations to evaluate their effect on drug release. As depicted in , after 15 min the quinine release from ni-SEDDS F3, F4 and F5 and c-SEDDS F6 and F8 was significantly accelerated by the presence of bile salts and decanoate in the medium (p < 0.05). All formulations followed the same trend even though the increase was not significant. Over time, the difference in the drug release behavior due to the presence of BS and decanoate became less pronounced. At 240 min, only the quinine release from ni-SEDDS F3 and F5 was significantly affected by bile salts and decanoate. Generally, ni-SEDDS were more affected by bile salts and decanoate than c-SEDDS. No correlation could be established between the observed size shrinking, characterizing formulations F1, F5 and F6 ()), and the increase in release rate in the presence of bile salts and decanoate.
Impact of the findings
Within this study it was shown that bile salts and decanoate have a substantial effect on properties of SEDDS such as zeta potential, size, PDI, transmittance, cloud point temperature (CPT) and drug release. The extent of the observed impact on SEDDS physical properties and drug release was mostly formulation dependent. SEDDS are complex systems and it is likely that in particular substructures of surfactants on the surface of SEDDS have a substantial impact on interactions with bile salts and medium chain fatty acids in vivo. These interactions effect size, zeta potential, PDI and drug release from SEDDS. The decrease in zeta potential, for instance, is likely attributed to the integration of bile salts and decanoate in the surface structure of the oily droplets formed by SEDDS. Similar phenomena were observed when lipid nanoparticles and emulsions were incubated with media containing bile salts. Ball et al., for instance, observed a decrease in zeta potential and size of lipid nanoparticles containing siRNA, which was attributed to the surfactant-like properties of bile salts. When lipid nanoparticles loaded with siRNA were incubated with 15 mM bile salts a complete loss of siRNA gene silencing properties was observed indicating the substantial impact of bile salts on the performance of this drug delivery system [Citation17]. Bile salts have even been used as excipients for the preparation of vesicles to stabilize them against endogenous bile salts of GI fluids [Citation46]. Indeed, bile salts have a solubilizing effect on liposomes favoring the formation of mixed micelles causing a faster drug release [Citation47]. When incorporated in liposomes bile salts act as stabilizer by repulsing bile salts of GI fluids. The effect of bile salts on ni- and c-SEDDS was not destabilizing. Size and PDI profiles remained unchanged or were improved and zeta potential became negative. This could promote SEDDS diffusion through the intestinal mucus gel layer as it was demonstrated that SEDDS characterized by negative zeta potential and small size are most favored in terms of mucus permeation [Citation11]. Moreover, only droplets exhibiting a size up to 100 nm seem to passively permeate the cell membrane [Citation48]. Furthermore, bile salts and medium chain fatty acids are recognized for their permeation enhancing properties [Citation34,Citation49,Citation50].
In the last years, zeta potential changing SEDDS (zpc-SEDDS) have been developed with the aim to promote both permeation through the mucus and cellular uptake of SEDDS. Zpc-SEDDS are loaded with phosphorylated ligands bearing amino groups and possess an overall negative charge in the intestinal lumen facilitating their mucus diffusion. Once Zpc-SEDDS reach the epithelium the phosphate groups are cleaved by intestinal alkaline phosphatase (AP) with a shift in zeta potential toward positive values, that reduces back diffusion of the formulations and promotes their uptake [Citation51]. In vivo data provided evidence of the efficacy of zeta potential changing systems in improving oral bioavailability of peptides [Citation52]. Even though these systems showed promising results in vitro and in vivo, to further improve their performance in vivo, their behavior in media containing bile salts and fatty acids should be investigated.
Conclusion
Within this study it was demonstrated that bile salts and medium chain fatty acids such as decanoate have a substantial impact on the properties of SEDDS depending on their composition. Both bile salts and decanoate caused a decrease in zeta potential of ni- and c-SEDDS, which became highly negative when concentrations of bile salts or decanoate in the range of fasted and fed state were tested. Ni- and c-SEDDS displayed a decrease in size and PDI in presence of bile salts and decanoate. Bile salts and decanoate did not affect SEDDS stability. Bile salts and decanoate incorporation in SEDDS was further confirmed by changes in cloud point temperature of the formulations. Moreover, a formulation dependent increase in the release of quinine in presence of bile salts and decanoate was observed. The results underline the importance of formulating SEDDS taking the effects of bile salts and fatty acids into account.
Disclosure statement
No potential conflict of interest was reported by the author(s).
References
- Pouton CW , Porter CJH. Formulation of lipid-based delivery systems for oral administration: materials, methods and strategies. Adv Drug Deliv Rev. 2008;60(6):625–637.
- Williams HD , Ford L , Igonin A , et al. Unlocking the full potential of lipid-based formulations using lipophilic salt/ionic liquid forms. Adv Drug Deliv Rev. 2019;142:75–90.
- Zupančič O , Partenhauser A , Lam HT , et al. Bernkop-Schnürch, Development and in vitro characterisation of an oral self-emulsifying delivery system for daptomycin. Eur J Pharm Sci. 2016;562:180–186.
- Bonengel S , Jelkmann M , Abdulkarim M , et al. Impact of different hydrophobic ion pairs of octreotide on its oral bioavailability in pigs. J Control Release. 2018;273:21–29.
- Zupančič O , Grieβinger JA , Rohrer J , et al. Development, in vitro and in vivo evaluation of a self-emulsifying drug delivery system (SEDDS) for oral enoxaparin administration. Eur J Pharm Biopharm. 2016;109:113–121.
- Hintzen F , Perera G , Hauptstein S , et al. In vivo evaluation of an oral self-microemulsifying drug delivery system (SMEDDS) for leuprorelin. Int J Pharm. 2014;472(1-2):20–26.
- Menzel C , Holzeisen T , Laffleur F , et al. In vivo evaluation of an oral self-emulsifying drug delivery system (SEDDS) for exenatide. J Control Release. 2018;277:165–172.
- Zhang J , Li J , Ju Y , et al. Mechanism of enhanced oral absorption of Morin by phospholipid complex based self-nanoemulsifying drug delivery system. Mol Pharm. 2015;12(2):504–513.
- Zaichik S , Steinbring C , Menzel C , et al. Development of self-emulsifying drug delivery systems (SEDDS) for ciprofloxacin with improved mucus permeating properties. Int J Pharm. 2018;547(1-2):282–290.
- Hetényi G , Griesser J , Moser M , et al. Comparison of the protective effect of self-emulsifying peptide drug delivery systems towards intestinal proteases and glutathione. Int J Pharm. 2017.
- Griesser J , Hetényi G , Kadas H , et al. Self-emulsifying peptide drug delivery systems: How to make them highly mucus permeating. Int J Pharm. 2018;538(1-2):159–166.
- Parthasarathi S , Muthukumar SP , Anandharamakrishnan C. The influence of droplet size on the stability, in vivo digestion, and oral bioavailability of vitamin E emulsions. Food Funct. 2016;7(5):2294–2302.
- Michaelsen MH , Wasan KM , Sivak O , et al. The effect of digestion and drug load on halofantrine absorption from self-nanoemulsifying drug delivery system (SNEDDS). Aaps J. 2016;18(1):180–186.
- Leonaviciute G , Zupančič O , Prüfert F , et al. Impact of lipases on the protective effect of SEDDS for incorporated peptide drugs towards intestinal peptidases. Int J Pharm. 2016;508(1-2):102–108.
- He H , Lu Y , Qi J , et al. Adapting liposomes for oral drug delivery. Acta Pharm Sin B. 2019.
- Jódar-Reyes AB , Torcello-Gómez A , Wulff-Pérez M , et al. Different stability regimes of oil-in-water emulsions in the presence of bile salts. Food Res Int. 2010;43:1634–1641.
- Ball RL , Bajaj P , Whitehead KA. Oral delivery of siRNA lipid nanoparticles: fate in the GI tract. Sci Rep. 2018;8. DOI:10.1038/s41598-018-20632-6
- Maldonado-Valderrama J , Wilde P , MacIerzanka A , et al. The role of bile salts in digestion. Adv Colloid Interface Sci. 2011;165(1):36–46.
- Zhang Z , Gao F , Jiang S , et al. Bile salts enhance the intestinal absorption of lipophilic drug loaded lipid nanocarriers: mechanism and effect in rats. Int J Pharm. 2013;452(1-2):374–381.
- Matsuoka K , Moroi Y. Micelle formation of sodium deoxycholate and sodium ursodeoxycholate (Part 1). Biochim Biophys Acta - Mol Cell Biol Lipids. 2002;1580(2-3):189–199.
- Lupo N , Tkadlečková VN , Jelkmann M , et al. Self-emulsifying drug delivery systems: In vivo evaluation of their potential for oral vaccination. Acta Biomater. 2019;94:425–434.
- Leichner C , Menzel C , Laffleur F , et al. Development and in vitro characterization of a papain loaded mucolytic self-emulsifying drug delivery system (SEDDS). Int J Pharm. 2017;530(1-2):346–353.
- AboulFotouh K , Allam AA , El-Badry M. Self-emulsifying drug delivery systems: easy to prepare multifunctional vectors for efficient oral delivery. In: Current and Future Aspects of Nanomedicine. IntechOpen;. 2020. http://dx.doi.org/10.5772/intechopen.88412
- Denninger A , Westedt U , Rosenberg J , et al. A rational design of a biphasic dissolution setup—modelling of biorelevant kinetics for a ritonavir hot-melt extruded amorphous solid dispersion. Pharmaceutics. 2020;12(3):237.
- Pouton CW. Lipid formulations for oral administration of drugs: Non-emulsifying, self-emulsifying and “self-microemulsifying” drug delivery systems. Eur J Pharm Sci. 2000;11:S93–S98.
- P, Marteau M, Minekus R, Havenaar JHJ. Huis In’t Veld, survival of lactic acid bacteria in a dynamic model of the stomach and small intestine: validation and the effects of bile. J Dairy Sci. 1997.
- Wickham M , Garrood M , Leney J , et al. Modification of a phospholipid stabilized emulsion interface by bile salt: effect on pancreatic lipase activity. J Lipid Res. 1998;39(3):623–632.
- Beysseriat M , Decker EA , McClements DJ. Preliminary study of the influence of dietary fiber on the properties of oil-in-water emulsions passing through an in vitro human digestion model. Food Hydrocoll. 2006;20:800–809.
- Mun S , Decker EA , McClements DJ. Influence of emulsifier type on in vitro digestibility of lipid droplets by pancreatic lipase. Food Res Int. 2007;40(6):770–781.
- Tiss A , Ransac S , Lengsfeld H , et al. Surface behaviour of bile salts and tetrahydrolipstatin at air/water and oil/water interfaces. Chem Phys Lipids. 2001;111(1):73–85.
- Euston SR , Bellstedt U , Schillbach K , et al. The adsorption and competitive adsorption of bile salts and whey protein at the oil-water interface. Soft Matter. 2011;7(19):8942.
- Vadnere M , Lindenbaum S. Distribution of bile salts between 1-octanol and aqueous buffer . J Pharm Sci. 1982;71(8):875–881.
- Poša M , Ćirin D , Krstonošić V. Physico-chemical properties of bile salt-Tween 80 mixed micelles in the viewpoint of regular solution theory. Chem Eng Sci. 2013;98:195–202.
- Maher S , Leonard TW , Jacobsen J , et al. Safety and efficacy of sodium caprate in promoting oral drug absorption: from in vitro to the clinic. Adv Drug Deliv Rev. 2009;61(15):1427–1449.
- Ainousah BE , Perrier J , Dunn C , et al. Dual level statistical investigation of equilibrium solubility in simulated fasted and fed intestinal fluid. Mol Pharm. 2017;12:4170–4180.
- Persson EM , Gustafsson AS , Carlsson AS , et al. The effects of food on the dissolution of poorly soluble drugs in human and in model small intestinal fluids. Pharm. Res. 2005;22(12):2141–2151.
- Rogerson ML , Robinson BH , Bucak S , et al. Kinetic studies of the interaction of fatty acids with phosphatidylcholine vesicles (liposomes). Colloids Surf B Biointerfaces. 2006;48(1):24–34.
- Muhamad R , Misran M. Adsorption kinetics of partially ionized fatty acids at oil/water interface of their monomeric and liposomal solution. Colloids Surfaces A Physicochem. Eng. Asp. 2017;528:23–29. https://doi.org/10.1016/j.colsurfa.2017.04.040.
- Beaman DK , Robertson EJ , Richmond GL. From head to tail: structure, solvation, and hydrogen bonding of carboxylate surfactants at the organic-water interface. J Phys Chem C. 2011;115(25):12508–12516.
- Ding Z , Jiang Y , Liu X. Chapter 12 - Nanoemulsions-based drug delivery for brain tumors. In: Kesharwani P , Gupta BT , editors. Nanotechnology-based targeted drug delivery systems for brain tumors. Elsevier; 2018. p. 327–358. https://doi.org/10.1016/B978-0-12-812218-1.00012-9.
- Polychniatou V , Tzia C. Study of formulation and stability of co-surfactant free water-in-olive oil nano- and submicron emulsions with food grade non-ionic surfactants, JAOCS. J Am Oil Chem Soc. 2014;91(1):79–88.
- Al-Sabagh AM , Nasser NM , Migahed MA , et al. Effect of chemical structure on the cloud point of some new non-ionic surfactants based on bisphenol in relation to their surface active properties. Egypt. J. Pet. 2011;20(2):59–66.
- Schott H , Han SK. Effect of symmetrical tetraalkylammonium salts on cloud point on nonionic surfactants. J Pharm Sci. 1977;66(2):165–168. https://doi.org/https://doi.org/10.1002/jps.2600660208.
- Walsh D. Microemulsions. Background, new concepts, applications, perspectives. Edited by Cosima Stubenrauch. Angew Chem Int Ed. 2009;48(25):4474–4474.
- Kumar S , Sharma D , Khan ZA. Kabir-ud-Din, Salt-induced cloud point in anionic surfactant solutions: Role of the headgroup and additives. Langmuir. 2002;18:4205–4209.
- Aburahma MH. Bile salts-containing vesicles: promising pharmaceutical carriers for oral delivery of poorly water-soluble drugs and peptide/protein-based therapeutics or vaccines. Drug Deliv. 2016;23(6):1847–1867.
- Hu S , Niu M , Hu F , et al. Integrity and stability of oral liposomes containing bile salts studied in simulated and ex vivo gastrointestinal media. Int J Pharm. 2013;441(1-2):693–700.
- Bunchongprasert K , Shao J. Cytotoxicity and permeability enhancement of Capmul®MCM in nanoemulsion formulation. Int J Pharm. 2019;561:289–295.
- Krug SM , Amasheh M , Dittmann I , et al. Sodium caprate as an enhancer of macromolecule permeation across tricellular tight junctions of intestinal cells. Biomaterials. 2013;34(1):275–282.
- Stojančević M , Pavlović N , Goločorbin-Kon S , et al. Application of bile acids in drug formulation and delivery. Front Life Sci. 2013;7(3–4):112–122.
- Griesser J , Hetényi G , Federer C , et al. Highly mucus permeating and zeta potential changing self-emulsifying drug delivery systems: a potent gene delivery model for causal treatment of cystic fibrosis. Int J Pharm. 2019;557:124–134.
- Wu J , Zheng Y , Liu M , et al. Biomimetic viruslike and charge reversible nanoparticles to sequentially overcome mucus and epithelial barriers for oral insulin delivery. ACS Appl Mater Interfaces. 2018;10(12):9916–9928.
Appendix
Table A1. Zeta potential (mV) of nonionic (ni-SEDDS) and cationic (c-SEDDS) SEDDS (0.5% m/v) emulsified in water containing indicated concentrations of bile salts or decanoate; Indicated values are means ± SD (n = 3).
Table A2. Size (nm) of nonionic (ni-SEDDS) and cationic (c-SEDDS) SEDDS (0.5% m/v) emulsified in water containing indicated concentrations of bile salts or decanoate; Indicated values are means ± SD (n = 3). Formulations not significantly affected by bile salts or decanoate are not reported in the table.
Table A3. PDI of nonionic (ni-SEDDS) and cationic (c-SEDDS) SEDDS (0.5% m/v) emulsified in water containing indicated concentrations of bile salts or decanoate; Indicated values are means ± SD (n = 3). Formulations not significantly affected by bile salts or decanoate are not reported in the table.
Table A4. Transmittance (%) of nonionic (ni-SEDDS) and cationic (c-SEDDS) SEDDS (0.5% m/v) emulsified in water containing indicated concentrations of bile salts or decanoate; Indicated values are means ± SD (n = 3). Formulations not significantly affected by bile salts or decanoate are not reported in the table.
Table A5. Cloud point temperature (°C) of nonionic (ni-SEDDS) and cationic (c-SEDDS) SEDDS (0.5% m/v) emulsified in water containing indicated concentrations of bile salts or decanoate; Indicated values are means ± SD (n = 3). Formulations not significantly affected by bile salts or decanoate are not reported in the table.